Abstract
We have prepared multifunctional magnetic mesoporous Fe–CaSiO3 materials using triblock copolymer (P123) as a structure-directing agent. The effects of Fe substitution on the mesoporous structure, in vitro bioactivity, magnetic heating ability and drug delivery property of mesoporous CaSiO3 materials were investigated. Mesoporous Fe–CaSiO3 materials had similar mesoporous channels (5–6 nm) with different Fe substitution. When 5 and 10% Fe were substituted for Ca in mesoporous CaSiO3 materials, mesoporous Fe–CaSiO3 materials still showed good apatite-formation ability and had no cytotoxic effect on osteoblast-like MC3T3-E1 cells evaluated by the elution cell culture assay. On the other hand, mesoporous Fe–CaSiO3 materials could generate heat to raise the temperature of the surrounding environment in an alternating magnetic field due to their superparamagnetic property. When we use gentamicin (GS) as a model drug, mesoporous Fe–CaSiO3 materials release GS in a sustained manner. Therefore, magnetic mesoporous Fe–CaSiO3 materials would be a promising multifunctional platform with bone regeneration, local drug delivery and magnetic hyperthermia.
1. Introduction
Calcium silicate (CaSiO3) materials, a classic example of Ca–Si-based bioceramics, have been proposed as potential bioactive materials for bone tissue regeneration due to their excellent bioactivity and degradability [Citation1–Citation4]. Studies demonstrated that biomaterials with high specific surface area and pore volume could accelerate the kinetic process of apatite formation and therefore, enhance the bone-forming bioactivity [Citation5, Citation6]. In recent years, many efforts have been made to develop mesoporous CaSiO3 materials for bone regeneration [Citation7–Citation12], because mesoporous materials have unique structural characteristics including high specific surface area, large pore volume and controllable porosity at mesoscale [Citation13]. Li et al [Citation7] synthesized mesoporous CaSiO3 materials using mesoporous silica SBA-15 as the template and silicon source, which exhibited a significantly enhanced bone-forming ability compared to the conventional amorphous CaSiO3 materials. Chang and co-workers [Citation8, Citation9] prepared mesoporous CaSiO3 materials using the surfactant templating method to study its potential application in filling the apical root canals of teeth. In addition, Wei et al [Citation10] and Zhu et al [Citation11] designed mesoporous CaSiO3/polymer composites for potential use in hard tissue repair. On the other hand, mesoporous materials have been considered as promising carriers for drug delivery [Citation13, Citation14], and mesoporous CaSiO3 nanoparticles could also exhibit sustained drug delivery ability [Citation12]. Therefore, mesoporous CaSiO3 materials with local drug delivery would be beneficial for bone regeneration.
Generally, both texture and composition of bioceramics control their physicochemical and biological properties [Citation15–Citation17]. Studies demonstrated that the incorporation of zinc (Zn), strontium (Sr), titanium (Ti) or magnesium (Mg) in CaSiO3 ceramics improved their physicochemical and biological properties [Citation18–Citation24]. For example, Ramaswamy et al [Citation19] incorporated Zn into Ca–Si system to form Ca2ZnSi2O7 ceramics, and found that Ca2ZnSi2O7 ceramics supported osteoblast-like cells attachment with a well-organized cytoskeleton structure and significantly increased cellular proliferation and differentiation compared to CaSiO3 ceramics. Lu et al [Citation23] synthesized a mesoporous magnesium–CaSiO3, which could support cell attachment and promote the proliferation and differentiation of MC3T3-E1 cells. We recently substituted Sr into mesoporous CaSiO3 materials, and found that the mesoporous Sr–CaSiO3 materials kept mesoporous structure and enhanced the proliferation and alkaline phosphatase activity of MC3T3-E1 cells compared to the mesoporous CaSiO3 materials [Citation24].
Iron (Fe) plays a vital role in the functioning of body with Fe pool in humans being found in the red blood cells, with a lesser extent in the tissues and a small amount circulating in the plasma [Citation25]. Studies demonstrated that the Fe-containing bioceramics could stimulate their cell response ability [Citation26–Citation29]. For example, Wu et al [Citation26] fabricated a CaP ceramic–magnetite nanoparticles (CaP–MNP) composite, and the in vitro results indicated that the CaP–MNP composite was able to significantly promote Ros17/2.8 and MG63 cells’ proliferation and differentiation compared to ordinary CaP ceramics. Panseri et al developed magnetic hydroxyapatite scaffolds to enhance tissue regeneration. The hydroxyapatite/magnetite 90/10 scaffolds were shown to enhance cell proliferation at the early stage, and a good level of histocompatibility was observed in a critical size lesion of the rabbit condyle in vivo [Citation27]. On the other hand, studies demonstrated that magnetic bioceramics could generate heat under alternating magnetic field and be used for hyperthermia therapy in bone defects caused by bone tumors [Citation30–Citation33], because cancer cells generally perish around 43 °C due to hemorrhage, stasis and vascular occlusion, whereas normal cells are not damaged until higher temperature [Citation33, Citation34].
