Abstract
The voice is produced by the vibration of vocal cords which are located in the larynx. Therefore, one of the major consequences for patients subjected to laryngectomy is losing their voice. In these cases, a synthetic one-way valve set (voice prosthesis) can be implanted in order to allow restoration of speech. Most voice prostheses are produced with silicone-based materials such as polydimethylsiloxane (PDMS). This material has excellent properties, such as optical transparency, chemical and biological inertness, non-toxicity, permeability to gases and excellent mechanical resistance that are fundamental for its application in the biomedical field. However, PDMS is very hydrophobic and this property causes protein adsorption which is followed by microbial adhesion and biofilm formation. To overcome these problems, surface modification of materials has been proposed in this study. A commercial silicone elastomer, SylgardTM 184 was used to prepare membranes whose surface was modified by grafting 2-hydroxyethylmethacrylate and methacrylic acid by low-pressure plasma treatment. The hydrophilicity, hydrophobic recovery and surface energy of the produced materials were determined. Furthermore, the cytotoxicity and antibacterial activity of the materials were also assessed. The results obtained revealed that the PDMS surface modification performed did not affect the material's biocompatibility, but decreased their hydrophobic character and bacterial adhesion and growth on its surface.
1. Introduction
One of the basic human attributes is the voice. This phenomenon is produced through a simple mechanical setup [Citation1], i.e. muscular contractions within the chest that force the air to pass from the lungs through several components of the vocal mechanism during expiration. This stream of air is the energy carrier that is then modulated in its velocity and pressure to produce sounds [Citation2]. The larynx plays a key role in this process, since the voice cords are stretched across it and have the shape of membranes, forming a diaphragm. The air flow through this diaphragm causes vibrations on the cords generating the voice [Citation3]. Therefore, it is not surprising that one of the main issues of a total laryngectomy (surgical ablation of the larynx due to extensive cancer) is loss of the voice [Citation4]. Rehabilitation of these patients has become a major challenge and only in the last three decades has speech restoration been achieved in order to improve the patient's quality of life [Citation5]. Nowadays, the common procedure used for this purpose is comprised of the insertion of a silicone rubber voice prosthesis based on polydimethylsiloxane (PDMS) in a surgically created tracheoesophageal fistula in the patient [Citation6]. There are two main types of prosthesis available, the non-indwelling (caregiver) that can be changed independently by the patient and the indwelling prosthesis that must be changed by a clinician [Citation6]. Commonly, patients who are laryngectomized prefer the use of indwelling voice prosthesis, since they find the handling of the prosthesis uncomfortable and distressing.
Non-indwelling devices are removed on a daily basis, in order to keep them clean. However, indwelling prosthesis are meant to remain implanted for a long period of time [Citation7], and one of the main problems associated with this type of prosthesis is biofilm formation, which eventually leads to dysfunction and the need for device replacement [Citation8]. The high hydrophobicity of PDMSs has been pointed to as the main cause of adsorption of significant amounts of proteins from the surrounding biological environment, followed by microbial adhesion and biofilm formation [Citation9]. Several approaches have been used in order to reduce biofilm formation over PDMS [Citation10], among them, coating it with various compounds such as poly(carboxybetaine methacrylate) [Citation11], polyacrylamide [Citation12] or metals [Citation13]; or grafting hydrophilic monomers by laser [Citation14], plasma [Citation15] or chemical treatment [Citation10].
Herein, membranes based on a commercial PDMS (Sylgard™ 184) were plasma surface modified by separately grafting 2-hydroxyethilmethacrylate (HEMA) and methacrylic acid (MAA) onto its surface in order to have suitable properties for application in the envisioned biomedical application.
The properties of the modified membranes were then characterized in order to evaluate their potential for being used in the near future in the production of voice prostheses. Several parameters were assessed such as their antibacterial activity, cytotoxicity, hydrophilicity, hydrophobic recovery and surface energy.
