Abstract
Ti-doped barium ferrite powders BaFe12−xTixO19 (x = 0, 0.2, 0.3, 0.4, 0.5, 0.6, 0.7 and 0.8) were synthesized by the sol–gel method. The phase structure and morphology were analyzed by x-ray diffraction (XRD) and scanning electron microscopy, respectively. The powders were also studied for their magnetic properties and microwave absorption. Results show that the Ti-doped barium ferrites (BFTO) exist in single phase and exhibit hexagonal plate-like structure. The anisotropy field Ha of the BFTO decreases almost linearly with the increase in Ti concentration, which leads to a shift of the natural resonance peak toward low frequency. Two natural resonance peaks appear, which can be assigned to the double values of the Landé factor g that are found to be ∼2.0 and ∼2.3 in the system and can be essentially attributed to the existence of Fe3+ ions and the exchange coupling effect between Fe3+ and Fe2+ ions, respectively. Such a dual resonance effect contributes a broad magnetic loss peak and thus a high attenuation constant, and leads to a dual reflection loss (RL) peak over the frequency range between 26.5 and 40 GHz. The high attenuation constants are between 350 and 500 at peak position. The optimal RL reaches around −45 dB and the practicable frequency bandwidth is beyond 11 GHz. This suggests that the BFTO powders could be used as microwave absorbing materials with extraordinary properties.
1. Introduction
Recently, electronic devices such as local-area networks, mobile phones, satellite television sets and radar systems have been widely used for wireless communication based on electromagnetic (EM) waves in the gigahertz (GHz) range with the advantage of large data transmission [Citation1, Citation2]. Meanwhile, the emergence of EM interference, EM wave pollution and other problems has triggered extensive studies on the applications of microwave absorbing materials, which can absorb unwanted EM signals and are expected to be used in military technology, microwave darkrooms, human health, etc [Citation3, Citation4]. Researchers all around the world have focused on obtaining novel materials with excellent microwave absorption properties, i.e. strong absorption and broad bandwidth. Microwave radiation with the frequency range between 26.5 and 40 GHz has the characteristics of both centimeter waves and millimeter waves. Materials with microwave absorption properties can thus work not only as all-weather materials but also as high-resolution probes. Today there are many kinds of radar with the 26.5–40 GHz wave band being widely applied. So it is very important to have extensive research on microwave absorption properties over the frequency range between 26.5 and 40 GHz.
Microwave absorbing ability depends strongly on material properties, including complex permeability, complex permittivity and resistivity. Ferrites exhibit outstanding microwave absorption properties and are widely employed in military and civil fields due to their high resistivity and severe EM energy attenuation, especially near the natural resonance frequency of magnetic moments [Citation5–Citation8]. As for the M-type barium ferrite BaFe12O19 (BFO), its fairly large magnetic anisotropy, large saturation magnetization, high coercive force and excellent chemical stability make it one of the most potential microwave absorbers in the future [Citation9, Citation10]. As a matter of fact, the natural resonance, which is related to the appearance of Fe3+ ions in the ferrite, contributes to magnetic loss strongly and thus a high attenuation constant as well as a strong EM wave loss at the resonant frequency. In addition, the magnetic properties of the M-type barium ferrite depend strongly on the substitution of Fe3+ ions in different sites by other cations or cationic combinations, including Al3+, Cr3+, Co2+–Ti4+, Co2+–Ru4+ and so on [Citation11–Citation13]. As is known, if the low positively charged Fe3+ ions are substituted by foreign high positively charged ions in the ferrite, the rest of the Fe3+ ions will convert into Fe2+ ions in response to the remnant electrical neutrality [Citation14–Citation17] and an exchange interaction between Fe2+ and Fe3+ ions will occur [Citation18]. Consequently, a new natural resonance with respect to the exchange coupling between Fe2+ and Fe3+ ions will appear in the ferrite, but the resonant frequency will be different from that related to the Fe3+ ions. This implies that if the exchange coupling occurs in the ferrite, a new resonance peak and thus a new magnetic loss peak are expected to appear at another frequency position resulting from the Fe3+ ions [Citation19, Citation20]. Therefore, double natural resonance peaks will be generated simultaneously in the ferrite in this case. It will probably bring about two magnetic loss peaks and two absorption peaks, which expand the absorption bandwidth in the EM wave spectrum. Thus, it is attractive to obtain the ferrite with excellent absorption properties.
