Abstract
Novel low-dimensional thermoelectric (TE) materials suffer from poor mechanical reliability, which limits their applications, especially in mechanically harsh environments. Here, we propose a new concept, in which the novel, abundant, thermally stable TE-nanostructures are dispersed and then intimately embedded inside a protective, mechanically reliable tetragonal ZrO2 (TZP) ceramic matrix with a low thermal conductivity. We also demonstrate an experimental proof-of-principle verification of our concept in reduced-graphene oxide (GO)–3 mol% Y2O3–ZrO2 (3YSZ or 3Y-TZP) nanocomposite system. TE characterizations suggest that our protective TZP matrix does not degrade the intrinsic TE property of the reduced GO network. These preliminary results are promising and encouraging to start research on similar TZP-matrix TE-nanocomposites, which contain more effective TE-nanostructures with larger intrinsic power factors. In this regard, we propose a scalable approach for fabrication of similar dense TE-nanocomposites composed of other one-dimensional and/or two-dimensional TE-nanostructures, which involves an aqueous colloidal approach and a subsequent spark plasma sintering. These new TZP-matrix TE-nanocomposites could be used for sustainable clean power generation, especially in mechanically harsh environments with thermal/mechanical shocks and vibrations, where energy availability, reliability and durability are more important than the energy efficiency. Considering the excellent biocompatibility of TZP matrix, they could even be used inside the body to power implanted medical devices.
Introduction
Harvesting clean electricity from waste heat, an abundant source of energy, by using thermoelectric (TE) materials could indeed play an important role in addressing the energy crisis in the near future. The performance of TE solid is generally judged by the value of dimensionless figure of merit, ZT, which is S2σT/κ, where S is Seebeck coefficient or thermopower, σ is electrical conductivity, κ is thermal conductivity and T is temperature [Citation1, Citation2]. The higher the ZT, the higher the heat-electricity conversion efficiency; currently, materials with ZT around 1 could be considered as potential candidates for commercial applications in temperature ranges where they are stable. Without considering the materials price, availability and environmental issues, some prominent examples are systems of (Bi1−xSbx)2(Se1−yTey)3 alloys (bismuth chalcogenides) [Citation3–Citation6], Pb–Te alloys [Citation7, Citation8], Si–Ge alloys [Citation9–Citation11], phonon-glass electron-crystal systems such as partially filled skutterudites (CoSb3 family) [Citation12–Citation15], half Heusler alloys [Citation16–Citation18], and transition metal oxides (layered cobaltites [Citation19–Citation22] and Ruddlesden–Popper phase SrTiO3-based perovskites in the form of (SrTiO3)n(SrO)m [Citation23, Citation24], doped ZnO [Citation25, Citation26], etc). In conventional TE material systems, increasing the ZT has been a great challenge, as S, σ and κ are interrelated and could not easily be controlled independently; for instance increasing σ would generally decreases S and enhances the carrier contribution to the total κ (κlattice + κcarrier) [Citation1–Citation26]. Note that S is strongly dependent on the carrier concentration and thereby σ. However, as the material size decreases approaching nanometer scales, new phenomena such as quantum-confinement effects could potentially enhance S and facilitate an independent control of S, σ and κ over a wide range of temperature for further increasing of ZT (e.g. increasing S more than decreasing σ) [Citation2]. In addition, by reducing the size, many interfaces are created, which could potentially scatter phonons more effectively than the electrons and therefore, effectively lower the lattice κ at the same time [Citation2]. These possibilities attracted many researchers and started a new field of low-dimensional thermoelectricity in 1990s [Citation2].
Now, it has been well established that nanostructures and nanostructured TE composites, compared to their normal bulk counterparts with similar compositions, could possess superior TE characteristics. Some prominent examples are 2D PbTe/Pb1−xEuxTe quantum-well superlattices [Citation27, Citation28], PbTe/PbSe0.98Te0.02 quantum-dot superlattices [Citation29] and Sb2Te3/Bi2Te3 superlattice thin films [Citation30], nanostructured Si–Ge bulks [Citation31] or superlattices [Citation32, Citation33], disordered plasma-treated few-layer graphene (FL-G) films [Citation34] and carbon nanotubes (CNTs) [Citation35], 2D hexagonal CoO2 in layered cobaltites [Citation19–Citation22, Citation36, Citation37], one-dimensional (1D) [Citation38, Citation39] and two-dimensional (2D) [Citation40] bismuth chalcogenide nanostructures, Bi nanowires [Citation41, Citation42], 1D [Citation43, Citation44] and 2D [Citation45, Citation46] perovskite SrTiO3 nanostructures and Ca9Co12O28 nanowires [Citation47]. Unfortunately, these promising low-dimensional TE systems are highly fragile and mechanically unreliable (e.g. even fracture toughness of their sintered, dense bulk counterparts is highly poor, even less than 1 MPa m0.5 [Citation48]), the fact that reduces their reliability especially in mechanically harsh environments. Most of them are also expensive and contain rare and environmentally hazardous elements. However, if those abundant, inexpensive and environmentally safe TE-nanostructures, such as oxides [Citation36, Citation37, Citation43–Citation47] and semiconducting carbon [Citation34, Citation35] could be dispersed like a three-dimensional (3D) connective network and embedded within a protective strong, tough, hard and wear-resistant ceramic host matrix having also a very low thermal conductivity, the important issue of poor mechanical reliability of these novel low-dimensional TE systems might be effectively addressed.