2. Experimental
2.1. Materials
Bacterial strain Escherichia coli (E. coli) DH5α was purchased from ATCC. Fetal bovine serum (FBS) was acquired from Biochrom AG (Berlin, Germany). SylgardTM184 PDMS kit containing two parts, a liquid silicone rubber base and a curing agent, was obtained from Dow Corning. Human fibroblast cells (normal human dermal fibroblasts adult, cryopreserved cells) were bought from PromoCell (Labclinics, S.A.; Barcelona, Spain). Lysogeny broth agar was acquired from Pronadise. 3-(4,5-dimethylthiazol-2-yl)-5-(3carboxymethoxyphenyl)-2- (4-sulfofenyl)-2H-tetrazolium, inner salt (MTS), amphotericin B, Dulbecco's modified eagle's medium (DMEM-F12), ethylenediaminetetraacetic acid (EDTA), HEMA, L-glutamine, MAA, penicillin G, phosphate-buffered saline solution (PBS), streptomycin, trypan blue and trypsin were purchased from Sigma-Aldrich (Sintra, Portugal).
2.2. Methods
2.2.1. Membrane preparation.
PDMS pre-polymer and curing agent were mixed at a mass ratio of 10:1, stirred thoroughly, and then degassed under vacuum. The mixture was poured into Petri dishes (0.5 mm of thickness) and cured in an oven, at 65 °C for 4 h. Subsequently, the membranes were removed from the dishes and washed thoroughly with acetone.
2.2.2. Plasma grafting.
Plasma surface modification experiments were carried out with a small-scale production plasma system manufactured by Diener Electronics, with a stainless steel plasma chamber of 100 mm diameter and 270 mm length. The PDMS membranes were placed at 80 mm from the electrode and then treated with argon plasma, at 0.6 mbar for 3 min, applying 100 W of power to the electrodes to generate the plasma [Citation15]. Then, as schematically shown in figure , the plasma-treated PDMS membranes were dipped into a 10% (v/v) aqueous solution of either HEMA or MAA and then introduced in an oven at 60 °C, for 8 h. Finally, the membranes were washed thoroughly with deionized water and dried until they achieved constant weight. The modified membranes, HEMA-grafted PDMS (PDMS–HEMA) and MAA-grafted PDMS (PDMS–MAA) were then obtained.
2.3. Characterization techniques
2.3.1. ATR–FTIR spectroscopy.
Attenuated total reflectance (ATR)–Fourier transform infrared (ATR–FTIR) analyses were performed with a Magma-IRTM Spectrometer 750 from Nicolet, equipped with a Golden Gate Single Reflection Diamond ATR attachment. Spectra were averaged over 128 scans at a resolution of 4 cm−1.
2.3.2. Contact angle and surface free energy.
The contact angle and surface free energy measurements were performed at room temperature in an OCA 20 contact angle measurement unit from Dataphysics. The water contact angle was evaluated by static contact angle measurements using the sessile drop method. Surface free energy (γ S) values as well as the dispersive (γ SD) and polar (γ SP) components were obtained according to the Owens–Wendt–Rabel–Kaelbe method (OWRK) by static contact angle measurements with three liquids: water, diiodomethane and formamide. All measurements were performed on the air-facing surfaces of the samples with the three liquids using the sessile drop method. Nine measurements on different points were performed on each sample, from which the mean static contact angle and its standard deviation were determined. The surface energies were assessed for all the prepared membranes.
2.3.3. Hydrophobicity recovery.
Samples were stored in vials containing milli-Q water or PBS at 37 °C and examined after 1, 2, 7, 15 and 30 days. Samples aged in air were wrapped in aluminum foil to minimize hydrocarbon contamination, and those aged in milli-Q water and PBS were thoroughly washed with milli-Q water and dried before analysis. The hydrophobicity recovery was evaluated by water contact angle determination as previously described in section 2.3.1.
2.3.4. X-ray diffraction.
X-ray powder diffraction analysis was used to determine the physical form (amorphous or crystalline) of the different PDMS membranes. The experiments were performed over the range of 2θ from 1° to 100°, using a Rigaku Geiger Flex D-max III/c diffractometer (Rigaku Americas Corporation, Texas, USA) with a copper ray tube operated at 30 kV and 20 mA. The membranes were mounted on silica supports using a double-sided adhesive tape and then analyzed.
