Abstract
Polymeric micelles with core–shell–corona nanoarchitecture were designed for intracellular therapeutic anti-cancer drug carriers. Poly(styrene-b-acrylic acid-b-ethylene glycol) (PS-b-PAA-b-PEG) asymmetric triblock copolymer underwent self-assembly in aqueous solution to form spherical micelles with hydrophobic PS core, anionic PAA shell and hydrophilic PEG corona. The anti-cancer drug (doxorubicin, DOX) was successfully incorporated into the polymeric micelles. The in vitro release experiment confirmed that the release of DOX from the micelles was inhibited at pH 7.4. In contrast, an accelerated release of DOX was observed at mildly acidic conditions such as pH 4.5. The excellent biocompatibility of our PS-b-PAA-b-PEG-based micelles made the synthesized nano-carrier best suited for the delivery of anti-cancer drugs.
1. Introduction
In biomedical fields, various nanomaterials have been utilized for different purposes such as therapy, diagnostics and drugs [Citation1–Citation6]. Amphiphilic block copolymers have been extensively studied to develop various carriers for drugs, dyes and si-RNA [Citation7–Citation10]. One can adjust the chemical nature of block copolymer as well as the molecular characteristics (molecular weight, composition and functional group) in order to optimize the performance [Citation11–Citation13]. Polymeric micelles usually form spherical core–shell structures in which the hydrophobic core serves as the microenvironment for the encapsulation of a wide range of hydrophobic agents and the hydrophilic shell serves as a stabilizing interface between the hydrophobic core and the external medium. Various PEG–hydrophobic block combinations have given rise to a number of functional micelle systems, in which the PEG units are important for increasing the suitability as drug carriers [Citation14, Citation15]. Ringsdorf et al reported polymeric micelles as drug carriers using poly(ethylene glycol-b-l-lysine) and cyclophosphamide as block copolymer and anti-cancer drugs, respectively [Citation16]. Kataoka et al also developed a similar approach using poly(ethylene oxide-b-aspartic acid) block copolymer modified with DOX [Citation17]. Eisenberg et al reported the application of poly(acrylic acid-b-styrene) (PAA-b-PS) vesicles as drug carriers [Citation18]. The vesicles prepared from PAA-b-PS were spherical bilayers in which the insoluble PS blocks constitute the vesicle wall, whereas PAA chains extend from the inner and outer surfaces. These vesicles offer a hydrophilic reservoir, suitable for the incorporation of water-soluble molecules, as well as a hydrophobic wall that protects the loaded molecules from the external solution.
Double-hydrophilic block copolymers (DHBC) consisting of two different hydrophilic blocks are likely to provide more applications in the biomedical fields because their micelles can be used as carriers for both ionic and nonionic drugs [Citation19, Citation20]. However, when they reach the bloodstream, the micelles completely collapse within a short interval of time. This is because the micelles are in dynamic equilibrium. This decreases the bioactivity of the drugs, leading to an ineffective treatment. Thus, it is essential to find a new micelle system of frozen nature so that it takes several hours to take equilibrium between unimers and micelles even when it is diluted in the blood stream.
Advances in polymer chemistry make it possible to synthesize an infinite number of block copolymers with sophisticated structures [Citation21]. Triblock copolymers with chemically distinct domains possess many advantages over diblock amphiphilic block copolymer and DHBC [Citation22–Citation25]. Armes and co-workers successfully loaded a model drug dipyridamole into the micelles of ABC triblock copolymer of methoxy-capped poly[ethylene glycol-b-2-(dimethyl amino)ethylmethacrylate-b-2-(diethylamino)ethyl methacrylate] by controlling the pH [Citation26]. As another example, micelles made of poly(styrene-b-2-vinyl pyridine-b-ethylene oxide) (PS-b-PVP-b-PEO) were used as a carrier for the anionic drug coaxicillin sodium [Citation27]. The drug was released significantly faster at physiological pH (7.4) compared to the acidic pH (3.0). Ideally, the nanocarrier should release the drug faster in mildly acidic conditions than at physiological pH.
In this study, we exploit the usefulness of newly synthesized asymmetric triblock copolymer (PS-b-PAA-b-PEG) as an anti-cancer drug carrier. The rigid hydrophobic PS core can stabilize the nano-carriers in extreme dilute condition, the anionic PAA is the reaction site for several cationic drugs and the hydrophilic PEG corona stabilizes each particle in aqueous media. Thus, the core–shell–corona architecture of newly synthesized polymeric micelles can serve as multi-functional drug carriers.