Therefore, we hypothesized that mesoporous Fe-doped CaSiO3 (Fe–CaSiO3) materials could induce an improved bone-forming bioactivity and stimulate bone cell growth due to the mesoporous structure and the Fe incorporation. Furthermore, mesoporous Fe–CaSiO3 materials could effectively load drugs or growth factors as a potential local drug delivery system, and could be magnetic seeds for magnetic hyperthermia treatment. It can be believed that mesoporous Fe–CaSiO3 materials would be a promising multifunctional platform with bone regeneration, local drug delivery and magnetic hyperthermia for the treatment of bone defects caused by bone tumors after surgery. Recently, magnetic mesoporous iron oxide and silica particles were developed for drug delivery and hyperthermia therapy, but they were not bioactive for bone regeneration [Citation32, Citation35–Citation37]. We prepared magnetic mesoporous bioactive glass scaffolds, which showed the potential for bone regeneration with bioactivity, sustained drug delivery and magnetic hyperthermia properties [Citation38, Citation39]. However, the preparation procedure was multistep and rather complex. To the best of our knowledge, there are no previous reports describing the preparation of magnetic mesoporous Fe–CaSiO3 materials and further investigating their multifunctionality for bone regeneration.
In this study, we have successfully prepared magnetic mesoporous Fe–CaSiO3 materials using triblock copolymer (P123) as a structure-directing agent. The effects of the Fe substitution on the mesoporous structure, magnetic heating ability and in vitro bioactivity of mesoporous CaSiO3 materials have been investigated. Gentamicin, an antibiotic for treating osteomyelitis, was used as a model drug and introduced into mesoporous Fe–CaSiO3 materials to evaluate the drug delivery property.
2. Experimental methods
2.1. Preparation and characterization of mesoporous Fe–
materials
Mesoporous Fe-–CaSiO3 materials were prepared according to the previously reported method with some modifications [Citation8]. The chemical compositions and the sample names are listed in table . In a typical synthesis for mesoporous 5%Fe–CaSiO3 materials, 3.0 g of P123 (Mw = 5800, Aldrich) was dissolved in 130 ml of H2O and 20.5 ml of HCl ( 37%, Sigma-Aldrich) while stirring at 38 °C in oil bath until the solution became clear. After the addition and dissolution of 9.15 g of Ca(NO3)2·4H2O (Aldrich) and 0.824 g of Fe(NO3)3·9H2O (Aldrich) in P123 solution, 8.5 g of tetraethyl orthosilicate (TEOS, 98%, Aldrich) was then added into the solution. The mixture was stirred at 38 °C for 24 h, and the resulting precipitate was dried at 100 °C for 24 h in air without any filtering and washing. The as-synthesized materials were calcined from room temperature to 600 °C with a heating rate of 1 °C min−1, and maintained at 600 °C for 6 h to remove the templates. Finally, the calcined materials were treated in 10% H2 per 90% Ar at 400 °C for 3 h to obtain magnetic mesoporous Fe–CaSiO3 materials.
Table 1. Chemical composition and the reactants of mesoporous Fe–CaSiO3 materials.
The wide angle x-ray diffraction patterns were obtained on a Stoe Stadi P powder diffractometer equipped with a curved germanium (111) monochromator and linear position-sensitive detector using Cu Kα1 radiation (1.5405 Å) in transmission geometry. Scanning electron microscopy (SEM) was carried out with an FEI Quanta 450 field emission scanning electron microscope. Transmission electron microscopy (TEM) was performed with a JEM-2010 electron microscope operated at an acceleration voltage of 200 kV. N2 adsorption–desorption isotherms were obtained on a Quadrasorb SI automated surface area and pore size analyzer at −196 °C under continuous adsorption condition. Brunauer–Emmett–Teller (BET) and Barrett–Joyner–Halenda (BJH) methods were used to determine the surface area, the pore size distribution and the pore volume. Magnetic measurement was performed using a vibrating sample magnetometer.
2.2. Ion dissolution and apatite formation of mesoporous Fe–
materials in simulated body fluids (SBF)
To investigate the ion dissolution from mesoporous Fe–CaSiO3 materials, the SBF were prepared according to Kokubo’s method [Citation40]. Mesoporous Fe–CaSiO3 materials were soaked in the SBF solutions at 37 °C for 1, 3, 5 and 7 days, and the ratio of mesoporous Sr–CaSiO3 mass to the SBF volume was 2 mg ml−1. The concentrations of the Ca, Si and Fe ions in the SBF solutions were determined by inductively coupled plasma optical emission spectrometer (ICP-OES, Shimadzu ICPS-8100). The pH values of the SBF solutions were measured after soaking mesoporous Fe–CaSiO3 materials at predetermined time intervals.
The apatite formation ability of mesoporous Fe–CaSiO3 materials was also carried out in the SBF solution. Typically, 0.1 g of mesoporous Fe–CaSiO3 material was compacted into a pellet of 6 mm diameter by uniaxial compression at 3 MPa. Mesoporous Fe–CaSiO3 pellets were soaked in the SBF solution in a polyethylene bottle at 37 °C for different periods (MFe–CaSiO3/VSBF = 2 mg ml−1). After soaking, mesoporous Fe–CaSiO3 pellets were collected from the SBF solution, rinsed with ethanol and dried. SEM observations and energy-dispersive x-ray spectroscopy (EDS) measurements were used to study the apatite formation on the surfaces of mesoporous Fe–CaSiO3 pellets.