Here, we propose our new concept, in which tetragonal ZrO2-based ceramics (hereafter referred to as TZP) with excellent mechanical properties and low thermal conductivities [Citation49–Citation54] are used as a host matrix to protect the novel 1D and/or 2D TE-nanostructures and their fragile networks, especially in mechanically harsh environments. In addition, we demonstrate an experimental proof-of-principle verification of our concept in reduced few-layer graphene oxide (FL-GO)–3 mol% Y2O3-stabilized TZP (3YSZ or 3Y-TZP) matrix system. Here, thermally reduced FL-GO nanosheets are considered as the thermally stable TE-nanostructures. Furthermore, we propose a facile, scalable approach for the large-scale fabrication of similar TE-nanocomposites, which involves an aqueous colloidal approach [Citation55] and a subsequent spark plasma sintering (SPS) [Citation56, Citation57].
The concept
A schematic illustration of our concept is presented in figure . In this nanocomposite system, the abundant, environmentally safe and thermally stable TE-nanostructures (e.g. nanowires, nanotubes, nanosheets) forming a 3D electrically conductive network inside the host insulator TZP ceramic matrix, would generate electricity from the temperature gradient, while nicely protected, especially in mechanically harsh environments, by the nanostructured TZP matrix having excellent mechanical properties and a low thermal conductivity.
Figure 1 Conceptualization of the TZP-matrix TE nanocomposite system, where abundant and environmentally safe 1D and/or 2D TE nanostructures forming an electrically conductive 3D network are embedded in a strong, tough, hard and wear-resistant TZP host matrix with a low thermal conductivity. The 3D network of TE nanostructures produces electricity from the temperature gradient, while nicely protected by the nanostructured TZP ceramic matrix, especially in mechanically harsh environments.
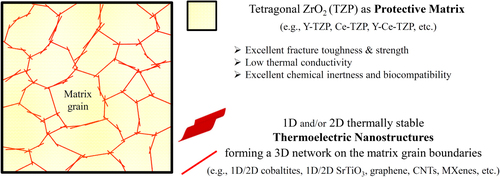
On the protection of TE-nanostructures, TZP ceramics with stabilized tetragonal structure could be the best mechanically reliable and low-thermal conductivity matrix material for this concept. Thanks to their metastable tetragonal structure, TZP ceramics are one of the strongest and toughest structural ceramics ever, which possess a unique combination of excellent fracture toughness (∼ 10 MPa m0.5), flexural strength (∼ 1–2 GPa), hardness (∼ 15 GPa), stiffness, wear resistance, thermal-shock resistance, chemical inertness and thermal stability [Citation49–Citation54]. In addition, they have a very low thermal conductivity (∼ 2–3 W m−1 K−1) among strong/tough ceramics, which is an essential prerequisite in our concept [Citation52]. Furthermore, due to their excellent biocompatibility, they have been widely used in medical industry, particularly orthopedics (e.g. hip implants) and dentistry [Citation51, Citation53, Citation54]. In order to stabilize the nice high-temperature tetragonal structure of ZrO2 at lower temperatures, some additives such as Y2O3 and/or CeO2, etc must be used to prevent transformation of tetragonal phase to the monoclinic one upon cooling or environmental changes; the resulting TZP could then be labeled as Y-TZP, Ce-TZP, Y-Ce-TZP, etc with tunable mechanical properties.
On the TE-nanostructures, the important issue of thermal stability must be considered in our concept: i.e. the possible TE-nanostructures must be stable at temperatures above 1300 K to allow their incorporation into the TZP matrix by an advanced consolidation process such as SPS [Citation56–Citation59]. With this in mind, transition metal oxides [Citation19–Citation24, Citation36, Citation37, Citation43–Citation47, Citation60–Citation62], various carbons [Citation34, Citation35], and possibly the layered, ternary transition metal carbides and nitrides (MAX-phases [Citation63]), which all have intrinsically high melting point and thermal stability and are composed of abundant and environmentally safe elements, could be the potential sources of the TE-nanostructures in our concept. Here, we present some examples.
