Abstract
SUS316L stainless steel and cobalt–chromium and platinum–chromium alloys are widely used platforms for coronary stents. These alloys also contain nickel (Ni), which reportedly induces allergic reactions in some subjects and is known to have various cellular effects. The effects of Ni on neointima formation after stent implantation remain unknown, however. We developed coronary stents made of Ni-free high-nitrogen austenitic stainless steel prepared using a N2-gas pressurized electroslag remelting (P-ESR) process. Neointima formation and inflammatory responses following stent implantation in porcine coronary arteries were then compared between the Ni-free and SUS316L stainless steel stents. We found significantly less neointima formation and inflammation in arteries implanted with Ni-free stents, as compared to SUS316L stents. Notably, Ni2+ was eluted into the medium from SUS316L but not from Ni-free stainless steel. Mechanistically, Ni2+ increased levels of hypoxia inducible factor protein-1α (HIF-1α) and its target genes in cultured smooth muscle cells. HIF-1α and their target gene levels were also increased in the vascular wall at SUS316L stent sites but not at Ni-free stent sites. The Ni-free stainless steel coronary stent reduces neointima formation, in part by avoiding activation of inflammatory processes via the Ni-HIF pathway. The Ni-free-stainless steel stent is a promising new coronary stent platform.
1. Introduction
In-stent restenosis following coronary stent implantation remains an important problem following percutaneous coronary intervention (PCI) [Citation1, Citation2]. Despite intensive efforts to improve the design and metal composition of stents, the rates of in-stent restenosis induced by bare metal stents range from 22 to 49% [Citation2–Citation5]. Drug-eluting stent technologies have markedly reduced in-stent restenosis rates to 0–31% [Citation5–Citation10], but drug eluting stents cause a new problem, namely late thrombosis [Citation11], though a recent meta-analysis reported that a cobalt–chromium (Co–Cr) everolimus-eluting stent had a lower rate of stent thrombosis than bare metal stents during the 2 years after implantation [Citation12]. To suppress stent thrombosis, the current American Heart Association/American College of Cardiology guidelines recommend dual anti-platelet therapy (DAPT), entailing the use of clopidogrel plus aspirin, for at least 12 months after drug-eluting stent implantation and for a minimum of 1 month after implantation of a bare metal stent [Citation13, Citation14]. However, DAPT increases the risk of major bleeding [Citation15, Citation16] and poses a challenge if non-cardiac surgery is necessary: while cessation of anti-platelet therapy increases the risk for stent thrombosis, its continuation increases the risk of surgical bleeding [Citation17]. Furthermore, a recent meta-analysis showed that while implantation of drug-eluting stents after ST-segment elevation myocardial infarction reduced the need for target-vessel revascularization, as compared to bare metal stents, the drug-eluting stents were associated with increased risks of very late stent thrombosis and reinfarction. Mechanisms proposed to cause this late stent thrombosis include delayed endothelialization and vascular healing, late acquired stent malapposition and hypersensitivity reactions [Citation18, Citation19]. Therefore, it would be highly desirable to develop coronary stents that have better profiles with respect to the pathological processes involved in in-stent restenosis and vascular healing.
At present, SUS316L, Co–Cr and platinum–chromium (Pr–Cr) are the most widely used coronary stent platforms [Citation9, Citation10]. Ni is added to these metal alloys to increase their flexibility [Citation10, Citation20]. However, Ni is known to induce biological responses in animals. For instance, Ni allergy is a major cause of contact hypersensitivity and affects millions of people worldwide [Citation21, Citation22]. Ni allergy is also reportedly associated with higher risks of restenosis after PCI [Citation23, Citation24], though conflicting data have been reported [Citation25]. Ni2+ solubilized in sweat and other body fluids serves as a sensitizing allergen in Ni-induced contact hypersensitivity, and Ni2+ has been shown to directly activate inflammatory processes. For instance, Ni2+ triggers rapid expression of surface adhesion molecules such as vascular cell adhesion molecule 1 (VCAM-1) and intercellular adhesion molecule 1 (ICAM-1) on endothelial cells, as well as chemokines such as MCP-1 [Citation26, Citation27]. Mechanistically, it has been proposed that the hypersensitivity depends on activation of Toll-like receptor 4 and hypoxia inducible factor (HIF) signaling, and downregulation of the anti-apoptotic factor cellular Fas associated death domain-like interleukin 1 beta-converting enzyme-like inhibitory protein (cFLIP) [Citation21].