Herein, we propose the Ti-doped barium ferrites BaFe12−xTixO19 (BFTO) synthesized by the sol–gel method. It is worth noting that BFO is a typical ferrite with strong EM wave absorption and Ti4+ is a typical dopant with a relatively high electron valence as well as a close radius compared to that of Fe3+ in the ferrite [Citation21, Citation22]. In this case, the Ti4+ ions could easily substitute the Fe3+ ions in the BFO ferrite to engender exchange coupling between Fe3+ and Fe2+ in addition to the existence of Fe3+ centers. In this work, the resonances with respect to both Fe3+ ions and the exchange coupling between Fe3+ and Fe2+ were investigated in detail. The dual magnetic loss peaks with different magnetic resonances were successfully obtained and controlled in the Ti-doped ferrite. A broad bandwidth beyond 11 GHz was obtained with dual reflection loss (RL) peaks over the frequency range from 26.5 to 40 GHz.
2. Experimental procedure
Ti-doped barium ferrite powders were synthesized by the sol–gel method. Barium nitrate (Ba(NO3)2), tetrabutyl titanate (Ti(OC4H9)4), ferric nitrate nonahydrate (Fe(NO3)3·9H2O), citric acid, ammonia, absolute ethyl alcohol and deionized water were selected as raw materials. According to the composition of BaFe12−xTixO19 (x = 0, 0.2, 0.3, 0.4, 0.5, 0.6, 0.7 and 0.8), the metal nitrates and an appropriate amount of citric acid were initially dissolved in the deionized water by stirring to obtain a clear solution (1), and then specific amounts of Ti(OC4H9)4 and citric acid were dissolved in absolute ethyl alcohol by stirring to get a clear solution (2). Solution (2) was slowly added into solution (1) to get a clear solution (3), and then ammonia was used to adjust the pH value to 7.0. The obtained solution was evaporated with stirring to form viscous sol precursors, then dried at 120 °C for 24–48 h and heat treated at 1200 °C for 3 h to get BFTO powders.
The phase structures of the ferrite powders were identified by XRD (PANalytical B V Empyrean 200895, Cu Kα radiation). The microstructure studies were conducted by scanning electron microscopy (SEM) (Hitachi SU-70 FESEM). The magnetic properties were measured by a magnetic property measurement system (MPMS-XL-5).
The microwave absorbing properties were measured by an Agilent vector network analyzer (E8363C PNA) and the RL was calculated by EM parameters in the frequency range of 26.5–40 GHz. The samples were mixed with paraffin initially with a weight ratio of 8:3 and then heated at 80 °C, and then the mixtures were filled into holders of different thickness for the measurement of EM parameters.
3. Results and discussion
Figure shows the XRD patterns of the BaFe12−xTixO19 (x = 0, 0.3, 0.6 and 0.8) powders sintered at 1200 °C for 3 h. It is seen that all the BFTO powders with different titanium contents exhibit only the M-type barium ferrite phase. The lattice constants ‘a' and ‘c' are listed in table , and they slowly increase with increasing Ti content. Moreover, the ratio of ‘c/a' also increases due to the larger increase of the c-axis than the a-axis with increasing Ti4+ ions because the ionic radius of Ti4+ (0.68 Å) is larger than that of Fe3+ (0.64 Å) [Citation23].
Figure 1. XRD patterns of BaFe12−xTixO19 (x = 0, 0.3, 0.6 and 0.8) powders synthesized at 1200 °C for 3 h.
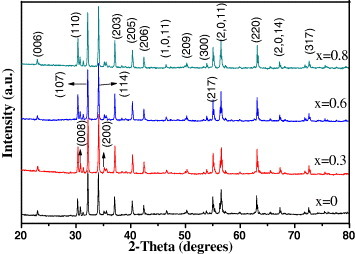
Table 1. Lattice constants ‘a' and ‘c' of the ceramic powder BaFe12−xTixO19 with x values of 0, 0.3, 0.6 and 0.8.