In the metal oxide family, TE-nanostructures could either be directly synthesized using chemical or hydrothermal methods (e.g. p-type NaxCoO2 nanosheets [Citation64]; n-type SrTiO3 nanowires [Citation43], nanotubes [Citation44] and nanosheets [Citation45, Citation46]; Ca9Co12O28 nanowires [Citation47]; p-type Na2−xHxTi3O7 protonated titanate nanotubes [Citation65]; p-type La1−xSrxCoO3 nanowires [Citation66], etc) or just extracted from the layered cobaltites such as p-type NaxCoO2 [Citation19] and Ca3Co4O9 [Citation21] through exfoliation. The exfoliation of Na7CoO2 powders through the selective etching of Na layers by acid treatment has already been reported, which resulted in preparation of NaxCoO2 and/or CoO2 TE-nanosheets [Citation36, Citation37]. In this TE-oxide, hexagonal CoO2 layers, which currently could be extracted as nanosheets, are responsible for the hole conduction and the high thermopower, S (100 μV K−1 at 573 K for a single crystal). Similar exfoliation possibilities could therefore be expected in the other layered cobaltites [Citation20–Citation22, Citation60–Citation62] and even Ruddlesden–Popper phase SrTiO3-based perovskites [Citation23, Citation24].
Carbon nanostructures could be potentially used as TE-nanostructure in our concept because of their remarkable thermal stability in vacuum. In the carbon family, disordered semiconducting graphene nanosheets, nanoribbons and CNTs could be the best TE-nanostructure candidates [Citation34, Citation35]. So far, TE performance of carbon nanostructures, macrostructures and their polymer–matrix nanocomposites has been the subject of various experimental studies, which mainly triggered by the theoretical works predicting, for instance, great ZT values exceeding 4 for graphene nanoribbons [Citation67, Citation68] and large Seebeck coefficient exceeding 500 μV K−1 for the CNTs [Citation69].
Layered, ternary transition metal carbides, nitrides or carbonitrides family (known as MAX-phases with over 60 members) [Citation63] could also be considered as the potential sources of thermally stable TE-nanostructures. Recently, Al-containing MAX-phases were exfoliated by using HF treatment selectively removing Al layers and leaving the MC nanosheets such as Ti3C2, Ti2C, etc behind [Citation70] (M is an early transition metal). The functionalities of these novel nanosheets, referred to as MXene, are currently unexplored. Recent first-principles calculations showed that some single-layer MC-nanosheets such as Ti2C–O2 (available) and Sc2C–(OH)2 (hypothetical), which become semiconductor after possible functionalization with O and OH groups, could possess giant Seebeck coefficients at low temperatures; e.g. ∼1140 μV K−1 for Ti2C–O2 and ∼2200 μV K−1 for Sc2C–(OH)2 single layers at 100 K [Citation71]. Future success in the exfoliation of other MAX-phase solids could bring new opportunities for the TE community.
These novel TZP-matrix TE-nanocomposites could be used for sustainable clean power generation, especially in mechanically harsh environments with thermal/mechanical shocks and vibrations, where energy availability, reliability and durability are more important than the energy efficiency. Considering the excellent biocompatibility of the TZP matrix, such novel nanocomposites could even be used inside the body, for instance, to power implanted medical devices [Citation51, Citation53, Citation54].
Proof-of-principle verification in GO–3YSZ system
Ultimately, we demonstrate an experimental proof-of-principle verification of our concept in reduced-GO–3YSZ (Y-TZP) matrix nanocomposite system. We used FL-GO nanosheets as an example of thermally stable, organic TE-nanostructures because of their facile large-scale preparation and excellent colloidal stability in water without the need of any polymers or surfactants.