2. Experimental details
2.1. Materials
PS-b-PAA-b-PEG block copolymer was synthesized by RAFT (reversible addition fragmentation chain transfer) polymerization as reported previously [Citation28]. Doxorubicin hydrochloride (DOX), 4′,6-diamidino-2-phenylindole (DAPI), 3-(4,5-dimethylthiazol-2-yl)-2,5-diphenyltetrazolium bromide, and dimethyl sulfoxide (DMSO, 99.5%) were all purchased from Sigma-Aldrich.
2.2. Preparation of the micellar solution
0.1 g of PS-b-PAA-b-PEG block copolymer was first dissolved in N, N-dimethylformamide (DMF). After complete dissolution, it was subjected to dialysis against pure water. An aqueous solution of micelles was collected after the complete removal of DMF. The aqueous solution of micelles was diluted to 100 ml to make the final concentration 1 g l−1 and the pH was maintained at 7.4 using PBS buffer. DOX was titrated with pure PS-b-PAA-b-PEG micelle solution at pH 7.4. The added amount of DOX was expressed in terms of molar ratio. The solution was stirred with a magnetic stirrer for 1 h at room temperature to accelerate the interaction between the micelles and DOX.
2.3. Characterization
The dynamic light scattering (DLS) measurement was carried out using an Otsuka ELS Z zeta potential and particle analyzer. All the measurements were carried out at 25 °C. The scattered light of a vertically polarized He–Ne laser (632.8 nm) was measured at an angle of 90° and was collected by an autocorrelator. The correlation functions were analyzed by the CONTIN method and used to determine the diffusion coefficient (D) of the particles. The hydrodynamic diameter (Dh) was calculated from D using the Stokes–Einstein equation (Dh = kBT/(3πηD), where kB is the Boltzmann constant, T is the absolute temperature and η is the solvent viscosity. The electrophoretic mobility (EPM) was also measured. The zeta potential (ζ) was calculated from the EPM using the Smoluchowski equation (μE = ζε/η, where μE is the EPM, ε is the permittivity of solvent and η is the solvent viscosity). The fluorescence spectra were recorded by a JASCO FP-6500 fluorescence spectrophotometer (right angle geometry, 1 cm × 1 cm quartz cell). The band widths were 3 and 1 nm on the excitation and emission sides, respectively. The UV–Vis absorption spectra were recorded on a JASCO V-570 UV/VIS/NIR spectrophotometer. The morphology of the polymeric micelles was observed by transmission electron microscopy (TEM) (JEOL JEM-1210). One drop of micellar solution was placed on the TEM grid and dried in air after being negative-stained with phosphotungstic sodium solution (0.1%). The surface morphology was observed by atomic force microscopy (AFM) (Bruker Nanoscope).
2.4. Cell experiment
BT-20 human breast cancer cells (ATCC® Number: HTB-19™) were used in this study. They were cultured in flasks with the MEM medium supplemented with 10% fetal bovine serum, 2% sodium bicarbonate, 1% l-glutamine and 1% penicillin at 37 °C under a humidified atmosphere containing 5% CO2. Cell viability was investigated using the MTT assay. First, BT-20 cells were cultured in a 24-well culture plate at a density of 1.0 × 105 cells per well, and allowed to attach overnight. The cell-attached plate was then washed with PBS solution three times, and immersed in serum-free MEM medium (0.5 ml well−1) containing different concentrations of nanoparticles or drug-loaded nanoparticles. After incubation for different periods, the nanoparticle-immersed plate was washed several times with PBS solution to remove the nanoparticle residue. The MTT stock solution (5 mg ml−1) was then diluted ten times with serum-free MEM medium and added to each well (0.5 ml well−1). These cells were further incubated for 4 h to allow the yellow dye to transform into blue formazan crystals. The unreacted dye was then removed by aspiration, and DMSO (400 μl) was added to each well to dissolve the blue formazan crystals. Finally, the dissolved DMSO solution was transferred to a 96-well culture plate (100 μl well−1), and its optical density was measured with an Elisa Reader at a wavelength of 570 nm.