Given the extensive medical application of stainless steel, its susceptibility to corrosion (metal ion release) and fatigue are important factors. Generally, elemental Ni is added to stainless steel to mitigate its negative characteristics. However, Ni2+ released from the amorphous surface layer of stainless steel reportedly causes allergic reactions and inflammation. For that reason, the idea of substituting N2 for the Ni has been considered. However, homogenous injection of N2 into stainless steel is not feasible using classical methods such as N2 adsorption [Citation28]. We therefore developed Ni-free austenitic stainless steel with a high N2 content fabricated using an electroslag remelting (P-ESR) method under a pressurized N2 gas atmosphere [Citation29]. This P-ESR method enables us to inject N2 homogenously into stainless steel, yielding Ni-free stainless steel that is highly resistant to corrosion [Citation29, Citation30] and fatigue [Citation31].
In this study, we produced a Ni-free stainless steel coronary artery stent and evaluated its utility in a porcine coronary model. This is one of the first reports examining the feasibility of using Ni-free stainless steel for treating heart disease.
The material of coronary stents must be strong to withstand the forces produced by the beating heart and also biocompatible, because local inflammation of the coronary artery wall can lead to in-stent restenosis [Citation32]. Indeed, Ni allergy is thought to be one cause of in-stent restenosis [Citation23]. We therefore speculate that a Ni-free stainless steel stent fabricated using our new method would have advantages over conventional Ni-containing stents.
In this study, we found that arteries implanted with the Ni-free stent showed significantly less neointima formation than arteries implanted with other bare metal stents. In addition, HIF activation by Ni2+ eluted from stent struts was a key contributor to neointima formation in arteries implanted with SUS316L stents, but was avoided in arteries implanted with Ni-free stents.
2. Materials and methods
2.1. Animals
All experimental studies were approved by the University of Tokyo Ethics Committee for Animal Experiments and strictly adhered to the guidelines for animal experiments of the University of Tokyo.
2.2. Ni-free high-nitrogen stainless steel (HNS) stent
Ni-free HNS ingot was first prepared using a N2-gas P-ESR process [Citation29–Citation31]. Melting was conducted under 4 MPa of N2 gas pressure. FeCrN powder was used as the nitrogen source. Because nitrogen is an austenite-forming element, Ni-free austenitic stainless steel was successfully fabricated by adding soluble nitrogen up to more than 1 wt%. SUS316L stainless steel stents served as controls. The Ni contents of the Ni-free stainless steel and SUS316L were less than 0.005 and 12 wt%, respectively. To produce a seamless pipe 1.4 mm in diameter and 0.1 mm in thickness, a seamless pipe was machined first, followed by drawing with frequent annealing treatment. Stents were then formed through laser machining followed by surface smoothing using an electropolishing method.
2.3. Stent implantation into porcine coronary artery
Ten pigs were implanted with stents via femoral access according to the standard procedure, using balloon pressure set to expand the arterial diameter by a ratio of 1.2:1 [Citation33]. The extent of the dilation of the stenting region was immediately assessed using intravascular ultrasound Eagles Eye Gold Catheter, (Volcano Corporation, California, USA), and expansion ratios were determined to be 1.20 ± 0.06 (SD). Each pig received one or two 3 × 12 mm stents in each of two out of three main coronary arteries (left anterior descending artery and left circumflex artery). A total of 20 coronary arteries received the control SUS316L stainless stents and 17 received Ni-free stainless stents. Coronary artery angiography and intravascular ultrasound imaging were performed 28 days after the stent implantation.
After coronary angiography, hearts were explanted from the thoracic cavity and flushed using pressure perfusion with saline. The arterial portions that received stents were removed and fixed in 10% buffered formalin, embedded in glycol methacrylate (Sakura Finetek Japan, Tokyo, Japan), and duplicate samples were cut into 5-μm-thick sections using published procedures [Citation33]. The sections were cut at three positions: a proximal section, 2 mm distal from the proximal end of stent; a middle section, 6 mm distal from the proximal end; and a distal section, 2 mm proximal from the distal end. Of these, three from each group were subsequently examined histologically. Sections were stained with hematoxylin/eosin or Masson's trichrome, or processed for immunohistochemistry.