Figure shows the SEM morphologies of the BaFe12−xTixO19 (x = 0, 0.3, 0.6 and 0.8) ferrite powders. It is seen that typical plate-like particles of M-type hexagonal barium ferrite form in all samples. Particle size varies between 400 nm and 2 μm. It reduces initially and then increases gradually with increasing Ti content in the ferrite. When x = 0.3–0.4, the mean particle size is minimum and only about 600 nm. It grows bigger with increasing Ti content especially above x = 0.5, which is from about 600 nm at x = 0.4 to 2 μm at x = 0.8.
Figure 2. SEM photographs of BaBaFe12−xTixO19 powders synthesized at 1200 °C for 3 h with (a) x = 0, (b) x = 0.2, (c) x = 0.3, (d) x = 0.4, (e) x = 0.5, (f) x = 0.6, (g) x = 0.7 and (h) x = 0.8.
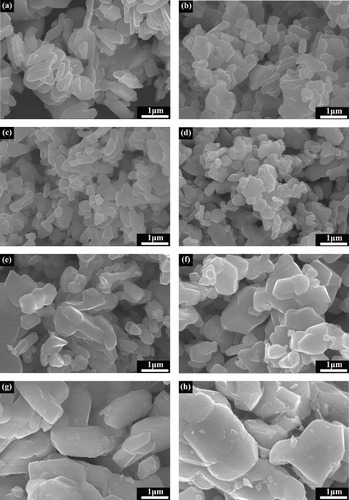
The magnetic hysteresis loops of BaFe12−xTixO19 with x = 0, 0.3, 0.6 and 0.8 are shown in figure (a). It can be found that the magnetization increases steeply at first and then slowly with increasing applied magnetic field, and the areas of the hysteresis loops as well as the coercive force (Hc) are typically large. All the ferrites approach saturation under an applied field of up to 3 T. The saturation magnetization (Ms) and the anisotropy field (Ha) of all the samples are obtained according to the law of approach to saturation (LAS), which is an effective method to describe the magnetization properties of polycrystalline magnetic material in approaching the saturation stage [Citation24]. According to LAS, magnetization (M) versus magnetic field (H) can be expressed as [Citation25]
1
where A is the inhomogeneity parameter that can be neglected at high fields, B is the anisotropy parameter and χp is the high-field differential susceptibility. Under the situation of hexagonal symmetry, B may be expressed as B = Ha2/15 [Citation24]. Using the experimental data within the high-field range (approaching saturation) as shown in figure (a) and equation (Equation1
1 ), both Ms and Ha can be obtained.
Figure 3. Plots of (a) hysteresis loops of BaFe12−xTixO19 ferrites with x between 0 and 0.8 measured at room temperature and (b) coercive force (Hc), saturation magnetization (Ms) and anisotropy field (Ha) of the ferrites as a function of Ti content (x).
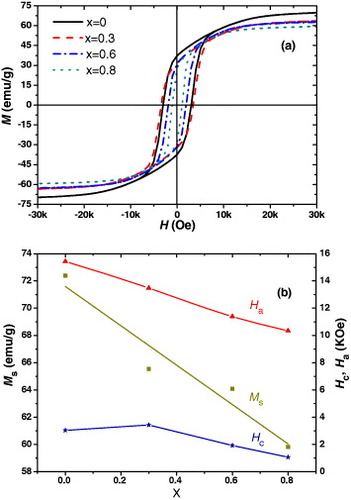
Figure (b) shows the dependence of Hc, Ms and Ha on Ti content. Note that Ha decreases linearly from 15.43 to 10.34 kOe with x changing from 0 to 0.8. Likewise, there is also a reduction in Ms, but not very great, from 72.38 emu g−1 (x = 0) to 59.82 emu g−1 (x = 0.8). However, Hc increases from 3.03 to 3.43 kOe when x changes from 0 to 0.3 initially and then decreases greatly from 3.43 to 1.06 kOe when x is between 0.3 and 0.8.