2.3. Cytotoxicity evaluation of mesoporous Fe–
materials
To investigate the cytotoxicity of mesoporous Fe–CaSiO3 materials, Osteoblast-like MC3T3-E1 cells and WST-8 assay were used in this study. Prior to the cytotoxicity evaluation, the extracts of mesoporous Fe–CaSiO3 materials were prepared in culture medium according to International Standard Organization (ISO/EN) 10993-5,Footnote4 by adding mesoporous Fe–CaSiO3 materials to serum-free α-MEM medium (without l-glutamine or ascorbic acid) at a final concentration of 50 mg ml−1. After incubating at 37 °C for 24 h, the mixtures were centrifuged and the supernatants collected. Serial dilutions of extracts (25, 12.5 and 6.25 mg ml−1) were prepared using serum-free α-MEM medium. The diluted extracts were filter sterilized and used for subsequent MC3T3-E1 cell culture experiments.
To evaluate the cytotoxicity of mesoporous Fe–CaSiO3 materials, MC3T3-E1 cells were seeded at a density of 5 × 103 cells cm−2 into a 96-well plate with regular α-MEM medium and incubated for 24 h, after which the medium was removed and replaced by 50 μl of α-MEM medium supplemented with 20% FBS and 50 μl of diluted extracts. For a blank control, 100 μl of α-MEM medium supplemented with 10% FBS but without the addition of diluted extracts was used. The cells were incubated at 37 °C in a humidified 5% CO2 atmosphere for 7 days. Subsequently, WST-8 (cell counting kit-8, CCK-8) assay was performed by adding 10 μl of CCK-8 solution to each well and incubated for 3 h at 37 °C to form WST-8 formazan. The absorbance of the WST-8 formazan was read at 450 nm on a microplate reader (MTP-880, Corona). The results were expressed as the absorbance reading from each well less the optical density value of a blank.
2.4. Magnetic heating property of magnetic mesoporous Fe–
materials
For the magnetic heating experiment, a high-frequency generator (power 5 kW) was used. The used inductor was a water-cooled copper coil with 11 turns on a length of 105 mm and a diameter of 42.5 mm. For measurement, an open-top vessel with a dispersion of magnetic mesoporous Fe–CaSiO3 materials (100 mg ml−1) was placed in the inductor. While applying the alternating magnetic field, the temperature was monitored using a pyrometer that was placed above the inductor and focused on the dispersion surface. To obtain the heating curve of the magnetic mesoporous Fe–CaSiO3 materials, a reference measurement of the pure solvent (equivalent volume) was subtracted from the data.
2.5. Loading and in vitro release of gentamicin
1.0 g of mesoporous Fe–CaSiO3 materials was immersed in 40 ml of gentamicin solution (10 mg ml−1). After 24 h, the drug-loaded mesoporous Fe–CaSiO3 materials were separated and dried at room temperature in vacuum for 48 h. The concentration of gentamicin in mesoporous Fe–CaSiO3 materials was estimated by measuring the absorbance values at 256 nm before and after the loading [Citation41]. Before determination, a calibration curve was recorded.
In vitro release of gentamicin from the drug-loaded mesoporous Fe–CaSiO3 materials was carried out with a shaking bed at 37 °C. The drug-loaded mesoporous Fe–CaSiO3 materials (0.5 g) were placed in a cover-sealed plastic bottle with 20 ml of the SBF solution, and the plastic bottle was fixed on a shaking bed with a shaking speed of 50 rpm. Gentamicin release was determined by UV analysis. The release medium was withdrawn at predetermined time intervals, and replaced with fresh SBF solution each time.
3. Results and discussion
3.1. Characterization of mesoporous Fe–
materials
Figure shows wide-angle XRD patterns of mesoporous Fe–CaSiO3 materials. Similar to the previously reported mesoporous CaSiO3, Sr–CaSiO3 and magnesium–CaSiO3 materials [Citation7–Citation9, Citation23, Citation24], mesoporous Fe–CaSiO3 materials also lacked diffraction peaks related to the incorporated Fe ions except for a broad reflection at 2θ = 25–35°, which indicated the amorphous structure of Fe–CaSiO3. Generally, amorphous CaSiO3 has quicker apatite-formation ability due to the rapid release of Ca ions compared to α-CaSiO3 and β-CaSiO3 [Citation42]. Therefore, the amorphous mesoporous Fe–CaSiO3 materials could contribute to better bioactivity.
Figure shows TEM images and EDS analysis of mesoporous Fe–CaSiO3 materials. Well-ordered mesoporous structure can be clearly observed on mesoporous CaSiO3 materials without Fe substitution (pore sizes about 5–6 nm). When 5 and 10% Fe were substituted for Ca in mesoporous CaSiO3 materials, similarly ordered mesoporous channels with mesopore size of 5–6 nm could be seen, which suggested that the substitution of Fe for Ca did not change the mesoporous structure of mesoporous CaSiO3 materials. From EDS analysis, Ca and Si were detected in the 0Fe–CaSiO3 material, while the Fe, Ca and Si were clearly detected in the 5Fe–CaSiO3 and 10Fe–CaSiO3 materials, which indicated that Fe was successfully incorporated in the 5Fe–CaSiO3 and 10Fe–CaSiO3 materials. However, no iron oxide particles or aggregates could be detected, indicating that the Fe ions substituted the Ca ions in the framework of mesoporous CaSiO3 materials, but did not form the magnetic nanoparticles inside the channels, which were similar to the previously reported results by Wu et al [Citation39] and Gu et al [Citation43].