2.4. Cell culture
Rat aortic smooth muscle cells (SMCs) were isolated as previously described [Citation34] and cultured in Dulbecco's modified Eagle medium/Nutrient F-12 (Ham's, DMEM/F12, Life Technologies Corporation, California, USA) supplemented with 10% fetal bovine serum (FBS, Thermo Scientific; Hyclone Laboratory, Massachusetts, USA), except where otherwise indicated. Cells from two separate isolates were used between passages 8 and 12.
2.5. Quantitative reverse transcriptase-PCR
Total RNA was purified from cultured cells using RNeasy (Qiagen, Hilden, Germany) and from in vivo samples using an Isogen PB kit (Nippon Gene, Tokyo, Japan) [Citation35]. For quantitation of the transcripts, real-time polymerase chain reaction (PCR) was carried out using a LightCycler (Roche, Basel, Switzerland) and a QuantiTect SYBR green PCR kit (Qiagen, Hilden, Germany). The expression level of each gene was normalized to that of 18s rRNA, which served as an endogenous internal control. The sequences of the primers used were as follows: rat Vegfa, 5′-AAA AAC GAA AGC GCA AGA AA-3′ and 5′-TTT CTC CGC TCT GAA CAA GG-3′; rat Adm1, 5′-ACA GTC CCG ACC CAG ACT C-3′ and 5′-AAT GTG GGC TGT GCT CTG A-3′; rat Adm2, 5′-GTC TGG CAG CCT GGG TAA G-3′ and 5′-CGC TGG AAG GAA TCT TGG-3′; rat Pdgfb, 5′-CCG CTC CTT TGA TGA CCT T-3′ and 5′-CTC AGC CCC ATC TTC GTC T-3′; porcineVegfa, 5′-AGC TAC TGC CGT CCA ATC GAG-3′ and 5′-TGT CAC ATC TGC AAG TAC GTT CG-3′; porcine Pdgfb, 5′-CGC ACC AAC GCC AAC TTC C-3′ and 5′-TTT GGC TCG CTG CTC CTG GG-3′; porcine Tnf, 5′-TGG CCC CTT GAG CAT CAA-3′ and 5′-CGA CGG GCT TAT CTG AGG T-3′.
2.6. Immunohistochemistry
For immunohistochemistry, a rabbit polyclonal antibody against HIF-1α (Novus Biologicals, Colorado, USA) served as the primary antibody, and staining was accomplished using catalyzed signal amplification (Dako Cytomation Japan, Tokyo, Japan). The HIF1α signal intensity was enhanced using a heat-induced antigen retrieval method with citrate buffer (pH 6.0). To detect SMα-actin, we used a fluorescein isothiocyanate (FITC)-conjugated mouse monoclonal antibody: clone 1A4 (Sigma Aldrich, Missouri, USA).
2.7. Measurement of Ni2+ ion release
Sample solutions were prepared by immersing five mirror-polished metal discs (10 mm in diameter, 1 mm in thickness) in 2.5 ml of saline for 7 days at 37 °C. Release of Ni2+ from metals was analyzed using a colorimetric method with dimethylglyoxime (DMG), as previously described [Citation36] with minor modifications. To determine the Ni2+ concentration, 500 μl of saturated citric acid solution was added to 500 μl of sample solution, after which 500 μl of 0.03 mol l–1 DMG solution was added. After incubation for 10 min, the absorbance at 540 nm was measured spectrophotometrically using a microplate reader.
2.8. Statistical analysis
Mean values were compared between two groups using Student's t-test for unpaired values. To compare more than two groups, analysis of variance (ANOVA) was carried out, followed by Tukey–Kramer's post hoc test for multiple comparisons to identify differences among specific groups. Values of P < 0.05 were considered significant.
3. Results
3.1. Visibility of Ni-free stainless stents on a clinical x-ray setup
Ni has little radiolucency, which enhances the visibility of SUS316L stainless stents on coronary angiography. Exclusion of Ni from stainless steel could therefore reduce the visibility of the stents. To test this possibility, we assessed the visibility of Ni-free stents within explanted porcine coronary arteries in a cardiac angiography system. Although the visibility of the Ni-free stents was slightly inferior to that of the SUS316L stents, Ni-free stent struts were clearly visible (figure (a)). Moreover, the Ni-free stents were also clearly visible during their implantation in porcine coronary arteries. The maneuverability of the Ni-free stents did not differ from that of SUS316L stents of the same design.