It is known that the Fe3+ ions are located in three kinds of coordination sites including five different crystallographic points in the magnetoplumbite phase structure of BFO. The first is the octahedral site including three different shapes represented as 12k, 2a and 4f2, respectively; the second is the trigonal bipyramid site represented as 2b; the third is the tetrahedral site represented as 4f1. The Fe3+ ions with up-spin are filled into the 12k, 2a and 2b sites, while they are in the 4f1 and 4f2 sites with spin in the opposite direction. It has been reported that the Ti4+ ions prefer the octahedral sites of 12k, 2a and 4f2 for stability because of their rare gas outer electron shell structure [Citation26, Citation27]. They can also occupy the 2b site at high doping levels [Citation16]. For the magnetic properties of the ferrite, the anisotropy field Ha is related to the sites occupied by Ti4+ ions. When the Fe3+ ions are substituted in different sites by Ti ions, a different anisotropy field in the ferrite will be induced. As is known, the Fe3+ ions in the 4f2 and 2b sites greatly contribute to the anisotropy field [Citation25]; thus the decrease in Ha exhibits the preferential replacement of Fe3+ ions in the 4f2 and 2b sites by Ti ions, although the substitution of Fe3+ ions by Ti increases slightly in the anisotropy field due to the lattice distortion as shown in table . The more substitution, the lower the Ha of the ferrite, as shown in figure (b). In addition, the Fe3+ ions with up-spin substituted by non-magnetic Ti ions reduce the magnetization of the ferrite, while the ferrite in which the Fe3+ ions with down-spin are substituted leads to an increase in magnetization [Citation28]. The slow reduction of Ms as shown in figure reveals likely more substitution of Ti4+ ions for Fe3+ ions in the 12k, 2a and 2b sites than in the 4f2 site. That is to say, the substitution of Ti4+ ions into 2b instead of other sites is the most probable preferential process in the BFTO ferrite. Moreover, the coercive force depends strongly on the anisotropy field [Citation26]. But it also changes in general with the grain size of the phase particles [Citation29]. The smaller the phase particle, the larger the Hc due to the large density of the grain boundary and thus more pinning centers that impede domain wall motion. As is shown in figure , the particle size of the ferrite powders decreases with increasing x to 0.3 and then increases with increasing x up to 0.8. As a result, Hc increases below x = 0.3 and then decreases above 0.3 as shown in figure (b). However, the trend of the coercive force that depends on the doping content of Ti still shows some decrement in the ferrite. This implies that the coercive force is controlled dominantly by Ha based on the substitution of Fe by Ti in the 2b site. Obviously, the Ti4+ ions doped into the ferrite contribute mainly a large part of substitution for Fe3+ ions in the sites of 2b in the magnetoplumbite phase structure of BFTO.
Figure shows the frequency dependence of the real part (ε′) and the imaginary part (ε″) of the complex permittivity and the real part (μ′) and the imaginary part (μ″) of the complex permeability for the BFTO samples over 26.5–40 GHz, respectively. There are no significant differences between ε′ (and ε″) in the ferrites with and without doping of Ti, although the ε′ values of all doped samples are larger than those that are undoped over the whole frequency range. However, the typical resonance phenomena of the magnetic moments can be seen for all the samples with doping Ti4+ ions. The imaginary part of the complex permeability μ″ increases from a low to high of about 0.5 and then decreases with frequency. The resonance peaks are asymmetric, which is more obvious in the samples with high Ti content. When x = 0.7 and 0.8, a distinct shoulder appears in μ″ depending on frequency. In general, the magnetic loss of a ferromagnetic material mainly originates from magnetic hysteresis loss, domain wall resonance, eddy current loss and natural resonance [Citation30, Citation31]. The magnetic hysteresis loss can be negligible under a weak applied field. The domain wall resonance mainly occurs in the frequency range lower than a certain GHz. The eddy current effect is responsible mainly for magnetic loss in low-resistivity materials [Citation32], but not for M-type BaFe12O19 with high resistivity [Citation9]. Doping Ti may make probably a contribution to enhancing the eddy current effect with decreasing resistivity, although M-type BaFe12O19 has high resistivity. However, it is limited and it can thus be ignored compared to natural resonance loss. Therefore, the magnetic loss peaks are mainly dependent on the natural resonance in the BFTO ferrites in the frequency range of 26.5–40 GHz. The resonance frequency (fr) obviously shifts toward low frequency from 39.60 to 29.46 GHz with the increase in Ti4+ content from x = 0.2 to 0.8. It is known that the natural resonance frequency (fr) is proportional to the anisotropy field (Ha) as expressed by the following equation [Citation33]:
2
where γ is the gyromagnetic ratio. The value of γ/2π is 1.4g GHz kOe−1, and g is the Landé factor. Hence, the shift of the natural resonance toward low frequency is contributed by decreasing Ha. The higher the natural resonance frequency, the higher the anisotropy field of the system. The high Ha of the undoped M-type barium ferrite that can be inferred according to the trend of the increase in frequency with decreasing Ti content contributes a higher natural resonance frequency above 40 GHz, which is agreeable with previous reports [Citation34].