Figure 2. TEM images and the corresponding EDS analysis of mesoporous Fe-CaSiO3 materials with different Fe substitution ((A) and (D): 0Fe-CaSiO3; (B) and (E): 5Fe-CaSiO3; (C) and (F): 10Fe-CaSiO3).
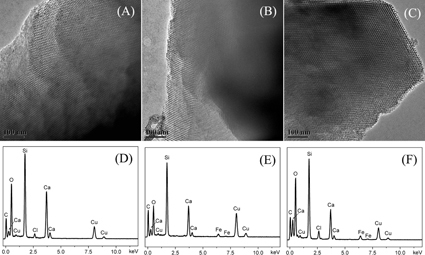
Elemental mapping was used to further confirm the distributions of Fe, Ca and Si in mesoporous Fe–CaSiO3 materials. As shown in figure , the chemical compositions of Fe, Ca and Si were distributed homogeneously in the 5Fe–CaSiO3 and 10Fe–CaSiO3 materials, which were similar to the Zr-, Mg-, Sr- and Fe-substituted mesoporous bioactive glass prepared using sol–gel technique [16, Citation38]. Therefore, the results further indicated that Fe ions substituted Ca ions in the framework of mesoporous CaSiO3 materials. In this study, mesoporous Fe–CaSiO3 materials were prepared after the calcination at 600 °C for 6 h and reduction in 10%H2 per 90%Ar atmosphere at 400 °C for 3 h, which might form magnetite in mesoporous Fe–CaSiO3. Li et al [Citation44] and Chen et al [Citation45] have reported that hematite could transform to magnetite in the Fe-incorporated mesoporous bioactive glasses and mesoporous silica particles after the reduction treatment under hydrogen atmosphere.
Figure 3. Element mappings of mesoporous Fe-CaSiO3 materials with different Fe substitution ((A): 0Fe-CaSiO3; (B): 5Fe-CaSiO3; (C): 10Fe-CaSiO3).
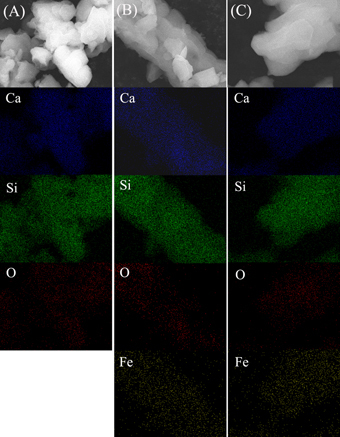
N2 adsorption–desorption isotherms of mesoporous Fe–CaSiO3 materials are shown (figure ) together with the corresponding pore size distributions. The data for the surface area, pore volume and peak pore size are listed in table . The Fe substitution in mesoporous CaSiO3 did not change the mesoporous structure. The type IV isotherms with a type H1 hysteresis loop were similar to those of the reported mesoporous CaSiO3 materials [Citation7, Citation11, Citation23, Citation24], indicating the P6mm mesoporous structure of mesoporous Fe–CaSiO3 materials. The BET surface areas (SBET) of the 0Fe–CaSiO3, 5Fe–CaSiO3 and 10Fe–CaSiO3 materials were 188.1 137.2 and 134.3 m2 g−1, respectively. The single point adsorption total volume (VP) at P/P0 = 0.97 for the 0Fe–CaSiO3, 5Fe–CaSiO3 and 10Fe–CaSiO3 materials were 0.233, 0.208 and 0.201 cm3 g−1, respectively. The substitution of Fe for Ca in mesoporous CaSiO3 decreased the surface area and pore volume, suggesting the decrease in the ordering degree of the 5Fe–CaSiO3 and 10Fe–CaSiO3 materials compared to the 0Fe–CaSiO3 materials. The substitution of Fe for Ca in mesoporous Fe–CaSiO3 might have generated defects in the mesoporous framework due to the difference of ion valence and radius, which has been reported in the substituted mesoporous bioactive glass [Citation16]. Previous studies have reported on the doping of Fe into glass-ceramics and Ca–P ceramics to make them magnetic, but they are unsuitable for drug delivery due to the absence of a nanoporous structure [Citation30–Citation33]. Furthermore, pore radius (RP) distributions of mesoporous Fe–CaSiO3 were narrow and peaked at 2.5–3 nm. In this study, the substitution of Fe for Ca in mesoporous CaSiO3 materials decreased their surface area and pore volume. However, the mesoporous structure and large surface area of mesoporous Fe–CaSiO3 materials (> 130 m2 g−1) are beneficial for adsorption and sustained release of drugs.
Figure 4. N2 adsorption-desorption isotherms (A) and the corresponding pore size distributions (B) of mesoporous Fe-CaSiO3 materials.
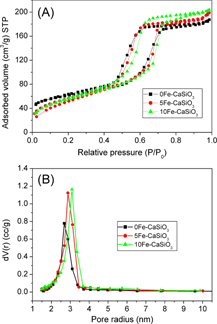
Table 2. Structural parameters and gentamicin loading of mesoporous Fe–CaSiO3 materials.