Figure 1 Neointima formation following coronary stenting is reduced with Ni-free stainless steel stents. (a) Representative x-ray photographs of SUS316L stainless and Ni-free stainless stents within isolated porcine coronary arteries 28 days after implantation. (b)–(g) Coronary stents made of SUS316L or Ni-free stainless steel were implanted in the left anterior descending and circumflex arteries of pigs. The stented regions were then analyzed using radiography and intravascular ultrasound imaging, after which the stented arteries were harvested. In (b), representative angiographic views of the porcine left circumflex arteries 28 days after stent implantation are shown. All angulation was approximately right anterior oblique 50. Arrowheads indicate the ends of the stent-implanted sites in the left circumflex arteries. (c) Per cent in-stent restenosis evaluated using angiography; n = 20 and 17 in the SUS315L and Ni-free stent groups, respectively. ∗P < 0.05. (d) Representative sections of stented arteries stained with hematoxylin/eosin. L: lumen, N: neointima, M: media, bars: 500 μm. For morphometric analysis, luminal areas were defined as the blank areas within arteries. The smooth muscle layer, including the neointima and media, was defined as the inner side (high cell density) area of the vascular wall, while the adventitia was defined as the outer side (low cell density) area. The borders of the neointima (inner side of the smooth muscle layer) and media (outer side of the smooth muscle layer) were defined as the boundaries between two different cell alignments. In addition, in-stent neointima formation was evaluated at three positions within each stent (proximal, middle or distal), and the data from the position that showed the largest intimal area/medial area ratio were selected as representative for that stent. Results of histological analyses of the intimal area (e), intimal area/medial area ratio (f) and lumen area (g); n = 20 and 17 in the SUS316L and Ni-free stent groups, respectively. ∗P < 0.05.
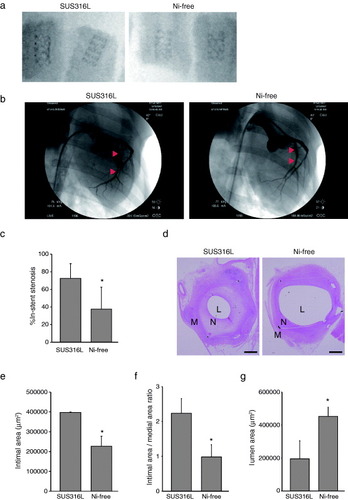
3.2. Neointima formation after coronary stent implantation was reduced with Ni-free stainless stents
Seventeen Ni-free and 20 SUS316L stents were deployed into the left anterior descending and left circumflex coronary arteries. Ballooning pressures required to gain the aimed pre-post-artery diameter ratio (1:1.2) did not significantly differ between the two groups (data not shown), which indicates the compliance of the Ni-free stents was similar to that of the SUS316L stents. Angiography showed that all arteries were patent 28 days after stent implantation (figure (b)). Ni-free stents were associated with a significantly smaller per cent in-stent stenosis (figure (c)), smaller neointimal area (figures (d) and (e)), smaller intimal area/medial area ratio (figure (f)), and a significantly larger luminal area than SUS316L stents (figure (g)). We also performed morphometric analyses at three positions within the stents (proximal, middle and distal), but we found no specific tendency for positional effects on in-stent neointima formation (supplementary S1, available from stacks.iop.org/STAM/13/064218/mmedia). All stent struts were completely covered by the endothelium and vascular SMCs in both groups (figure (d)). Thus Ni-free stents avoided neointima formation with no evidence of unfavorable healing delay (e.g. uncovered stent struts).
3.3. Vascular inflammation after stent implantation was reduced with Ni-free stainless stent
A number of basophilic mononuclear cells were observed, mainly surrounding stent struts, in the SUS316L stent-implanted arterial walls (figures (a) and (b)). By contrast, Ni-free stent-implanted arteries showed markedly fewer infiltrating cells (figures (a) and (b)). The SUS316L stent sites also showed extensive proliferation of neointimal cells, and much less neointimal cell proliferation was seen following Ni-free stent implantation (figure (a)). Moreover, the deposition of extracellular matrix, including collagen (figure (c), blue signal indicates collagen deposition), was greatly reduced in the walls of arteries implanted with Ni-free stents, as compared to SUS316L stents.