As mentioned earlier, there have been many experimental studies investigating the TE performance of novel carbon nanostructures such as CNTs and graphene assembled either as film, paper [Citation34, Citation35, Citation72–Citation74] or 3D sponge-like bulk [Citation75] or used as TE-filler for conductive polymer matrix composites [Citation73, Citation76, Citation77]. Note that all these macrostructures are highly fragile especially in mechanically harsh environments. Although the experimental results have been disappointing (ZT ∼ 10−4–10−2 and S ∼ 10–80 μV K−1 [Citation72–Citation77]) mainly because of low intrinsic Seebeck coefficients (maximum ∼80 μV K−1); however, recent studies reported great improvements. For instance, Xiao et al showed that the Seebeck coefficient of FL-G films grown by chemical vapor deposition (CVD) could reach ∼700 μV K−1 at 575 K after a 15 s oxygen plasma treatment (thermopower of pristine CVD-grown FL-G film was measured to be ∼40 μV K−1 at room temperature (RT) and ∼80 μV K−1 at 575 K) [Citation34]. It was shown that despite a 30% decrease of electrical conductivity after the treatment (∼ 2–3 × 104 S m−1), the power factor, S2σ could be significantly enhanced to ∼4.5 × 10−3 W m−1 K−2. This unprecedented TE performance was attributed to the local disordered carbons opening a band gap [Citation34]. A similar strategy using Ar-plasma has also been applied by Zhao et al for the CNTs, and they reached a Seebeck coefficient of ∼350 μV K−1, conductivity of ∼990 S m−1, and ZT of 0.4 (0.01 for pristine) at ∼670 K for semiconducting single-walled CNT (SWCNT) paper treated for 20 s (note that for pristine SWCNT-Bucky paper, maximum thermopower was ∼50 μV K−1 at 720 K) [Citation35]. These promising results could indeed justify the use of carbon nanostructures in our concept.
We also propose a facile approach for the scalable fabrication of similar TZP-matrix TE-nanocomposites composed of other 1D and/or 2D TE-nanostructures, which involves an aqueous surfactant-free or surfactant-assisted colloidal approach and a subsequent SPS consolidation process.
Processing of GO–3YSZ nanocomposite
Our method is very simple in principle but greatly effective in achieving uniformity, in terms of dispersion of TE-nanostructures and grain size distribution of TZP host matrix, which indeed determine the mechanical reliability and functionality of the TE-nanocomposite. As demonstrated in figure , it basically involves preparation of separate, stable aqueous suspensions of well dispersed TE-nanostructures and TZP nanoparticles, which have high surface-charge densities (zeta potential 30 mV) with opposite signs, at similar pH values. Once these suspensions are mixed together, the neighboring oppositely charged TE and TZP nanostructures easily attract each other by electrostatic forces, then form heavy composite aggregates and settle down short after the mixing is stopped (see figure (b)). If the supernatant of the mixing suspensions become transparent or at least free of TE-nanostructures, it guarantees no aggregation and uniform dispersion of individual TE-nanostructures within the TZP powders. Otherwise, TE-nanostructures would obviously re-agglomerate during the water removal, which indeed must be avoided. In order to prevent such re-agglomeration of TE-nanostructures during drying, the relative solid loading of the suspensions must be controlled; i.e. the overall surface area of the TZP nanoparticles must be large enough to completely attract their guest TE-nanostructures and avoid re-agglomeration during drying. In this regard, we established a criterion as follows: addition of TZP suspension into the TE-nanostructure suspension of any solid loadings is continued until the supernatant becomes free of TE-nanostructures after a few minutes of stagnancy. According to our experience with FL-GO aqueous suspension, 3YSZ suspensions having a range of solid loadings are able to satisfy this requirement (i.e. completely attract the FL-GO nanosheets), and therefore, homogeneous composite powders with different TE-loadings could be synthesized.
Figure 2 Processing of GO–3YSZ matrix nanocomposite (a) Bright-field TEM images of individual FL-GO nanosheets of our concentrated aqueous suspension, and their zeta potential–pH relationship in water; (b) uniform dispersion of the negatively charged FL-GO nanosheets within the positively charged 3YSZ nanoparticles, in water, by using a scalable, entirely aqueous and surfactant-free colloidal method, and fabrication of fully dense bulk by using SPS. Here, thermally reduced, conductive FL-GO nanosheets are considered as an example for 2D TE-nanostructures. The TG analysis (part b, weight loss–temperature graph) precisely determined the FL-GO content in the composite powder to be 2.65 wt%.
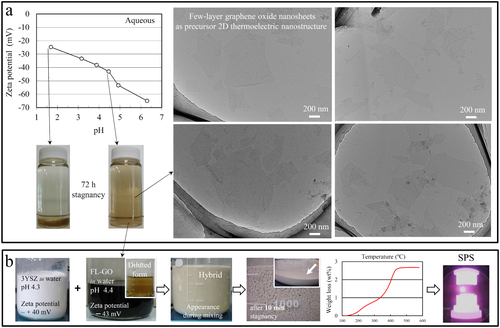
For the FL-GO/3YSZ system, at first, a highly stable, concentrated, aqueous FL-GO suspension was prepared; the details are described in the experimental section (see figure (a)). Next, we kept adding the aqueous 3YSZ (TZ-3Y-E grade, Tosoh, Japan) suspension into the FL-GO one until the entire FL-GO nanosheets were arrested by the 3YSZ nanoparticles (here, 20 g 3YSZ loading) and a clear supernatant was observed. The settled composite powders and the clear supernatant are shown in figure (b). Having the black color of the concentrated FL-GO suspension in mind, the bright color of the mixture is a nice evidence for the uniform dispersion of FL-GO nanosheets within the 3YSZ nanoparticles. The precise FL-GO loading of the powders was determined by thermal gravimetry (TG) to be 2.65 wt%. After drying, fully dense nanocomposite was fabricated by a rapid, pressure/current assisted densification process, commonly known as electric current activated/assisted sintering or SPS [Citation56–Citation59]. This scalable, environmentally friendly and inexpensive method could be applied to any other TZP-matrix TE-nanocomposite system in our concept (section 2), once the related aqueous suspension of TE-nanostructure is prepared. Recently, we successfully used this method to fabricate novel CNT-concentrated Al2O3 matrix nanocomposites with advanced functional [Citation58, Citation78, Citation79] and structural properties [Citation59, Citation80, Citation81].