3. Results and discussion
The polymeric micellar solution was prepared by using the dialysis method [Citation29]. The PS-b-PAA-b-PEG polymer was completely dissolved into DMF, which has been identified as a good solvent for all the blocks of the polymer. The micelles in aqueous solution were collected by solvent exchange during dialysis. According to the hydrophilic/hydrophobic properties of the three blocks, the self-assembled micelle contains three layers; a hydrophobic PS core, an anionic PAA shell and a hydrophilic PEG corona [Citation30, Citation31]. The formation of micelles was observed using light scattering measurement and the hydrodynamic diameter was found to be 80 ± 5 nm at pH 7.4. The TEM observation (figure (a)) provides concrete evidence for the formation of micelles. The difference in the particle size obtained from DLS measurement and TEM observation is attributed to the thickness of the extended PAA shell and PEG corona.
Figure 1. (a) TEM image of PS-b-PAA-b-PEG micelles and (b) AFM image of DOX/PS–PAA–PEG. The scale bar is 50 nm.
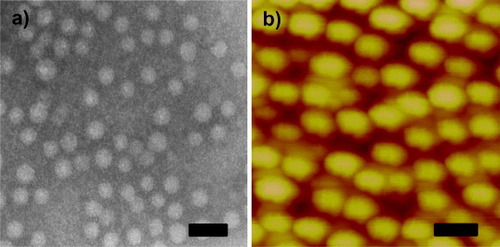
The size of the polymeric micelles can be tuned by changing the conformation of the PAA shell. PAA is a weak polyelectrolyte with a carboxylic acid group whose pKa is 4.6 [Citation32]. For pH < pKa, most carboxylic groups exist as the protonated form (COOH), whereas for pH > pKa, most carboxylic groups exist as the deprotonated form (COO−) [Citation33]. Thus, with an increase in pH, the carboxylic groups change from the protonated form to the deprotonated one. There is significant electrostatic repulsion between the deprotonated carboxylic groups. Therefore, with an increase pH, the PAA block undergoes a conformational change from shrunken to extended forms. The Dh at pH 7.4 (80 nm) was much larger than that at pH 4.5 (50 nm), as shown in figure . Such pH-responsive behavior of the PAA block could be useful for pH-triggered drug delivery. Another appealing feature of our carrier system is the presence of hydrophobic PS core. We estimated the glass-transition temperature of the PS block from its molecular weight [Citation34] and obtained a value of 88 °C. Block copolymers containing hydrophobic block with a high glass-transition temperature (higher than room temperature) usually form the frozen micelles. The micelles with frozen core exhibit superb stability upon dilution.
The electrostatic interaction between the anionic PAA shell and cationic DOX at pH 7.4 is the basis for the drug binding to the polymeric micelles. Figure (a) shows the absorbance spectra for DOX and PS-b-PAA-b-PEG micelles with DOX (hereafter as abbreviated as DOX/PS-PAA-PEG). Red shift (around 10 nm) of λmax in the spectra indicates the formation of DOX/PS–PAA–PEG by electrostatic interaction between DOX and PAA block [Citation35]. The interaction of DOX with PAA was also confirmed by fluorescence spectra, as shown in figure (b). Fluorescence characteristics of DOX are sensitive to changes in the microenvironment [Citation36]. Polymeric micelles themselves are not fluorescent quenchers. However, after mixing DOX with micelle solution, the fluorescent intensity is sharply reduced, indicating the stacking interaction of DOX with micelles. The reduced fluorescence intensity of DOX in the presence of PS-b-PAA-b-PEG micelles is related to a self-quenching of bound DOX [Citation37].
Figure 3. (a) Absorption and (b) fluorescence spectra of (1) DOX and (2) DOX/PS–PAA–PEG, respectively.
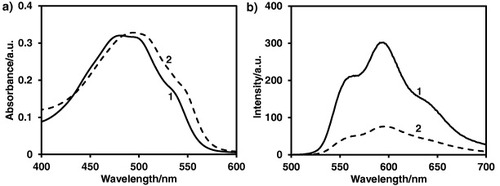
The effect of the added DOX amounts on Dh and the surface charge is shown in figure . Zeta-potential data indicated that polymeric micelles have a net surface charge of around −40 mV (figure (b)). When DOX was introduced into PS-b-PAA-b-PEG micellar solutions, the DOX preferentially binds with PAA blocks and neutralizes the negatively charged PAA blocks (figure (b)). The net surface charge was reduced to −10 mV, indicating the cancellation of anionic PAA with DOX. After the complexation reaction, Dh decreased to 50 nm (figure (a)). The decrease in Dh is attributed to the neutralization of the anionic acrylic group of the PAA block by the positively charged DOX.
Figure 4. (a) Dh and (b) ζ of DOX/PS–PAA–PEG as a function of DOX molar ratio. The concentration of polymer is fixed at 0.2 g l−1.