Figure 2 Reduced inflammatory cell infiltration and fibrotic changes following Ni-free stent implantation. Photomicrographs of cross-sections of porcine coronary arteries 28 days after implantation of SUS316L or Ni-free stainless stents. Hematoxylin/eosin staining (a, b) and Masson's trichrome staining (c). Ni-free stainless stent-implanted arteries showed significantly less inflammatory cell infiltration, as indicated by the smaller number of hematoxylin-positive mononuclear cells (a, b) and smaller areas of collagen deposition (blue signal), as compared to SUS316L stainless stent-implanted vessel segments (c). L: lumen, N: neointima, M: media, ∗: stent struts, scale bars: 1 mm (a, c) and 100 μm (b).
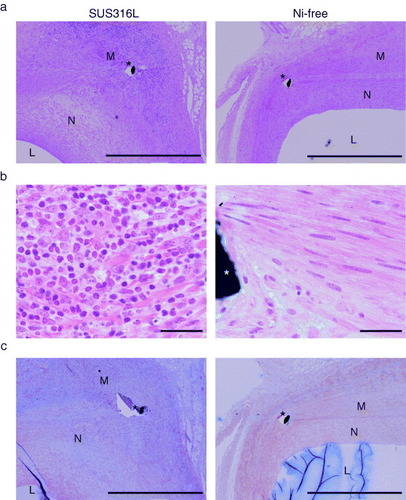
3.4. Ni2+ is released from SUS316L but not from Ni-free stainless steel
Given the clear histological differences in the responses to Ni-free and SUS316L stents, we hypothesized that dissolved Ni2+ ion might enhance neointima formation. To test this idea, we first determined whether Ni might be eluted from SUS316L stainless steel discs in saline solution. As expected, Ni2+ was indeed eluted from the SUS316L stainless, but was undetectable in the saline containing Ni-free stainless discs (figure (a)).
Figure 3 Ni2+ activates HIF-1α in vascular SMCs. (a) Dissolution of Ni2+ from SUS316L and Ni-free stainless into saline solution, ∗P < 0.05. (b) Western blots of HIF-1α in cultured vascular SMCs supplemented with Ni2+ for 6 h under normoxic conditions. Ni2+ stabilized HIF-1α in cultured vascular SMCs under normoxic conditions. (c) Hypoxia modestly induced HIF-1α target genes (Vegfa, Adm2 and Pdgfb) in cultured SMCs; n = 3 in each group, ∗P < 0.05 versus control, ∗∗P < 0.05 versus 0.5 mM Ni under the same O2 conditions. #P < 0.05 versus the same Ni concentration group in 20% O2.
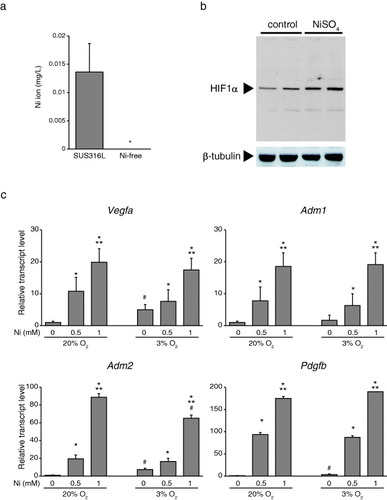
3.5. Ni2+ increases HIF-1α levels in arterial SMCs
SMCs are the major cell type forming neointimal lesions. For that reason, we next analyzed the effects of Ni2+ on SMCs. As described previously in rat pleural mesothelial cells [Citation37] and human lung epithelial cells [Citation38], Ni2+ increased the level of HIF-1α (HIF1A) protein, which is important for SMC proliferation and vascular remodeling [Citation39] under the normoxic conditions (figure (b)). In addition, expression of downstream target genes of HIF-1α, including Vegfa, Adm1, Adm2 and Pdgfb [Citation40, Citation41], was markedly upregulated by Ni2+ under normoxic conditions. As expected, hypoxia modestly increased levels of Vegfa, Adm2 and Pdgfb; however, hypoxia did not further increase the target gene expression induced by Ni2+ (figure (c)). These results suggest that Ni2+ eluted from SUS316L stainless may promote vascular remodeling by activating HIF-1 signaling in SMCs. A schematic summary of this study is shown in figure .