Figure shows the bright-field scanning transmission electron microscopy (BF-STEM) images of the spark plasma sintered FL-GO/3YSZ matrix nanocomposite. According to various BF-STEM images similar to those presented, individual FL-GO nanosheets are uniformly dispersed on the grain boundaries of YSZ host matrix and form an interconnecting network. STEM mode of imaging, compared to conventional TEM, enables a better demonstration of the FL-GO interconnecting network within the YSZ matrix. The YSZ matrix is also shown to be nanostructured, pore-free and structurally uniform. The average grain size of the matrix is ∼250 nm. It is obvious that the FL-GO nanosheets strongly inhibit the grain growth of 3YSZ matrix during sintering, and since they were uniformly dispersed within the matrix, the grain refinement was also realized uniformly throughout the whole nanocomposite. Similar graphene-assisted grain refinements have been also reported in Si3N4 [Citation82] and Al2O3 [Citation83] matrix composites. Conventional BF-TEM images demonstrated in figure , also confirm the excellent structural uniformity of the nanocomposite in terms of FL-GO dispersion and grain size distribution of YSZ matrix.
Figure 3 BF-STEM images of fully dense, spark plasma sintered FL-GO/3YSZ nanocomposite, clearly demonstrating its outstanding structural homogeneity in terms of FL-GO dispersion and 3YSZ grain size distribution. Thin interconnecting bright lines with highly comparable thicknesses are the FL-GO nanosheets, thermally reduced during SPS, and uniformly dispersed on the grain boundaries of nanostructured 3YSZ matrix.
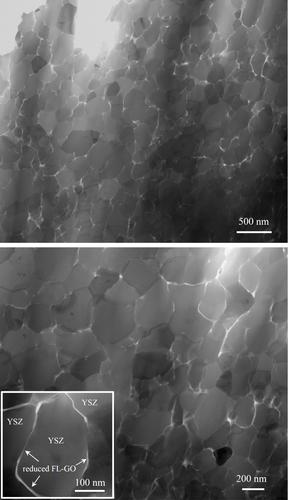
Figure 4 TEM images of fully dense, spark plasma sintered FL-GO/3YSZ nanocomposite. (a) Bright-field TEM images with different magnifications confirming the structural homogeneity of the nanocomposites in terms of GO dispersion and 3YSZ grain size distribution; (b) high-resolution TEM images of atomically intimate, pore-free GO/3YSZ interfaces; (c) STEM image with the corresponding STEM-EDS elemental mapping.
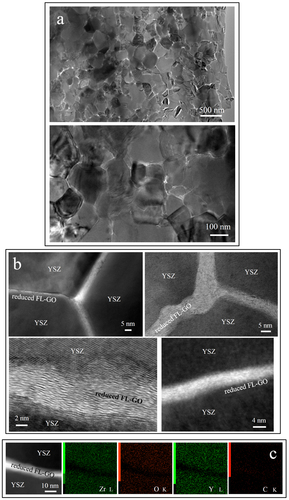
According to many high-resolution TEM (HRTEM) images similar to those shown in figure (b), individual FL-GO nanosheets having highly comparable thicknesses are located on the triple junctions and grain boundaries, and form atomically intimate interfaces with 3YSZ matrix. No secondary interfacial phases either amorphous or crystalline were detected, suggesting the great thermal stability of FL-GO nanosheets and 3YSZ matrix in vacuum, at 1573 K under 90 MPa stress.