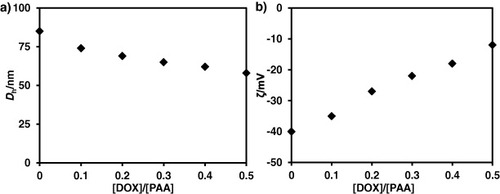
As shown in figure , the particle size of DOX/PS–PAA–PEG varied from 50 to 80 nm, depending on their compositional ratios. This is extremely suitable for a drug carrier because this size prevents elimination via either renal excretion or mechanical filtration by inter-endothelial cell slits in the spleen [Citation38]. Furthermore, the obtained DOX/PS–PAA–PEG is very stable for several months, as checked by DLS measurement and AFM observation (figure (b)). The maximum amount of DOX incorporated into the micelles was 1.5 g g−1 of polymer. This remarkably high loading capacity of the core–shell–corona micelles is valuable from a clinical point of view as it minimizes the total dose of drug carrier.
The release study was carried out in vitro using dialysis followed by UV–Vis absorption measurement at 37 °C at different pH levels, i.e. 4.5 and 7.4, which are the most commonly used conditions (figure ). The amount of released drug was monitored at the outside of the dialysis tube. The solution (3.0 ml) outside the dialysis tube was taken off at definite time intervals to measure the amount of released DOX. The absorbance of the DOX was recorded at 496 nm. The solution after measurement was immediately returned to the solution outside the dialysis tube. It was observed that the drugs were released in a sustainable manner without burst release. At mildly acidic pH (4.5), the drug release was significantly faster compared to physiological pH (7.4). The pH of the tumor cell is more acidic than blood and normal tissues (pH = 7.4). The acrylic acid is a weak electrolyte. At low pH, the protonation of carboxylic acid weakens the electrostatic interaction of DOX with PAA, resulting in faster drug release.
To demonstrate that the synthesized polymeric micelles can be used as a drug carrier that can penetrate cell membrane and deliver anti-cancer drug into cancer cells, we chose a human breast cancer cell line BT-20 for intracellular drug delivery systems. The viability of the BT-20 cells was evaluated by MTT assay after 4 h with different dosages of DOX/PS–PAA–PEG and PS-b-PAA-b-PEG micelles. As shown in figure , in contrast to the PS-b-PAA-b-PEG micelles showing excellent viability even at high concentrations, the viability of cells treated with DOX-loaded micelles (DOX/PS–PAA–PEG) gradually decreased when the DOX/PS–PAA–PEG concentration increased, indicating a dose-dependent effect. Thus, our DOX/PS–PAA–PEG exhibited high efficacy in killing BT-20 cancer cells. Remarkably, more than 60% of BT-20 cells were killed at a dosage of 250 μg ml−1 in 4 h. The acid-assisted protonation of the PAA shell facilitated drug release in acidic endosomes or lysosomes after endocytosis, resulting in enhanced cell death.
4. Conclusions
A newly synthesized asymmetric triblock copolymer was used as a nanocarrier for an anti-cancer drug, DOX. DOX was successfully incorporated into the polymeric micelles made of PS-b-PAA-b-PEG block copolymer, and the DOX-loaded micelles were successfully used in the therapeutic treatment of human breast cancer cells, BT-20. Absorption, fluorescence and zeta-potential measurement confirmed the binding of drug to anionic PAA block. The pH shell swelled or shrank with the variation of environmental pH to control the release of DOX. The synergic contribution of hydrophobic PS, pH-sensitive PAA and hydrophilic PEG and superb biocompatibility is highly useful for delivery of anti-cancer drugs.