2.3.5. Scanning electron microscopy analysis.
The materials morphology with/without human fibroblast cells were analyzed by scanning electron microscopy (SEM). To evaluate cell adhesion and proliferation, human fibroblast cells were seeded over the materials. After one day of culture, the samples were fixed overnight with 2.5% glutaraldehyde in PBS, at 4 °C. Hereafter, samples were rinsed three times with distilled water for 2 min and dehydrated in graded ethanol of 70, 80, 90 and 100% for 10 min in each solution [Citation16, Citation17]. Subsequently, the materials were mounted on stubs using a double-side adhesive tape and sputter coated with gold using an Emitech K550 sputter coater (London, UK). SEM images were acquired with a scanning electron microscope Hitachi S-2700 (Tokyo, Japan) at an acceleration voltage of 20 kV and different magnifications [Citation18].
2.3.6. Characterization of the biocompatibility of the materials.
Proliferation of cells in the presence of the samples. Human fibroblast cells were seeded in a T-25 flask with 6 ml of DMEM-F12 supplemented with heat-inactivated FBS (10% v/v) and 1% antibiotic/antimycotic solution. After the cells become confluent, they were subcultivated by a 3–5 min incubation in 0.18% trypsin (1:250) and 5 mM EDTA. Subsequently, cells were centrifuged, resuspended in culture medium and then seeded in T-flasks with a surface area of 75 cm2. Hereafter, cells were kept in culture at 37 °C in a 5% CO2 humidified atmosphere, inside an incubator. To evaluate cell behavior in the presence of the materials, human fibroblast cells were seeded with materials in 96-well plates at a density of 1 × 104 cells per well, for 96 h. The materials were sterilized by UV irradiation for 30 min before being placed in contact with cells. Cell growth was monitored using an Olympus CX41 inverted light microscope (Tokyo, Japan) equipped with an Olympus SP-500 UZ digital camera [Citation17, Citation19]. A trypan blue exclusion test was used to determine the number of viable cells on each material. Briefly, a mixture of 90 μl of 0.4% trypan blue and 10 μl of cell suspension, from each well, was used. Then, the number of unstained (viable) cells was counted with a hemacytometer.
Characterization of the cytotoxic profile of the membranes. Human fibroblast cells were seeded in the presence of materials, in 96-well plate, with 100 μl of DMEM-F12 and then incubated at 37 °C, in a 5% CO2 humidified atmosphere. After an incubation period (24, 48 and 72 h), cell viability was assessed through the reduction of the MTS into a water-soluble formazan product. Briefly, the medium of each well was removed and replaced with a mixture of 100 μl of fresh culture medium and 20 μl of MTS/PMS reagent solution. Then, cells were incubated for 4 h at 37 °C, under a 5% CO2 humidified atmosphere. The absorbance was measured at 492 nm using a microplate reader (Sanofi, Diagnostics Pauster). Wells containing cells in the culture medium without materials were used as negative controls (K −). Ethanol (96%) was added to wells that contained cells, as a positive control (K +) [Citation16, Citation17, Citation20].
2.3.7. Characterization of the antibacterial activity of PDMS materials.
To evaluate biofilm formation on the three PDMS material surfaces, E. coli were seeded over them at 1 × 108 CFU ml−1 in an agar plate. After 24 h, the materials were prepared for SEM analysis as described in section 2.3.4 [Citation21].
2.3.8. Statistical analysis.
The one-way (one factor) ANOVA test and post-hoc test (Bonferroni–Holm) were used to analyze the difference of the data collected at different time points [Citation22]. For all statistical tests, a p value 0.05 was considered statistically significant.
3. Results and discussion
3.1. Surface modification and hydrophilicity evaluation
PDMS surface was modified by grafting with HEMA or MAA. ATR–FTIR was used to characterize both unmodified and grafted PDMS in order to identify typical chemical groups attributed to the grafted monomers. Infrared spectroscopy, usually in the ATR mode, has been often used to detect the grafting of molecules to surfaces by plasma treatment of PDMS [Citation23–Citation25]. Figure shows the ATR–FTIR spectra obtained for all samples. Comparison of the original PDMS with the modified surfaces indicates the presence of both monomers on the PDMS surface. In PDMS–HEMA, the characteristic absorption bands of the monomer appear at 3590 cm−1 (hydroxyl groups) and at 1743 and 1249 cm−1 (ester groups) [Citation23]. Also, in PDMS–MAA, the monomer main bands are visible. Hydroxyl groups of MAA are responsible for the band at 3290 cm−1 while the bands at 1729 and 1256 cm−1 are attributed to C = O and C–O stretching of the carboxylic groups, respectively [Citation24].