Figure 4. Frequency dependence of (a) the real part (ε′) and (b) the imaginary part (ε″) of the relative complex permittivity and (c) the real part (μ′) and (d) the imaginary part (μ″) of the relative complex permeability of BaFe12−xTixO19 samples.
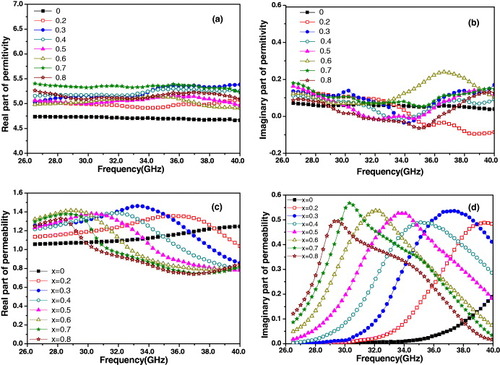
Furthermore, double peaks appear in the magnetic loss μ″ curves of the doped samples as shown in figure (d). The μ″ curves can be separated into two single peaks mathematically as illustrated in figure , which shows the samples of BaFe11.4Ti0.6O19, BaFe11.3Ti0.7O19 and BaFe11.2Ti0.8O19, respectively. It is clear that the intensity of the resonance peak at high frequency is relatively increased with the increase in Ti4+ content. The frequencies of the two peaks for the BaFe11.4Ti0.6O19, BaFe11.3Ti0.7O19 and BaFe11.2Ti0.8O19 samples are (31.69 GHz, 36.08 GHz), (30.25 GHz, 34.40 GHz) and (29.39 GHz, 33.53 GHz), respectively. As is known, the Landé factor g for Fe3+ ions is 2.0 and it is around 3.4 for localized Fe2+ ions [Citation20, Citation35], while the exchange coupling between Fe3+ and Fe2+ gives a g factor that is greater than 2.0 [Citation20]. According to equation (Equation22 ), the two values of g can be obtained based on the resonance frequency revealed by the two peaks. They are 2.02 and 2.31 for BaFe11.4Ti0.6O19 and 2.03 and 2.32 for BaFe11.2Ti0.8O19, respectively. Furthermore, the same amount of Fe3+ ions in the phase structure will change into Fe2+ ions, with Ti4+ ions substituting the Fe3+ ions in order to maintain charge balance. The exchange coupling between Fe2+ ions and Fe3+ ions is thus generated in the ferrite with the g value of about 2.3. Obviously, the peaks at low and high frequencies can be ascribed to the Fe3+ ions and the exchange coupling between Fe3+ and Fe2+, respectively. The increase in the intensity of the high-frequency peak with dopant can be therefore attributed to the increase in Fe2+ ions and the appearance of exchange coupling between Fe3+ and Fe2+. What is more interesting is that the double resonance peaks contribute actually two magnetic loss peaks in the ferrite within a broad frequency range. It may make an effective contribution to the improvement of EM wave absorption properties, and therefore the BFTO will be attractive for use as a high-quality material for EM wave absorption.
Figure 5. Separating curves of the natural resonance peaks from experimental data for (a) BaFe11.4Ti0.6O19, (b) BaFe11.3Ti0.7O19 and (c) BaFe11.2Ti0.8O19. The dotted lines are experimental data; the solid lines are fitted data.