3.2. Magnetic heating ability of mesoporous Fe–
materials
The room temperature magnetization curves of the 0Fe–CaSiO3, 5Fe–CaSiO3 and 10Fe–CaSiO3 materials are shown in figure (A). The magnetization of mesoporous Fe–CaSiO3 materials increased with increase in Fe substitution. The 0Fe–CaSiO3 materials have no magnetization due to their non-magnetic nature, whereas the 5Fe–CaSiO3 and 10Fe–CaSiO3 materials had a magnetization of 0.73 and 1.19 emu g−1 at 25 kOe, respectively, because the incorporated Fe ions formed a magnetite structure. Also, almost no hysteresis loops were observed on the curves of the 5Fe–CaSiO3 and 10Fe–CaSiO3 materials, suggesting superparamagnetic behavior. In practice, magnetic field generator that can operate under an AC frequency of 50 kHz–1.2 MHz and maximum magnetic field strength of 15 kA m−1 is considered safe for the human body [Citation33]. In this study, the magnetic heating experiments were limited to an AC frequency of 200 kHz and magnetic field strength of 1.86 kA m−1, respectively. Figure (B) shows the temperature increments of mesoporous Fe–CaSiO3 materials in an alternating magnetic field. The 0Fe–CaSiO3 suspension exhibited almost no increase in temperature during the experimental period. However, the temperatures of the 5Fe–CaSiO3 and 10Fe–CaSiO3 suspensions increased by 5.2 and 7.9 °C in 20 min, respectively. Therefore, the results indicated that the Fe substitution in mesoporous CaSiO3 materials can produce magnetic property, and the magnetic strength of mesoporous CaSiO3 materials can be tailored by changing the Fe substitution. Furthermore, magnetic mesoporous Fe–CaSiO3 materials can generate heat to raise the temperature of the surrounding environment due to the delay in Neel relaxation of the magnetic moment in mesoporous Fe–CaSiO3 materials [Citation46], allowing for magnetic hyperthermia application.
3.3. Ion release and apatite formation ability of mesoporous Fe–
materials
Studies demonstrated that the release of ions from biomaterials such as Si and Ca ions could stimulate the osteoblast proliferation and differentiation [Citation47, Citation48]. On the other hand, the release of ions changes the pH around biomaterials, which will affect the cell growth and the osseointergration ability [Citation18, Citation19]. Therefore, the release of Si, Ca and Fe ions from mesoporous Fe–CaSiO3 in the SBF solution was investigated. As shown in figure , the release of Ca and Si ions increased with the increase in soaking time. However, the concentrations of Ca and Si ions decreased with increasing the Fe substitution, which might be because Fe ions provide more bonds to Si and O network than Ca ions, contributing to more stable network for mesoporous Fe–CaSiO3 materials. The concentrations of Fe ions for the 5Fe–CaSiO3 and 10Fe–CaSiO3 materials were <1 mg ml−1 (not shown), which indicates that the concentration of released Fe ions was too low for ICP-OES detection. Another reason might be that the released Fe ions could be precipitated again due to the basic environment. The results were similar to those of the previously reported Fe-incorporating mesoporous bioactive glass [Citation38, Citation39]. Figure (C) shows the pH changes of the SBF solutions after soaking mesoporous Fe–CaSiO3 materials. It can be observed that the pH values of mesoporous Fe–CaSiO3-soaked SBF solutions decreased with increase in the Fe substitution in mesoporous Fe–CaSiO3 materials, and that the pH values could be stabilized at 8.2, 7.9 and 7.7 after 7 days of soaking for the 0Fe–CaSiO3, 5Fe–CaSiO3 and 10Fe–CaSiO3 materials, respectively. It indicated that the substitution of Fe for Ca in mesoporous CaSiO3 materials improved the ability to stabilize the pH environment, which is beneficial for cell growth on mesoporous Fe–CaSiO3 materials.
Figure 6. Ca (A) and Si (B) ion concentrations in SBF solutions and pH values (C) of SBF solutions after soaking mesoporous Fe-CaSiO3 materials for various time periods.
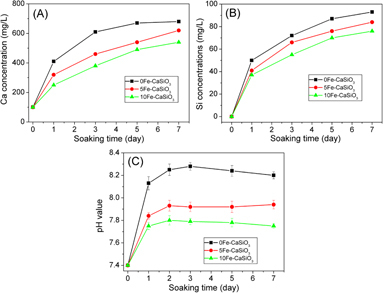
It has been accepted that the apatite-formation ability of a biomaterial in SBF solution is useful for predicting the in vivo bone bioactivity of a biomaterial, and the formation of apatite layer shows the ability of a biomaterial to form interfacial bonds with tissues when in contact with physiological fluid [Citation49]. In this study, the apatite formation on mesoporous Fe–CaSiO3 materials after soaking in the SBF solution was investigated by SEM with EDS analysis. As shown in figure , after soaking mesoporous Fe–CaSiO3 materials in SBF for 3 days, a new layer of apatite particles that had a diameter of several micrometers and flower morphology were formed on the surface of the pellets. Phosphorus signals appeared in the EDS spectra of mesoporous Fe–CaSiO3 materials after soaking in the SBF, and the Ca/P ratio was 1.79, 1.92 and 1.95 for the 0Fe–CaSiO3, 5Fe–CaSiO3 and 10Fe–CaSiO3 materials, respectively. The results indicated that mesoporous Fe–CaSiO3 materials possess good apatite-formation ability in physiological fluid. On the other hand, it can also be observed that the apatite layer exhibited a small decrease in thickness for mesoporous Fe–CaSiO3 materials with increasing Fe substitution, suggesting a small decrease in the apatite-formation rate. Generally, the release of Ca ions from a biomaterial accelerates the apatite formation due to the increase of Ca concentration in the SBF solution. In this study, the Ca ions were released from the 0Fe–CaSiO3, 5Fe–CaSiO3 and 10Fe–CaSiO3 materials, but the Ca ions release rates showed small decrease with increase in the Fe substitution in mesoporous CaSiO3 materials, which contributed to small decrease in the apatite-formation rate for mesoporous Fe–CaSiO3 materials with increase in the Fe substitution.