Hydrophilicity of the materials was determined by contact angle measurement and posterior surface energy determination. It is widely recognized that surface energy is an important parameter affecting protein adhesion on polymers' surface, material wettability and even biocompatibility [Citation26]. The measurement of contact angles is considered as the most convenient method for determining the surface free energy of solid samples. This technique relies on the determination of the interactions between the solid sample of interest and liquids with well determined surface tensions.
According to the OWRK method, the interfacial tension can be divided into two components: dispersive and polar interactions [Citation27, Citation28]. Polar interactions comprise Coulomb interactions between permanent dipoles and the ones between permanent and induced dipoles. The interactions caused by time fluctuations of the charge distribution within the molecules are called dispersive interactions.
Table summarizes the obtained results for these parameters as well as the percentage of the polar component of the surface energy for the original and modified PDMS membranes.
Table 1. Values of water contact angles and surface free energy (γ S), dispersive (γ SD) and polar components (γ SP) of the surface free of the original modified PDMS membranes.
From table , it can be seen that the untreated PDMS membrane is very hydrophobic (contact angle for water at 105.5°) and the surface energy of this membrane is mainly controlled by the dispersive component. These results are explained by the high Si–O bond energy that makes PDMS an excellent water repellent material resulting from their weak intermolecular interactions [Citation29]. However, water contact angle significantly decreases when monomers are grafted by plasma surface activation. For PDMS–HEMA and PDMS–MAA membranes, the water contact angles decreased to 60.6° and 56.3°, respectively. Also, while the original PDMS membrane presents a polar component of 0.6%, this value changed to 25.6% for PDMS–HEMA and to 28.3% for PDMS–MAA. The presence of polar functional groups such as OH, NH2 or COOH increases the hydrogen bonding interactions and therefore increases the polar component of the surface energy. In this study, grafting HEMA (which contains OH groups) and MAA (containing carboxylic groups) onto the membranes increased the polar component of the surface energy. These results confirm that the membranes treated with plasma present higher surface polarity independently of the monomer used, which shows that the surface modifications were successful.
3.2. Hydrophobic recovery analysis
Hydrophobicity recovery of PDMS has been explained as the result of the reorientation of surface silanol groups into the bulk polymer and cracking of the SiOx structure. As a consequence, a series of rearrangements occurs, such as free PDMS chains’ migration from the bulk to the surface, condensation of silanol groups at the surface, loss of volatile oxygen or other species into the atmosphere and changes in surface roughness [Citation30–Citation32]. In order to try to control this issue, hydrophobic recovery was evaluated by static water contact angle measurements for a period of 30 days and storage in three different environments (air, milli-Q water and PBS). The obtained results are shown in figure . PDMS bare membranes showed no significant differences with time for all studied environments, and for this reason these results are not shown in figure . As expected, when both surface modified membranes were stored in air, an increase of hydrophobicity of 28% for MAA and 55% for HEMA was registered. However, when comparing the contact angles of samples stored in water with those stored in PBS, it is clearly visible that PBS is more effective in maintaining the hydrophilic character than water. This fact is more evident when HEMA is used for grafting, which suggests that the changes in water contact angle for PDMS–HEMA samples are mainly due to the reorientation of HEMA's functional groups on the surface of PDMS [Citation33]. Nevertheless, it can be assumed that despite the PDMS surface reorientation of groups, HEMA and MAA form a permanent layer on the surface and allow a greater hydrophilic stability of the membranes, even under ambient atmosphere.
3.3. X-ray diffraction
In order to determine the microstructure of PDMS and its derivatives, the samples were analyzed by x-ray diffraction (XRD). As illustrated in figure , all the diffraction patterns are similar, presenting two diffraction halos: a first and larger one located at around 12.8° and the second, smaller and broader at 22.6°. These results indicate that the microstructure of the samples was amorphous and that grafting did not change the bulk properties of the base material. Similar XRD profiles were previously obtained for PDMS and copolymers of PDMS with polyurethanes by other authors [Citation34].