Another interesting feature is the irregularities formed within the internal layers of individual FL-GO nanosheets (figure (b)). In an artistic way, FL-GO nanosheets resemble ‘flowing rivers’ in the ‘YSZ land’. Such irregularities could enable the physical connection of the interior layers to each other and to the surface layers, and thus, enhance the overall functionality and performance of FL-GO nanosheets, for instance, in load bearing and transferring the charge carriers. Note that in general, carbon layers in graphite and multiwalled CNTs (MWCNTs) do not interact and are separated by weak van der Waals forces, so that the majority of the mechanical and electrical loads are carried only by the surface layers [Citation84, Citation85]. Formation of similar irregularities within individual MWCNTs, when they are embedded in an Al2O3 ceramic matrix, has also been reported by Estili et al [Citation84, Citation85]; i.e. MWCNTs were shown to become dramatically stronger in the ceramic matrix due to these irregularities causing inter-wall load transfer and highly energy-dissipating multiwall-type failures [Citation84, Citation85]. Formation of these irregularities in our FL-GO nanosheets could be a side-effect of graphite oxidation and exfoliation. Furthermore, considering the mismatch in thermal expansion coefficients and elastic moduli of FL-GO and YSZ matrix, another mechanism likely to be responsible for the generation of such beneficial internal irregularities might be the residual stresses (thermal misfit stress) leading to buckling of FL-GO nanosheets in c-axis direction. These misfit stresses, if compressive on FL-GO network, could also effectively enhance the overall connectivity of carbon 3D-network, reduce their junction resistance and increase the overall electrical conductivity of the TE-nanocomposite. A prominent example is an exceptional 5000 S m−1 dc-electrical conductivity of a concentrated MWCNT 3D-network (20 vol%), which was intimately embedded within an Al2O3 ceramic environment [Citation58]. Its large value of conductivity is comparable to those of pure hot-pressed, aligned [Citation86], or highly compacted MWCNT bulks [Citation87], and dramatically higher than porous sponge-like MWCNT bulks [Citation75].
Figure shows some SEM and TEM images from the fracture surface, which further support the STEM and TEM observations (figures and ) and reconfirm the success of our scalable method in the uniform dispersion of FL-GO nanosheets within a nanostructured, pore-free and structurally uniform 3YSZ host matrix. Observation of pulled out, separated nanosheets on the fracture surface means that no chemical reaction could have occurred at the interfaces (grain boundaries) during SPS, consistent with HRTEM images (figure (b)). Perhaps, the physical YSZ–GO–YSZ bonding in some grain boundary area could explain the entirely intergranular fracture mode of this nanocomposite system [Citation58, Citation59]. If these pullout processes are frictional and contribute to energy dissipation near the existing flaws' tips, then some improvements in fracture toughness and strength of the YSZ matrix could also be expected [Citation59, Citation84, Citation85], similar to those claimed in Si3N4-matrix composites [Citation82]. Here using only these images, we are unable to determine whether or not such pullout process is frictional and energy dissipating. Finally, despite being embedded in YSZ ceramic, the FL-GO nanosheets must have been highly flexible to allow them deflecting the crack around their surface area.
Figure 5 Fracture surfaces of fully dense, spark plasma sintered FL-GO/3YSZ nanocomposite. (a), (b) SEM images with different magnifications showing the exceptionally uniform dispersion of reduced FL-GO nanosheets within the structurally uniform, nanostructured 3YSZ matrix. (c), (d) TEM images of some individual FL-GO nanosheets partially pulled out from the 3YSZ matrix during fracture.
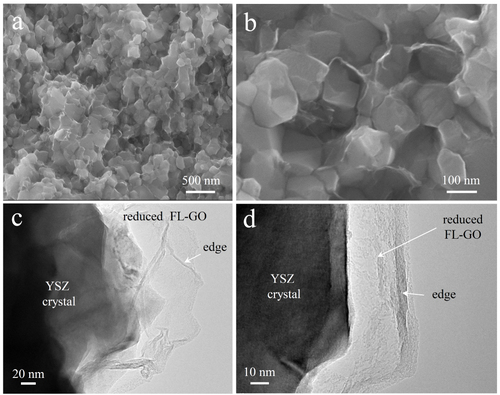
TE characterizations
Figure presents the electrical conductivity, Seebeck coefficient, thermal conductivity, power factor and TE figure-of-merit, ZT of the fully dense spark plasma sintered FL-GO/3YSZ matrix nanocomposite, as a function of temperature. The high value of electrical conductivity (600–700 S m−1 between RT and 673 K) confirms the formation of highly connective 3D-network of thermally reduced FL-GO nanosheets inside the insulating 3YSZ ceramic matrix. The increase of conductivity with the temperature shows a semiconducting-like behavior. Note that although the as-prepared GO is an insulator, it could become conductive upon appropriate chemical [Citation88] or thermal [Citation89] reductive processes. Therefore, SPS is shown here to be effective in efficient deoxygenation and structural restoration of the FL-GO nanosheets as well, without the need of any toxic reducing chemicals such as hydrazine [Citation88, Citation89]. This is further supported by the effective attenuation of carbon–oxygen peaks in the high-resolution C1 s x-ray photoelectron spectroscopy (XPS) measurements after SPS. As shown in figure , the amount of carbon–oxygen bonds detected in the C1 s spectra of dried composite powder (49.25%) is decreased to 21.41% after SPS. This also means that the weight of FL-GO nanosheets must have been reduced after SPS due to significant loss of atomic oxygen, and therefore, our value of 2.65 wt% only applies to the composite powder, while it should be much larger than the real FL-GO loading of the spark plasma sintered nanocomposite. Similar thermal reduction of GO was also reported in GO–Si3N4 system, reaching RT conductivities of 693 S m−1 (at 7.2 vol%) and 97 S m−1 (at 4.3 vol%) after comparatively longer thermal reduction process during SPS, in vacuum (30 min at 873 K, and 5 min at 1898 K under 50 MPa) [Citation90].