3.4. Cytotoxicity evaluation of mesoporous Fe–
materials
To evaluate the cytotoxicity of mesoporous Fe–CaSiO3 materials, the elution cell culture assay (also known as extract dilution) was used in this study. The cytotoxic effect of mesoporous Fe–CaSiO3 extracts on osteoblast-like MC3T3-E1 cells is shown in figure . It can be seen that there were no cytotoxic effects on MC3T3-E1 cells for mesoporous Fe–CaSiO3 materials from a low extract concentration (6.25 mg ml−1) to a high extract concentration (50 mg ml−1) after 7 days. The 5Fe–CaSiO3 and 10Fe–CaSiO3 extracts had comparable cell viability compared to the 0Fe–CaSiO3 extracts. However, the extracts of mesoporous Fe–CaSiO3 materials with concentrations from 6.25 to 25 mg ml−1 improved the proliferation of MC3T3-E1 cells compared to the blank control. The corresponding ion concentrations of mesoporous Fe–CaSiO3 extracts for cell culture are listed in table . The Ca, Si and Fe ions were released from mesoporous Fe–CaSiO3 materials in culture medium. The Ca and Si ion concentrations respectively ranged from 72.7 to 96.3 mg ml−1 and 4.9 to 63.1 mg ml−1, while the Fe ion concentrations were lower than 2.8 mg ml−1. Previous studies showed that high concentrations of Ca, Si and Fe ions can result in excessive cytotoxicity [25, Citation50]. In this study, the results indicated that the 0Fe–CaSiO3, 5Fe–CaSiO3 and 10Fe–CaSiO3 extracts were not cytotoxic to MC3T3-E1 cells and could induce osteoblast activity, suggesting that the released Ca, Si and Fe ions from mesoporous Fe–CaSiO3 materials are in the feasible levels and are very useful for bone regeneration.
Figure 8. The cytotoxic effect of mesoporous Fe-CaSiO3 extracts on MC3T3-E1 cells evaluated by WST-8 assay.
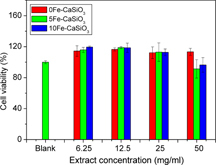
Table 3. The Ca, Si and Fe ion concentrations of mesoporous Fe–CaSiO3 extracts (mg l−1).
3.5. Gentamicin release from mesoporous Fe–
materials
Besides the magnetic heating ability and bioactivity, mesoporous Fe–CaSiO3 materials can also efficiently load drugs, such as antibiotics, for local drug delivery, which is very useful for bone regeneration, because implantation always leads to inflammatory responses and, quite often, to infections [Citation51]. In this study, gentamicin, an antibiotic, was used as a model drug to investigate the drug loading and release behavior of mesoporous Fe–CaSiO3 materials. The gentamicin-loading capacities of the 0Fe–CaSiO3, 5Fe–CaSiO3 and 10Fe–CaSiO3 materials were estimated at 155, 125 and 124 mg g−1, respectively. Figure shows the accumulative gentamicin release from mesoporous Fe–CaSiO3 materials in SBF at 37 °C. It can be seen that gentamicin in mesoporous Fe–CaSiO3 materials revealed a sustained release in the SBF solution. The 0Fe–CaSiO3, 5Fe–CaSiO3 and 10Fe–CaSiO3 materials exhibited a similar release behavior throughout the whole study period, with an initial fast release followed by a relatively slow release. Furthermore, the gentamicin release rates from the 0Fe–CaSiO3, 5Fe–CaSiO3 and 10Fe–CaSiO3 materials were close to each other, and the accumulative release of gentamicin was around 85% after 1 week. It suggested that mesoporous Fe–CaSiO3 materials maintained the sustained drug delivery property, and the substitution of Fe for Ca in mesoporous CaSiO3 materials did not change the drug release kinetics.
Figure 9. Gentamicin release profiles from mesoporous Fe-CaSiO3 materials with different Fe substitution in SBF solution.