3.4. Characterization of materials' biocompatibility
In vitro studies were performed seeding human fibroblast cells with the same initial density in the 96-well plates, with or without materials to characterize biocompatibility samples. A trypan blue exclusion test was used to determine the number of viable cells (figure ). Cell adhesion and proliferation in the presence of the materials was characterized through an inverted optical microscope (figure ) and SEM analysis (figure ).
Figure 5. Optical images of human fibroblast cells seeded in the presence of the different PDMS materials (∗) after 24, 48 and 72 h of incubation (A). Cell number per well in the presence of each material at 24, 48 and 72 h (B) K − , negative control; K + , positive control. All images were acquired with the same original magnification.
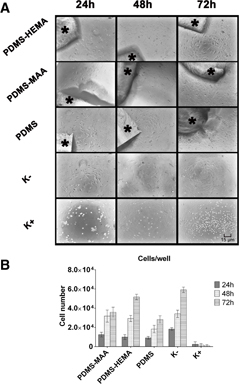
Figure 6. SEM images of PDMS materials surface without (left column) and with adhered cells (right column): PDMS–MAA (a) and (b); PDMS–HEMA (c) and (d); PDMS (e) and (f).
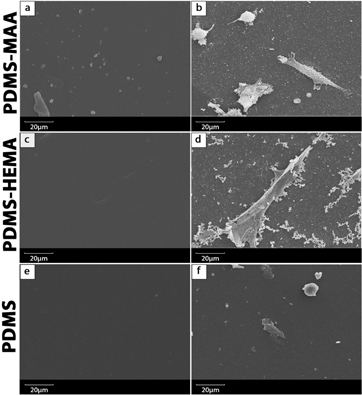
Both figures show that cells adhered and proliferated in contact with all the materials and in the negative control (figure ). However, due to the hydrophobic character of PDMS, a much lower number of cells adhered to its surface.
As previously described in the literature, the chemical groups exposed onto surface materials dictate their wettability and also influence protein adsorption to the materials’ surface [Citation35]. PDMS surfaces were modified with MAA and HEMA monomers. These plasma-assisted modifications improved the hydrophilic character of the materials, as confirmed by contact angles (table ). In this study, the functionalization of PDMS materials with HEMA contributed to the increase in the number of hydroxyl groups (–OH) present in the sample. Meanwhile, materials treated with MAA contained more carboxyl groups (–COOH). The presence of groups rich in oxygen, such as –COOH and –OH, on materials' surfaces may contribute to the increase in wettability.
Protein adsorption to materials’ surfaces is dependent on the hydrophobic character of materials. It has been previously described that surfaces with moderate hydrophilicity are considered the most effective for optimal protein adsorption [Citation35]. The modified materials presented contact angles between 40° and 70°, which are in the range of moderately hydrophilic materials [Citation36–Citation38]. Such results clearly demonstrate the suitability of materials to allow protein adsorption, in order to allow eukaryotic cell adhesion and proliferation as demonstrated by our experimental data [Citation35].
In order to characterize the physiological response of the fibroblasts to the presence of these materials, an MTS assay was performed. The MTS assay results (figure ) showed that cells in the presence of the membranes had higher viability than in the positive control. The results obtained show that none of the membranes affected cell integrity or viability, which is fundamental for the biomedical applications proposed for these materials.
Figure 7. Evaluation of the cellular activity after 24, 48 and 72 h. PDMS–MAA; PDMS–HEMA; positive control (K +); negative control (K −). Each result is the mean ± standard error of the mean of three independent experiments. Statistical analysis was performed using one-way ANOVA with Dunnet's post-hoc test (∗p < 0.001).
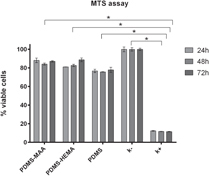
3.5. Antibacterial activity
In order to verify bacteria affinity to the surface of the materials tested, SEM micrographs were acquired (figure ) after a 24 h incubation period. Figure reveals a more pronounced reduction of the bacteria growth in PDMS–MAA membranes when compared to the other membranes.