Figure 6 The relationships of the DC-electrical conductivity (a), Seebeck coefficient (b), thermal conductivity (c), power factor (d) and TE figure-of-merit, ZT (e) with the operating temperature, in the fully dense, spark plasma sintered FL-GO/3YSZ nanocomposite.
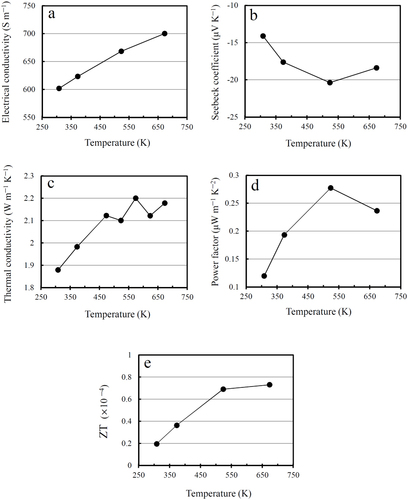
The absolute Seebeck coefficient values are in the range 14.1–20.4 μV K−1 at 300–673 K (figure (b)) and comparable to those reported for the majority of pure CNT, graphene and GO macrostructures [Citation34, Citation72–Citation75] and CNT polymer–matrix composites [Citation35, Citation73, Citation76, Citation77]. Here are some examples in the literature: Xiao et al measured the S values of hydrazine-reduced GO film to be in the range −7 to 16 μV K−1 with conductivities in the range 2000–9000 S m−1, at 300–525 K [Citation34]. They also reported p-type S values in the range 40–80 μV K−1 and conductivities in the range 40 000–50 000 S m−1 for the CVD-grown few-layer graphene film, at 300–575 K [Citation34]. Zhao et al [Citation35] fabricated flexible SWCNT paper showing p-type S values in the range 30–50 μV K−1 and conductivities in the range 3500–4800 S m−1, at 300–750 K. Liu et al [Citation72] reported p-type S value of 32 μV K−1 and conductivity of 3373 S m−1 for a SWCNT film at RT. Meng et al [Citation73] reported p-type S values in the range 12–17 μV K−1 for dense, 0.22 mm thick MWCNT film, 25–28 μV K−1 for 0.5 mm thick MWCNT array and 22–28 μV K−1 for polyaniline (PANI)-coated MWCNT film. For a porous sponge-like MWCNT bulk, Chen et al [Citation75] reported p-type S values in the range 18–27 μV K−1 and conductivities in the range 120–150 S m−1, at 300–600 K. Zhang et al [Citation76] processed 10–30 wt% MWCNT–PANI matrix composite bulks with p-type S values in the range 20–30 μV K−1 and conductivities in the range 3.5–160 S m−1, at 300–380 K. The majority of these carbon macrostructures show p-type behaviors with holes as the dominant charge carriers.
The negative sign of S suggests the n-type behavior in the examined temperature range (300–673 K), which means that electrons are the dominant charge carriers in our spark plasma sintered nanocomposite. It is interesting and promising that the Seebeck coefficient and electrical conductivity follow the same increasing trend from RT to 523 K, which results in the largest power factor of 0.28 μW m−1 K−2 at 523 K (figure (d)); in general, S and σ show opposing behavior [Citation1, Citation2]. This value is also comparable to those reported, for instance, for hydrazine-reduced GO film (0.8–3.6 μW m−1 K−2 at 300–525 K) [Citation34], pure SWCNT films (3.45 μW m−1 K−2 at RT) [Citation35], SWCNT–Nafion composites (< 0.1 μW m−1 K−2 at RT) [Citation77], MWCNT–Nafion composites (0.05–0.8 μW m−1 K−2 at RT) [Citation77], PANI-coated MWCNT films (0.5–5 μW m−1 K−2 at RT) [Citation73] and MWCNT–PANI composite bulks (0.002–0.1 μW m−1 K−2 at 300–380 K) [Citation76].