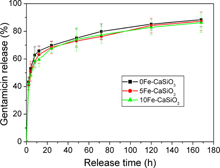
In this study, the mesopore sizes of the 0Fe–CaSiO3, 5Fe–CaSiO3 and 10Fe–CaSiO3 materials were between 5.3 and 6.2 nm, which are much larger than the size of a gentamicin molecule (0.52 × 1.53 nm) [Citation52], indicating that gentamicin molecules could be loaded in the mesoporous channels. Furthermore, studies demonstrated that silicate-based biomaterials can easily form Si–OH groups on the surface of materials, allowing interacting with gentamicin molecules by hydrogen bonding [Citation53]. On the other hand, the gentamicin release was determined by the mesoporous structure and the interaction between gentamicin and the surface of mesoporous Fe–CaSiO3 materials. The 0Fe–CaSiO3, 5Fe–CaSiO3 and 10Fe–CaSiO3 materials exhibited similar mesoporous structure and surface characteristics, which result in similar gentamicin release kinetics. Therefore, the mesoporous structure of mesoporous Fe–CaSiO3 materials and the Si–OH groups on their surface help to adsorb gentamicin and benefit the sustained release, which indicates their potential as a local drug delivery system for bone tissue regeneration.
4. Conclusions
Multifunctional magnetic mesoporous Fe–CaSiO3 materials have been prepared using P123 as a structure-directing agent. The substitution of Fe for Ca in mesoporous CaSiO3 materials did not change their mesoporous structure, but endowed them with magnetic property. The results indicated that mesoporous Fe–CaSiO3 materials exhibited good bioactivity and sustained drug delivery property. Furthermore, magnetic mesoporous Fe–CaSiO3 materials could generate heat in an alternating magnetic field for potential hyperthermia application. Therefore, magnetic mesoporous Fe–CaSiO3 materials have potential for the regeneration of bone defects caused by bone tumors with local drug delivery and magnetic hyperthermia therapy. Further studies will be conducted to investigate how to fabricate mesoporous Fe–CaSiO3 scaffolds with multifunctionality for bone regeneration.
Acknowledgments
The authors gratefully acknowledge the support by the Program for Professor of Special Appointment (Eastern Scholar) at Shanghai Institutions of Higher Learning, National Natural Science Foundation of China (no. 51102166), Program for New Century Excellent Talent in University (no. NCET-12-1053), Key Project of Chinese Ministry of Education (no. 212055), Shanghai Pujiang Program (no. 11PJ1407300), Shanghai Shuguang Project (no. 12SG39) and Innovation Program of Shanghai Municipal Education Commission (no. 12ZZ140).
Notes
4 ISO/EN 10993-5. Biological evaluation of medical devices—part 5 tests for cytotoxicity, in vitro methods: 8.2 tests on extracts.
References
- De AzaP NGuitiánFDe AzaS 1997 Biomaterials 18 1285 10.1016/S0142-9612(97)00063-X
- XueWLiuXZhengXDingC 2005 Biomaterials 26 3455 10.1016/j.biomaterials.2004.09.027
- FeiLWangCXueYLinKChangJSunJ 2012 J. Biomed. Mater. Res. B 100B 1237 10.1002/jbm.b.32688
- NiSChangJChouL 2006 J. Biomed. Mater. Res. A 76A 196 10.1002/jbm.a.30525
- YanXHuangXYuCDengHWangYZhangZ 2006 Biomaterials 27 3396 10.1016/j.biomaterials.2006.01.043
- OstomelT AShiQTsungC KLiangHStuckyG D 2006 Small 2 1261 10.1002/smll.200600177
- LiXShiJZhuYShenWLiHLiangJGaoJ 2007 J. Biomed. Mater. Res. B 83B 431 10.1002/jbm.b.30813
- XiaWChangJ 2008 Micropor. Mesopor. Mater. 108 345 10.1016/j.micromeso.2007.04.013
- WuCChangJFanW 2012 J. Mater. Chem. 22 16801 10.1039/c2jm33387b
- WeiJChenFShinJHongHDaiCSuLLiuC 2009 Biomaterials 30 1080 10.1016/j.biomaterials.2008.10.046
- ZhuHWuBFengXChenJ 2011 J. Biomed. Mater. Res. B 98B 330 10.1002/jbm.b.31856
- FanYHuangSJiangJLiGYangPLianHChengZLinJ 2011 J. Colloid Interface Sci. 357 280 10.1016/j.jcis.2011.01.109
- ArigaKVinuAYamauchiYJiQHillJ P 2012 Bull. Chem. Soc. Japan 85 1 10.1246/bcsj.20110162