Figure 4 Schematic of HIF-1α target gene activation in vascular smooth muscle cells by Ni2+ from a SUS316L stent. Under normoxic conditions within smooth muscle cells, HIF1-α is post-translationally hydroxylated by PHDs (prolyl hydroxylase domain-containing enzymes), and this hydroxylation leads to pVHL (von-Hippel–Lindau protein)-dependent ubiquitination and degradation of HIF-1α in the cytoplasm. Fe2+ is required for the reaction catalyzed by PHDs. Under hypoxic conditions, the degradation process is blocked, enabling HIF1-α to enter the nucleus and induce expression of its target genes, which include Vegfa, Pdgfb, Adm1 and Adm2. Even under normoxic conditions, Ni2+ may displace the Fe2+ from its binding site on PHDs, leading to HIF1-α activation.
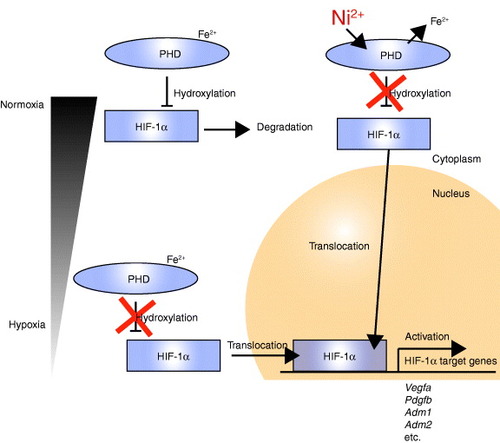
3.6. HIF-1α activation was avoided with Ni-free stents
We next investigated whether HIF-1α signaling might be activated by SUS316L stent implantation in vivo. We detected expression of HIF-1α protein in SMCs within the neointima induced by SUS316L stent placement, whereas HIF-1α was not detected in the walls of arteries receiving Ni-free stents (figure (a)). Consistent with that finding, levels of mRNA expression of the HIF-1α target genes Vegfa and Pdgfb and the inflammatory cytokine Tnf were markedly increased by implantation of SUS316 stents, but not Ni-free stents (figure (b)). Among these factors, Tnf was induced not only in vascular walls of SUS316L stainless stent implantation group, but also those of Ni-free stainless stent implantation group, compared to unimplanted vascular wall (figure (b)). During the stent implantation, we dilated the vessel wall to increase the vessel diameter up to 1.2 times. Mechanical stress might also activate many stress response pathways. Ni-free stent might block Ni2+-mediated injury including HIF1-α pathway activation. However, mechanical stretch mediated injuries are of course not avoided and might result in weak induction of Tnf in the Ni-free stainless stent implanted group (figure (b)). These results suggest that Ni2+ eluted from SUS316L stents activates HIF-1α within arterial walls, which in turn activates inflammatory processes, at least in part. Ni-free stents avoid this activation of inflammatory processes by Ni2+.
Figure 5 Reduced Hif-1α activation in vascular walls receiving Ni-free stainless stents. (a) Immunohistochemical detection of HIF-1α within stented arterial walls 28 days after stent implantation. N: neointima, M: media, A: arterial lumens, S: stent struts. HIF-1α is shown in yellow and SM α-actin, a SMC marker, is shown in red. Scale bars: 50 μm. (b) Expression levels of the HIF-1α target genes Vegfa and Pdgfa and the inflammatory cytokine Tnf in the walls stented arteries. ∗P < 0.05 versus control, #P < 0.05 versus SUS316L stent.
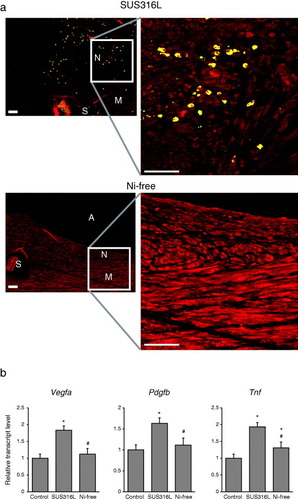
4. Discussion
In this study, we showed that Ni-free bare metal stents significantly reduce neointima formation, as compared to SUS316L stents, following implantation in porcine coronary arteries. We also showed that Ni2+ is released from SUS316L and that it activates the HIF-1α pathway in cultured SMCs in vitro. Within the walls of arteries receiving Ni-free stents, this inflammation, SMC proliferation and HIF-1α activation were avoided, suggesting that Ni2+ augments neointima formation by stimulating inflammatory processes, in part by activating HIF-1 signaling.