Figure 8. SEM photographs of E. coli seeded in the presence of the different PDMS materials: (a) PDMS, (b) PDMS–HEMA, and (c) PDMS–MAA.
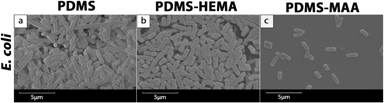
These results indicate that bacterial behavior on the surface is dependent on other factors besides surface energy. When HEMA and MAA are grafted onto the PDMS structure, surface energy increases due to the hydrophilicity of these monomers. Such hydrophilicity is usually associated with a decrease in bacterial adhesion [Citation39]. However, unlike HEMA which presents neutral properties, MAA, being an acidic molecule, contains carboxylic groups. For this reason, PDMS–MAA membrane presents a higher negative charge density than unmodified PDMS or PDMS–HEMA. These charges result from the ability of MAA carboxylic groups to become deprotonated and thus originating negatively charged groups [Citation40]. As a consequence, repulsive electrostatic interactions between PDMS–MAA and bacteria cell wall may prevent the initial bacterial adhesion and biofilm formation is severely reduced [Citation41]. Other authors have reported similar profiles of bacterial adhesion on surfaces modified with HEMA and other acidic monomers [Citation42].
4. Conclusions
HEMA and MAA were grafted onto PDMS surface by a low-pressure Ar plasma treatment leading to a modification of the membranes' native surface properties. Hydrophilicity as well as surface energy of the original PDMS increased with grafting. However, and as expected, bulk properties of the material were not compromised by the surface treatment as verified by XRD analysis.
In vitro studies with human fibroblast cells showed that modification of the surface with hydrophilic monomers did not compromise the biocompatibility of the materials.
More importantly, MAA grafted PDMS surface enhanced the bactericidal activity of the material. This feature is of extreme relevance for the preparation of voice prostheses since bacterial adhesion and biofilm formation are usually the main causes for their dysfunction and replacement.
Based on overall results, we may conclude that modification of PDMS with MAA is an appropriate approach to obtaining a suitable material to prepare voice prostheses that may be used as indwelling implants.
Acknowledgments
The authors thank Eng. Ana Paula for her help with the acquisition of SEM images. This work was supported by the Portuguese Foundation for Science and Technology (FCT), (PTDC/EME-TME/103375/2008 and PTDC/EBB-BIO/114320/2009).
References
- Heman-AckahY D 2005 J. Singing 62 173
- TackJVerkerkeGvan der HouwenEMahieuHSchutteH 2006 Ann. Biomed. Eng. 34 1896 10.1007/s10439-006-9196-3
- SawadaHKitaniMHayashiY 2008 J. Biomed. Biotechnol. 2008 768232 10.1155/2008/768232
- DeshpandeM 2010 Indian J. Surg. Oncol. 1 146 10.1007/s13193-010-0028-4
- SchusterMLohschellerJKummerPHoppeUEysholdtURosanowskiF 2003 Folia Phoniatr. Logo. 55 211 10.1159/000072152
- BalmA Jvan den BrekelM WTanI BHilgersF J 2011 Otolaryngol. Pol. 65 402 10.1016/S0030-6657(11)70731-4
- ChoneC TSpinaA LCrespoA NGrippF M 2005 Braz. J. Otorhinolaryngol. 71 504
- PawarP VSayedS IKaziRJagadeM V 2008 J. Cancer Res. Ther. 4 186 10.4103/0973-1482.44289