References
- MiyaharaYKobayashiHChenGKikuchiM 2010 Sci. Technol. Adv. Mater. 11 010302 10.1088/1468-6996/11/1/010302
- XingZ CChangYKangI K 2010 Sci. Technol. Adv. Mater. 11 014101 10.1088/1468-6996/11/1/014101
- SmithI OMaP X 2010 Sci. Technol. Adv. Mater. 11 014102 10.1088/1468-6996/11/1/014102
- SundarSKunduJKunduS C 2010 Sci. Technol. Adv. Mater. 11 014104 10.1088/1468-6996/11/1/014104
- OhnishiHOdaYOhgushiH 2010 Sci. Technol. Adv. Mater. 11 014110 10.1088/1468-6996/11/1/014110
- GreenR ABaekSPoole-WarrenL AMartensP J 2010 Sci. Technol. Adv. Mater. 11 014107 10.1088/1468-6996/11/1/014107
- AlexanderN Z 2010 ACS Nano 4 2494 10.1021/nn100634r
- DevadasuV RBhardwajVKumarM N V R 2012 Chem. Rev. 10 1686
- LarsonNGhandehariH 2012 Chem. Mater. 24 840 10.1021/cm2031569
- DasS KBhuniaM KChakrabortyDBukhshA R KBhaumikA 2011 Chem. Commun. 48 2891 10.1039/c2cc17181c
- BajpaiA KShuklaS KBhanuSKankaneS 2008 Prog. Polym. Sci. 33 1088 10.1016/j.progpolymsci.2008.07.005
- OmelczukM OMcGinityJ W 1992 Pharm. Res. 9 26 10.1023/A:1018967424392
- KumariAYadavS KYadavS C 2010 Colloids Surf. B 75 1 10.1016/j.colsurfb.2009.09.001
- CarstensM GBevernageJ J LNostrumC FSteenbergenM JFleschF MVerrijkRLeedeL G JCrommelinD J AHenninkW E 2007 Macromolecules 40 116 10.1021/ma0619738
- VellutoDDemurtasDHubbellJ A 2008 Mol. Pharmaceutics 5 632 10.1021/mp7001297
- BaderHRingsdorfHSchmidtB 1984 Angew. Makromol. Chem. 123 457 10.1002/apmc.1984.051230121
- YokoyamaMInoueSKataokaKYuiNSakuraiY 1987 Makromol. Chem. Rapid Commun. 8 431 10.1002/marc.1987.030080903
- ChoucairASooP LEisenbergA 2005 Langmuir 21 9308 10.1021/la050710o
- ColfenH 2001 Macromol. Rapid Commun. 22 219 10.1002/1521-3927(20010201)22:4%3C219::AID-MARC219%3E3.0.CO;2-G
- NakashimaKBahadurP 2006 Adv. Colloid Interface Sci. 123–126 75 10.1016/j.cis.2006.05.016
- KowollikC B 2008 Handbook of RAFT Polymerization New York Wiley 543 pp
- FustinC AAbetzVGohyJ F 2005 Eur. Phys. J. E 16 291 10.1140/epje/i2004-10086-0
- BastakotiB PGuragainSYonedaAYokoyamaYYusaSNakashimaK 2010 Polym. Chem. 1 347 10.1039/b9py00231f
- BastakotiB PGuragainSYokoyamaYYusaSNakashimaK 2011 Colloid Surf. B 88 734 10.1016/j.colsurfb.2011.08.009
- BastakotiB PGuragainSYokoyamaYYusaSNakashimaK 2010 Colloid Polym. Sci. 288 991 10.1007/s00396-010-2223-9
- TangYLiuS YArmesS PBillinghamN C 2003 Biomacromolecules 4 1636 10.1021/bm030026t
- KhanalANakashimaK 2005 J. Control. Release 108 150 10.1016/j.jconrel.2005.07.018
- BastakotiB PGuragainSYokoyamaYYusaSNakashimaK 2011 Langmuir 27 379 10.1021/la103660x
- RiessG 2003 Prog. Polym. Sci. 28 1107 10.1016/S0079-6700(03)00015-7
- BastakotiB PInuoeMYusaSLiaoSWuK C-WNakashimaKYamauchiY 2012 Chem. Commun. 48 6532 10.1039/c2cc32279j
- BastakotiB PWuK C-WInoueMYusaSNakashimaKYamauchiY 2013 Chem. Eur. J. 19 4812 10.1002/chem.201203958
- KajiwaraKRossS B M 1992 Nature 355 208 10.1038/355208a0
- LudwigsSSchmidtKKrauschG 2005 Macromolecules 38 2376 10.1021/ma047812q
- JadaAHurtrezGSiffertBRiessG 1996 Macromol. Chem. Phys. 197 3697 10.1002/macp.1996.021971117
- KitaevaM VMelikN N SMengerF MYaroslavovA A 2004 Langmuir 20 6575 10.1021/la0497144
- HusainNAghariaR AWarnerI M 1993 J. Phys. Chem. 97 10857 10.1021/j100143a054
- TianYRaviPBrombergLHattonT ATamK C 2007 Langmuir 23 2638 10.1021/la060780a
- TorchilinV P 2001 J. Control. Release 73 137 10.1016/S0168-3659(01)00299-1