HIF-1α is a key transcription factor involved in mediating the hypoxic response and regulates cellular survival, metabolism and proliferation [Citation42]. It is also known to activate angiogenesis, and is reportedly involved in the development of such cardiovascular diseases as myocardial infarction, atherosclerosis, abdominal aortic aneurysm, pulmonary hypertension, systemic hypertension and peripheral artery disease [Citation43–Citation45]. In addition to hypoxia, HIF-1α can be activated by various stimuli, including cytokines such as IL-1β, IL-4 and TNF-α [Citation46, Citation47]. In SMCs, HIF-1α is activated by PDGF, FGF2, EGF and angiotensin II and mediates cell proliferation and neointima formation [Citation39, Citation48, Citation49]. In this study, we found less SMC proliferation and HIF-1α activation in coronary arteries receiving Ni-free stents, suggesting the lower level of HIF-1 signaling likely contributes to the diminished neointima formation seen with Ni-free stents.
Although HIF-1α is produced constitutively, under normoxic conditions it is hydroxylated by Fe2+-containing prolyl hydroxylases (PHDs), which utilize O2 as a cosubstrate for catalysis, and then undergo ubiquitination and proteosomal degradation [Citation50, Citation51]. Under hypoxic conditions, by contrast, PHD activity is suppressed, leading to nuclear accumulation of HIF-1α and transcriptional induction of its target genes [Citation50, Citation51]. In addition to hypoxia, Ni and Co ions also mediate HIF-1α activation, as these metal ions can substitute for Fe2+ at the Fe2+-binding site of PHDs, thereby inhibiting these enzymes [Citation52, Citation53]. Consistent with that effect, we found that the presence of Ni2+ led to HIF-1α activation and induction of HIF-1α target genes in cultured SMCs. Moreover, SUS316L stainless released Ni2+ into saline solution, whereas Ni-free stainless did not (figure (a)), and SUS316L stents activated HIF-1α signaling within the arterial wall more prominently than Ni-free stents (figure ). Collectively, these results strongly suggest that Ni2+ released from SUS316L stainless stents promotes neointima formation via activation of HIF-1α signaling (figure ).
The novel accomplishments summarized in this report are: (i) production of a coronary stent from Ni-free stainless steel, (ii) documentation of the favorable effects of the Ni-free stainless steel stent, as compared to the SUS316L stainless steel stent in vivo and (iii) provided evidence of a Ni2+-dependent HIF1-α pathway leading to activation in smooth muscle cells in vivo and vitro. These results also identify Ni-free stainless steel as an attractive coronary stent platform.
Acknowledgments
We gratefully acknowledge M Hayashi, N Yamanaka, X Yinda, Y Tani, K Iwazaki, M Miwa and H Tanimoto for their excellent technical assistance. This study was supported by the ‘Funding Program for World-Leading Innovative R&D on Science and Technology (FIRST Program)’ (to RN), Grants-in-Aid for Scientific Research (S) and (B) and Grants-in-Aid for Young Scientists (B) from JSPS (23390203, 20390220, 22229006, 23790835) (to RN, IM, KF); a grant for Translational Systems Biology and Medicine Initiative (to RN) from JST; and research grants from the Sumitomo Foundation, the Takeda Science Foundation, the Mochida Memorial Foundation for Medical and Pharmaceutical Research and the SENSHIN Medical Research Foundation (to IM).