- HeQShiJ 2011 J. Mater. Chem. 21 5845 10.1039/c0jm03851b
- DerbyB 2012 Science 338 921 10.1126/science.1226340
- ZhuYLiXYangJWangSGaoHHanagataN 2011 J. Mater. Chem. 21 9208 10.1039/c1jm10838g
- SalinasA JShrutiSMalavasiGMenabueLVallet-RegíM 2011 Acta Biomater. 7 3452 10.1016/j.actbio.2011.05.033
- WuCRamaswamyYKwikDZreiqatH 2007 Biomaterials 28 3171 10.1016/j.biomaterials.2007.04.002
- RamaswamyYWuCZhouHZreiqatH 2008 Acta Biomater. 4 1487 10.1016/j.actbio.2008.04.014
- ZreiqatHRamaswamyYWuCPaschalidisALuZJamesBBirkeOMcDonaldMLittleDDunstanC R 2010 Biomaterials 31 3175 10.1016/j.biomaterials.2010.01.024
- ChenXOuJWeiYHuangZKangYYinG 2010 J. Mater. Sci. Mater. Med. 21 1463 10.1007/s10856-010-4025-5
- WuCRamaswamyYSoepartoAZreiqatH 2008 J. Biomed. Mater. Res. A 86A 402 10.1002/jbm.a.31623
- LuJ et al 2012 Micropor. Mesopor. Mater. 163 221 10.1016/j.micromeso.2012.06.037
- ZhuYZhuMHeXZhangJTaoC 2013 Acta Biomater. 9 6723 10.1016/j.actbio.2013.01.021
- YamasakiKHagiwaraH 2009 Toxicol. Lett. 191 211 10.1016/j.toxlet.2009.08.023
- WuYJiangWWenXHeBZengXWangGGuZ 2010 Biomed. Mater. 5 015001 10.1088/1748-6041/5/1/015001
- PanseriSCunhaCD’AlessandroTSandriMRussoAGiavaresiGMarcacciMHungC TTampieriA 2012 PLoS ONE 7 e38710 10.1371/journal.pone.0038710
- MengJZhangYQiXKongHWangCXuZXieSGuNXuH 2010 Nanoscale 2 2565 10.1039/c0nr00178c
- ParsonsA JEvansMRuddC DScotchfordC A 2004 J. Biomed. Mater. Res. A 71A 283 10.1002/jbm.a.30161
- BockNRiminucciADionigiCRussoATampieriALandiEGoranovV AMarcacciMDediuV 2010 Acta Biomater. 6 786 10.1016/j.actbio.2009.09.017
- ZengXHuHXieLLanFJiangWWuYGuZ 2012 Int. J. Nanomed. 7 3365 10.2147/IJN.S32264
- Martín-SaavedraF MRuíz-HernándezEBoréAArcosDVallet-RegíMVilaboaN 2010 Acta Biomater. 6 4522 10.1016/j.actbio.2010.06.030
- MurakamiSHosonoTJeyadevanBKamitakaharaMIokuK 2008 J. Ceramic Soc. Japan 116 950 10.2109/jcersj2.116.950
- KawashitaMIwahashiYKokuboTYaoTHamadaSShinjoT 2004 J. Ceram. Soc. Japan 112 373 10.2109/jcersj.112.373
- Julián-LópezBBoissièreCChanéacCGrossoDVasseurSMirauxSDuguetESanchezC 2007 J. Mater. Chem. 17 1563 10.1039/b615951f
- LeeHKimSChoiB HParkM TLeeJJeongS YChoiE KLimB UKimCParkH J 2011 Int. J. Hyperthermia 27 698 10.3109/02656736.2011.608217
- HuMBelikA AImuraMMibuKTsujimotoYYamauchiY 2012 Chem. Mater. 24 2698 10.1021/cm300615s
- ZhuYShangFLiBDongYLiuYLoheM RHanagataNKaskelS 2013 J. Mater. Chem. B 1 1279 10.1039/c2tb00262k
- WuCFanWZhuYGelinskyMChangJCunibertiGAlbrechtVFriisTXiaoY 2011 Acta Biomater. 7 3563 10.1016/j.actbio.2011.06.028
- KokuboTTakadamaH 2006 Biomaterials 27 2907 10.1016/j.biomaterials.2006.01.017
- ZhuYKaskelS 2009 Micropor. Mesopor. Mater. 118 176 10.1016/j.micromeso.2008.08.046
- SiriphannonPKameshimaYYasumorAOkadaKHayashiS 2002 J. Eur. Ceram. Soc. 22 511 10.1016/S0955-2219(01)00301-6
- GuJDongXElangovanS PLiYZhaoWIijimaTYamazakiYShiJ 2012 J. Solid State Chem. 186 208 10.1016/j.jssc.2011.12.018
- LiXWangX PHuaZ LShiJ L 2008 Acta Mater. 56 3260 10.1016/j.actamat.2008.03.013
- ChenYChenH RZengD PTianY BChenFFengJ WShiJ L 2010 ACS Nano 4 6001 10.1021/nn1015117
- KumarC S S RMohammadF 2011 Adv. Drug Deliv. Rev. 63 789 10.1016/j.addr.2011.03.008
- ValerioPPereiraM MGoesA MLeiteM F 2004 Biomaterials 25 2941 10.1016/j.biomaterials.2003.09.086
- MaenoSNikiYMatsumotoHMoriokaHYatabeTFunayamaA 2005 Biomaterials 26 4847 10.1016/j.biomaterials.2005.01.006
- KokuboTItoSHuangZHayashiTSakkaSKitsugiT 1990 J. Biomed. Mater. Res. 24 331 10.1002/jbm.820240306
- WuC TFanWGelinskyMXiaoYSimonPSchulzeRDoertTLuoY XCunibertiG 2011 Acta Biomater. 7 1797 10.1016/j.actbio.2010.12.018
- Vallet-RegiM 2006 Dalton Trans. 44 5211 10.1039/b610219k
- DoadrioA LSousaE M BDoadrioJ CParienteJ PBarbaI IVallet-RegiM 2004 J. Control Release 97 125 10.1016/j.jconrel.2004.03.005
- XiaWChangJ 2006 J. Control Release 110 522 10.1016/j.jconrel.2005.11.002