- ChenHBrookM ASheardownH 2004 Biomaterials 25 2273 10.1016/j.biomaterials.2003.09.023
- BodasDKhan-MalekC 2006 Microelectron. Eng. 83 1277 10.1016/j.mee.2006.01.195
- KeefeA JBraultN DJiangS 2012 Biomacromolecules 13 1683 10.1021/bm300399s
- FundeanuIvan der MeiH CSchoutenA JBusscherH J 2008 Colloids Surf. B 64 297 10.1016/j.colsurfb.2008.02.005
- Arweiler-HarbeckDSandersAHeldMJermanMEhrichHJahnkeK 2001 Acta Otolaryngol. 121 643 10.1080/000164801316878971
- KhorasaniMMirzadehHSammesP 1999 Radiat. Phys. Chem. 55 685 10.1016/S0969-806X(99)00212-1
- PintoSAlvesPMatosCSantosARodriguesLTeixeiraJGilM 2010 Colloids Surf. B 81 20
- CoimbraPAlvesPValenteTSantosRCorreiaIFerreiraP 2011 Int. J. Biol. Macromol. 49 573 579 573–9 10.1016/j.ijbiomac.2011.06.011
- FonsecaA CCoelhoJ F JValenteJ F ACorreiaT RCorreiaI JGilM HSimõesP N 2013 J. Biomater. Sci. Polym. Ed. 12 1391 1409 1391–409 10.1080/09205063.2012.762293
- GasparVSousaFQueirozJCorreiaI 2011 Nanotechnology 22 015101 10.1088/0957-4484/22/1/015101
- RibeiroM Pet al 2009 Wound Repair Regen. 17 817 10.1111/j.1524-475X.2009.00538.x
- CoimbraPFerreiraPDe SousaHBatistaPRodriguesMCorreiaIGilM 2011 Int. J. Biol. Macromol. 48 112 10.1016/j.ijbiomac.2010.10.006
- TsoligkasA NBowenJWinnMGossR JOvertonT WSimmonsM J 2012 Colloids Surf. B 89 152
- OguchiRDouwstraPFujitaTChowW STerashimaI 2011 New Phytol. 191 146 10.1111/j.1469-8137.2011.03669.x
- AlmutairiZRenC LSimonL 2012 Colloid Surf. A 415 406 10.1016/j.colsurfa.2012.10.008
- KarkhanehAMirzadehHGhaffariyehA R 2007 J. Appl. Polym. Sci. 105 2208 10.1002/app.26216
- WuZTongWJiangWLiuXWangYChenH 2012 Colloids Surf. B 96 37
- KwokS C HWangJChuP K 2005 Diamond Relat. Mater. 14 78 10.1016/j.diamond.2004.07.019
- OwensD KWendtR 1969 J. Appl. Polym. Sci. 13 1741 10.1002/app.1969.070130815
- KaelbleD H 1970 J. Adhes. 2 66 10.1080/0021846708544582
- KimJChaudhuryM KOwenM JOrbeckT 2001 J. Colloid Interface Sci. 244 200 10.1006/jcis.2001.7909
- MakambaHKimJ HLimKParkNHahnJ H 2003 Electrophoresis 24 3607 10.1002/elps.200305627
- SharmaVDhayalMShivaprasadSJainS 2007 Vacuum 81 1094 10.1016/j.vacuum.2007.02.004
- WangPTanKHoCKhewMKangE 2000 Eur. Polym. J. 36 1323 10.1016/S0014-3057(99)00193-7
- BodasDKhan-MalekC 2007 Sensors Actuators B 123 368 10.1016/j.snb.2006.08.037
- BaiCZhangXDaiJWangJ 2008 J. Coat. Technol. Res. 5 251 10.1007/s11998-007-9062-8
- OliveiraS MAlvesN MManoJ F 2012 J. Adhes. Sci. Technol. doi: 10.1080/01694243.2012.697776
- ArimaYIwataH 2007 Biomaterials 28 3074 10.1016/j.biomaterials.2007.03.013
- Van WachemPBeugelingTFeijenJBantjesADetmersJVan AkenW 1985 Biomaterials 6 403 10.1016/0142-9612(85)90101-2
- Van WachemPHogtABeugelingTFeijenJBantjesADetmersJVan AkenW 1987 Biomaterials 8 323 10.1016/0142-9612(87)90001-9
- SellenetP HAllisonBApplegateB MYoungbloodJ P 2007 Biomacromolecules 8 19 10.1021/bm0605513
- EharaAToriiMImazatoSEbisuS 2000 J. Dent. Res. 79 824 10.1177/00220345000790030701
- GottenbosBGrijpmaD WVan Der MeiH CFeijenJBusscherH J 2001 J. Antimicrob. Chemoth. 48 7 10.1093/jac/48.1.7
- KochkodanVHilalNGoncharukVAl-KhatibLLevadnaT 2006 Colloid J. 68 267 10.1134/S1061933X06030021