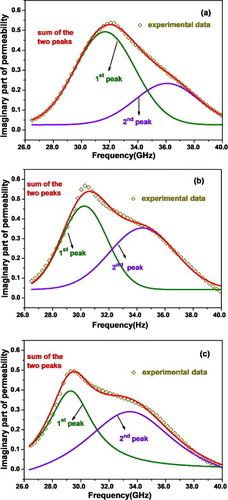
Figure (a) shows the reflection loss (RL) of the BFTO samples as a function of frequency over the range from 26.5 to 40 GHz. It is seen that the two peaks of the RL whose intensity is high up to ∼40 dB or above appear especially for samples with high Ti content in the BFTO, such as x from 0.6 to 0.8. The lower the content of Ti doping, the higher the absorbing peak frequency. As is known, RL is usually used to characterize the microwave absorbing property of materials, which can be calculated based on the complex permittivity and permeability according to transmission line theory by the expressions below [Citation6, Citation36]:
3
4
where Zin is the input impedance at the absorber surface, Z0 is the characteristic impedance in free space, εr and μr are the complex permittivity (εr = ε′–jε″) and permeability (μr = μ′–jμ″), respectively, f is the microwave frequency, d is the thickness of the absorber and c is the velocity of light. The peak indicates that the absorption property strongly occurs with high absorption intensity at a characteristic frequency. For as-prepared BFTO, the high intensity depicts that its absorbing property is quite strong at different frequencies for different samples, in which all the curves shown in figure (a) are obtained with the appropriate thickness needed according to equations (Equation3
3 ) and (Equation4
4 ). Figure (b) illustrates the two RL curves separately calculated by using the data resulting from the two separated resonance peaks, respectively, as shown in figure for BaFe11.4Ti0.6O19, BaFe11.3Ti0.7O19 and BaFe11.2Ti0.8O19. They indicate apparently that the single resonance peak contributes only one absorption peak and double peaks provide dual peaks correspondingly. The absorption peak at low frequency depends on the resonance peak at low frequency with the Landé factor g = 2 and that at high frequency is matched with the resonance peak at relatively high frequency with the Landé factor g above 2.3. Therefore, the peak frequency decreases gradually from high to low following the resonance peak along with the increase in Ti content as shown in figure (d), in which double absorption peaks begin to appear typically at x = 0.6 while the intensity of the high-frequency peak gradually increases.
Figure 6. RL of (a) BaFe12−xTixO19 with x from 0 to 0.8 and with appropriate matching thickness calculated by referring to μ″ with relative resonance peaks and (b) that with x values of 0.6, 0.7 and 0.8 calculated by referring to only the first or second resonance peak, respectively.
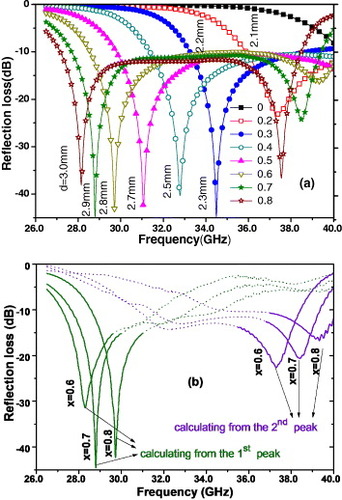
The strong absorption seems to be attributed to the high attenuation constant appearing in the Ti-doped ferrites. As is known, the attenuation constant α is calculated as below [Citation37]:
5
where f is the EM wave frequency and c is the velocity of light. The frequency dependence of the attenuation constant of the BFTO is shown in figure . It is obvious that all the ferrite samples with different Ti4+ contents have a high attenuation constant throughout the test frequency range of 26.5–40 GHz. Meanwhile, their attenuation constants at peak positions distribute between 350 and 500, in which the highest one is 3–10 times higher than that reported by other researchers [Citation37, Citation38]. Actually, the attenuation constant shows almost the same trend with frequency as that of the magnetic loss shown in figure (d) and is associated with the absorption properties as shown in figure (a); for instance, they exhibit two characteristic peaks in the ferrites with x above 0.6. It is thus most probably dominated by the permittivity and permeability as shown in equation (Equation5
5 ). The absorption peak appears hence following the peak occurring in the attenuation constant. Obviously, the magnetic and dielectric losses can promote the EM wave attenuation, and the magnetic loss peak obtained around the frequency of the natural resonance contributes to the outstanding absorbing properties of the BFTO.