References
- DussaillantG R 1995 J. Am. Colege Cardiol. 26 720 10.1016/0735-1097(95)00249-4
- FischmanD L 1994 New Engl. J. Med. 331 496 10.1056/NEJM199408253310802
- LauK WJohanASigwartUHungJ S 2004 Singapore Med. J. 45 305 15221045
- SerruysP W 1994 New Engl. J. Med. 331 489 10.1056/NEJM199408253310801
- StoneG W 2005 J. Am. Med. Assoc. 294 1215 10.1001/jama.294.10.1215
- MoriceM-C 2002 New Engl. J. Med. 346 1773 10.1056/NEJMoa012843
- De LucaG 2012 Arch. Intern. Med. 172 611 10.1001/archinternmed.2012.758
- LemosP A 2004 Circulation 109 190 10.1161/01.CIR.0000109138.84579.FA
- MeredithI T 2012 J. Am. College Cardiol. 59 1362 10.1016/j.jacc.2011.12.016
- StoneG W 2011 J. Am. College Cardiol. 57 1700 10.1016/j.jacc.2011.02.016
- McFaddenE P Lancet 364 1519 10.1016/S0140-6736(04)17275-9
- PalmeriniT 2012 Lancet 379 1393 10.1016/S0140-6736(12)60324-9
- FarbABoamA B 2007 New Engl. J. Med. 356 984 10.1056/NEJMp068304
- LevineG N 2011 J. Am. College Cardiol. 58 e44 10.1016/j.jacc.2011.08.007
- BergerP B 2010 Circulation 121 2575 10.1161/CIRCULATIONAHA.109.895342
- BrottB CHillegassW B 2012 Ann. Intern. Med. 157 JC2
- SharmaA B 2012 Cardiac Interv. Today 26
- NakazawaG 2011 J. Cardiol. 58 84 10.1016/j.jjcc.2011.07.004
- InoueK 2012 Thrombosis 2012 219389 10.1155/2012/219389
- PoncinPProftJ 2003 Stent tubing: understanding the desired attributes Conf. on Materials and Processes for Medical Devices
- SchmidtMGoebelerM 2011 J. Mol. Med. 89 961 10.1007/s00109-011-0780-0
- CostaM 2003 J. Environ. Monit. 5 222 10.1039/b210260a
- KosterR 2000 Lancet 356 1895 10.1016/S0140-6736(00)03262-1
- SaitoT 2009 Cardiovasc. Revasc. Med. 10 17 10.1016/j.carrev.2008.01.004
- Romero-BrufauS 2012 Circ. Cardiovasc. Interv. 5 220 10.1161/CIRCINTERVENTIONS.111.966614
- GoebelerM 1993 J. Invest. Dermatol. 100 759 10.1111/1523-1747.ep12476328
- GoebelerM 2001 Blood 97 46 10.1182/blood.V97.1.46
- KurodaD 2003 Mater. Trans. JIM 44 1363 10.2320/matertrans.44.1363
- SagaraM 2002 Tetsu To Hagane-J. Iron Steel Inst. Japan 88 672
- KatadaY 2004 Mater. Manuf. Process. 19 19 10.1081/AMP-120027495
- MaruyamaN 2009 Mater. Trans. 50 2615 10.2320/matertrans.M2009216
- VirmaniRFarbA 1999 Curr. Opin. Lipidol. 10 499 10.1097/00041433-199912000-00004
- PerkinsL E L 2009 J. Interv. Cardiol. 22 S28 10.1111/j.1540-8183.2009.00451.x
- ShimizuR T 1995 J. Biol. Chem. 270 7631 10.1074/jbc.270.13.7631
- FujiuK 2005 Circ. Res. 97 1132 10.1161/01.RES.0000190613.22565.13
- WatanabeK 2011 Bunseki Kagaku 60 557 10.2116/bunsekikagaku.60.557
- Glista-BakerE E 2012 Am. J. Respir. Cell Mol. Biol. 47 552 10.1165/rcmb.2012-0023OC
- PietruskaJ R 2011 Toxicol. Sci. 124 138 10.1093/toxsci/kfr206
- LambertC M 2010 Cardiovasc. Res. 88 196 10.1093/cvr/cvq152
- SemenzaG L 2006 Novartis Found. Symp. 272 2 (discussion 8–14, 33–16) 10.1093/cvr/cvq152
- KnowlesH J 2004 Circ. Res. 95 162 10.1161/01.RES.0000134924.89412.70
- SemenzaG L 2009 Blood 114 2015 10.1182/blood-2009-05-189985
- SemenzaG L 2001 Trends Mol. Med. 7 345 10.1016/S1471-4914(01)02090-1
- BonnetS 2006 Circulation 113 2630 10.1161/CIRCULATIONAHA.105.609008
- TuderR M 2001 J. Pathol. 195 367 10.1002/path.953
- Hellwig-BürgelT 1999 Blood 94 1561 10477681
- JiangH 2010 Am. J. Physiol., Lung Cell. Mol. Physiol. 298 L660 10.1152/ajplung.00394.2009
- RichardD E 2000 J. Biol. Chem. 275 26765 10.1074/jbc.M003325200
- SchultzK 2006 Am. J. Physiol. Heart Circ. Physiol. 290 H2528 10.1152/ajpheart.01077.2005
- HayaishiONozakiM 1969 Science 164 389 10.1126/science.164.3878.389
- JaakkolaP 2001 Science 292 468 10.1126/science.1059796
- MaxwellPSalnikowK 2004 Cancer Biol. Ther. 3 29 10.4161/cbt.3.1.547
- BatieC J 1987 J. Biol. Chem. 262 1510 3805038