Other than the strong absorption at the characteristic frequency of the absorption peak, the BFTO with high Ti content especially from x = 0.5 to 0.8 shows broad bandwidths with an RL of less than −10 dB over the frequency range beyond 11 GHz. As is known, an absorber is thought to be practicably and acceptably used as an absorbing material when its RL is less than −10 dB, which is equal to 90% attenuation corresponding to the power of the incident radiation into the absorber. The RLs of the BFTO are obviously less than −10 dB over the frequency range between the two absorption peaks. This implies that the BFTO with two types of natural resonance as well as strong peaks of magnetic loss makes the absorption bandwidth broader than 11 GHz. Take the BaFe12−xTixO19 ferrite for example; the bandwidths are 11.44, 11.73 and 11.32 GHz at x = 0.6, 0.7 and 0.8, respectively. That is to say, both the resonances of magnetic moments based on Fe3+ and the exchange coupling between Fe3+ and Fe2+ ions simultaneously contribute to the magnetic loss for EM wave absorption in Ti-doped ferrites. The absorption peak based on the exchange coupling between Fe3+ and Fe2+ ions increases gradually and the dual peaks are typically generated with increasing Ti above x = 0.6 as shown in figure (a). The broadband absorbing property appears thus in the BFTO ferrites in which the strongest microwave absorption property around −45 dB of RL and 11 GHz of practicable bandwidth are obtained with x varying from 0.5 to 0.8, making them attractive candidates for use as absorbing materials with extraordinary properties.
4. Conclusion
In this paper, the single-phased Ti-doped barium ferrites (BFTO) with typical hexagonal plate-like structure were synthesized successfully by the sol–gel method. The Ti4+ ions doped into the ferrite substitute for the Fe3+ ions in the sites of 2b in the magnetoplumbite phase structure of BFTO. Some unsubstituted Fe3+ ions in the phase structure change into Fe2+ ions in order to maintain the charge balance in the ferrite, resulting in two kinds of resonances of magnetic moments at relatively low and high frequency based on Fe3+ ions with Landé factor g ∼ 2.0 and on the exchange coupling between Fe3+ and Fe2+ with g ∼ 2.3, respectively. Simultaneously, the resonances supply dual magnetic loss peaks, promoting the EM wave attenuation of Ti-doped ferrites. The high attenuation constant with dual resonance peaks dominates beam absorption, contributing strong double RL peaks as well as considerably broad bandwidth in absorbing frequency. The BFTO ferrites can be used as excellent microwave absorbing materials with strong absorption and broad bandwidths in the frequency range between 26.5 and 40 GHz. The optimal RL reaches around −45 dB and the practicable bandwidth is beyond 11 GHz.
Acknowledgments
This work was supported by the Natural Science Foundation of China under grant numbers 51272230 and 50872120, the Zhejiang Provincial Natural Science Foundation (grant number Z4110040) and the National Key Scientific and Technological Project of China (grant number 2009CB623302).
References
- CaoJet al 2009 J. Phys. Chem. B 113 4642 4647 4642–7 10.1021/jp8093287
- LiuX GGengD YMengHShangP JZhangZ D 2008 Appl. Phys. Lett. 92 173117 10.1063/1.2919098
- LiGXieT SYangS LJinJ HJiangJ M 2012 J. Phys. Chem. C 116 9196 9201 9196–201 10.1021/jp300050u
- CuiC KDuY CLiT HZhengX YWangX HHanX JXuP 2012 J. Phys. Chem. B 116 9523 9531 9523–31 10.1021/jp3024099
- ChenQDuP YHuangW YJinLWengW JHanG R 2007 Appl. Phys. Lett. 90 132907 10.1063/1.2718482
- SunG BDongB XCaoM HWeiB QHuC W 2011 Chem. Mater. 23 1587 1593 1587–93 10.1021/cm103441u
- OhkoshiSKurokiSSakuraiSMatsumotoKSatoKSasakiS 2007 Angew. Chem. Int. Ed. Engl. 46 8392 8395 8392–5 10.1002/anie.200703010
- ZhengHDongY LWangXWengW JHanG RMaNDuP Y 2009 Angew. Chem. Int. Ed. Engl. 48 8927 8930 8927–30 10.1002/anie.200904269
- MaliAAtaieA 2005 Scr. Mater. 53 1065 1070 1065–70 10.1016/j.scriptamat.2005.06.037
- XuPHanX JJiangJ JWangX HLiX DWenA H J 2007 Phys. Chem. C 111 12603 12608 12603–8 10.1021/jp073872x
- QiuJ XGuM YShenH G 2005 J. Magn. Magn. Mater. 295 263 268 263–8 10.1016/j.jmmm.2005.01.018
- DuLDuY CLiYWangJ YWangCWangX HXuPHanX J 2010 J. Phys. Chem. C 114 19600 19606 19600–6 10.1021/jp1067268
- SinghCBindra-NarangSHudiaraI SSudheendranKRajuK C J 2011 J. Electroceram. 27 120 125 120–5 10.1007/s10832-011-9656-5
- ZhangX HZhuLDongY LWengW JHanG RMaNDuP Y 2010 J. Mater. Chem. 20 10856 10861 10856–61 10.1039/c0jm01506g
- BrabersV A MStevensA A EDaldcropJ H JŠimšaZ 1999 J. Magn. Magn. Mater. 196–197 312 313 312–3 10.1016/S0304-8853(98)00730-6
- QuirozPHalbedelBBustamanteAGonzálezJ C 2011 Hyperfine Interact. 202 97 106 97–106 10.1007/s10751-011-0361-1
- HuangJ QDuP YHongL XDongY LHongM C 2007 Adv. Mater. 19 437 440 437–40 10.1002/adma.200601217
- CanM MCoşkunMFıratT 2011 J. Nanopart. Res. 13 5497 5505 5497–505 10.1007/s11051-011-0537-2
- SastryM DNagarY CBhushanBMishraK PBalaramVSinghviA K 2008 J. Phys.: Condens. Matter. 20 025224 10.1088/0953-8984/20/02/025224
- CanM MCoşkunMFıratT 2012 J. Alloys Compounds 542 241 247 241–7 10.1016/j.jallcom.2012.07.091
- DongY LDuP YWengW JHanG RZhaoG L 2008 J. Electroceram. 21 327 330 327–30 10.1007/s10832-007-9160-0
- ZhuLDongY LZhangX HYaoY YWengW JHanG RMaNDuP Y 2012 J. Alloys Compounds 503 426 430 426–30 10.1016/j.jallcom.2010.05.025
- MeazT MKochC B 2004 Hyperfine Interact. 156/157 341 346 341–6 10.1023/B:HYPE.0000043251.88839.a1
- HanM GOuYChenW BDengL J 2009 J. Alloys Compounds 474 185 189 185–9 10.1016/j.jallcom.2008.06.047
- SinghCBindra-NarangSHudiaraI SBaiY 2008 J. Alloys Compounds 464 429 433 429–33 10.1016/j.jallcom.2007.10.009
- Mendoza-SuárezGRivas-VázquezL PCorral-HuacuzJ CFuentesA FEscalante-GarcíaJ I 2003 Physica B 339 110 118 110–8 10.1016/j.physb.2003.08.120
- KreiselJVincentHTassetFPatéMGanneJ P 2001 J. Magn. Magn. Mater. 224 17 29 17–29 10.1016/S0304-8853(00)01355-X
- ShamsM HSalehiS M AGhasemiA 2008 Mater. Lett. 62 1731 1733 1731–3 10.1016/j.matlet.2007.09.073
- DhoJLeeE KParkJ YHurN H 2005 J. Magn. Magn. Mater. 285 164 168 164–8 10.1016/j.jmmm.2004.07.033
- YanL GWangJ BHanX HRenYLiuQ FLiF S 2010 Nanotechnology 21 095708 10.1088/0957-4484/21/9/095708
- XiLWangZZuoY LShiX N 2011 Nanotechnology 22 045707 10.1088/0957-4484/22/4/045707
- LiuX GLiBGengD YCuiW BYangFXieZ GKangD JZhangZ D 2009 Carbon 47 470 474 470–4 10.1016/j.carbon.2008.10.028
- GairolaS PVermaVSinghAPurohitL PKotnalaR K 2010 Solid State Commun. 150 147 151 147–51 10.1016/j.ssc.2009.10.011
- PullarR CAppletonS GBhattacharyaA K 1998 J. Magn. Magn. Mater. 186 326 332 326–32 10.1016/S0304-8853(98)00107-3
- TovarMCausaM TButeraANavarroJMartínezBFontcubertaJPasseggiM C G 2002 Phys. Rev. B 66 024409 10.1103/PhysRevB.66.024409
- CheR CPengL MDuanX FChenQLiangX L 2004 Adv. Mater. 16 401 405 401–5 10.1002/adma.200306460
- WenF SZhangFLiuZ Y 2011 J. Phys. Chem. C 115 14025 14030 14025–30 10.1021/jp202078p
- LuBHuangHDongX LZhangX FLeiJ PSunJ PDongC 2008 J. Appl. Phys. 104 114313 10.1063/1.3040006