To sum up, considering all the experimental thermopower, S and power factor, S2σ values of the films, bulks and composites of various carbon nanostructures reported in the literature (especially the reduced GO film [Citation34]), it is fair to conclude that our protective, insulator 3YSZ ceramic matrix does not degrade the intrinsic TE property of the reduced FL-GO 3D network. While the conversion efficiency of this particular GO-TZP nanocomposite system is not very attractive for practical applications, however, these results are promising and encouraging to start research on similar TZP-matrix TE-nanocomposites, which contain more effective TE-nanostructures with larger loadings and intrinsic power factors, S2σ, as described in section 2, the concept.
Another promising and unprecedented observation is the ability of FL-GO 3D network to considerably decrease the thermal conductivity of 3YSZ matrix, τ, from 2.962 to 1.879 W m−1 K−1, at RT (figure (c)). This could be explained by the formation of large amount of new interfaces and grain boundaries as a result of FL-GO addition (figures –), which could effectively scatter phonons and reduce the thermal conductivity [Citation2]. Furthermore, the large number of defects in reduced FL-GO nanosheets, which are reflected in Raman spectra (figure ) could also act as scattering centers and reduce not only the electronic and lattice thermal conductivity of reduced FL-GO network but also the thermal conductivity of YSZ matrix. In Raman analysis, the intensities, broadenings and the ratio of D-band (defect mode) and G-band (graphite mode) are generally used to qualitatively evaluate the in-plane C–C integrity and disorders of carbonaceous materials [Citation91–Citation93]. Upon oxidation and exfoliation of graphite, the appearance of D-band, the significant increase of ID/IG ratio, broadening and shift of G-band, slight merging of D and G-bands, and disappearance of 2D-band clearly demonstrate the high level of defects available in FL-GO nanosheets before SPS. After SPS, however, the reduction of G-band broadening and ID/IG ratio, complete disappearance of G-band shift, complete separation of D and G-bands, and appearance of 2D-band strongly imply the considerable restoration of sp2 carbon sites and removal of carbon–oxygen bonds, which are supported by the high electrical conductivity of FL-GO network and the C1 s XPS spectra (figure ). The symmetry of 2D-band of FL-GO nanosheets in the spark plasma sintered nanocomposite is attributed to the disruption of stacking order in c-axis, which is indeed expected after exfoliation [Citation92, Citation93].
Finally, thermal conductivity values were used to calculate the TE figure-of-merit, ZT values (as S2σT/κ), which determine the overall TE performance of our spark plasma sintered nanocomposite. As demonstrated in figure (e), ZT increases with the temperature reaching the maximum of 0.73 × 10−4 at 673 K. Note that at 523 K, where the nanocomposite can properly work in air without burning of surface FL-GO nanosheets, ZT value of 0.69 × 10−4 is very close to the maximum obtained at 673 K. Thanks to the low thermal conductivity of the spark plasma sintered nanocomposite, our ZT values are also comparable to the majority of those reported for pure carbon macrostructures and polymer–matrix nanocomposites. The only difference is that our new nanocomposite could be reliably used in mechanically harsh environments with thermal/mechanical shocks and vibrations, where energy availability, reliability and durability are more important than the energy efficiency.
Experimental details
Preparation of graphite oxide powder.
Graphite oxide powder is the precursor to obtain FL-GO nanosheets. We used a modified version of Hummers and Offeman's method for oxidizing the graphite powder [Citation94, Citation95]. In a typical experiment, 5.09 g of the as-received, synthetic, conducting-grade graphite powder (Alfa Aesar; 200 mesh, 99.9995% purity) was slowly treated, for 5 days, in the strong oxidizing mixture of 3.75 g NaNO3 (99.5%, Hikotaro Shudzui Co. Ltd, Japan), 170 ml H2SO4 95% (Junsei Chemical Co. Ltd, Japan) and 22.5 g KMnO4 (99.3%, Nacalai Tesque Inc., Japan), at RT, in a round-bottom flask, under continuous stirring using magnetic stirrer. 525 ml of 5 wt% H2SO4 aqueous solution was then slowly added to the 5-day stirred, highly viscous, brown-colored mixture under continuous stirring. This addition was highly exothermic and thus performed slowly within about 40 min. The new mixture was gently stirred further for about 3 h. Next, 15 g of 30 wt% H2O2 aqueous solution (Junsei Chemical Co. Ltd, Japan) was added to the slurry and stirred for about 3 h. This addition generated a large amount of gas.
Next, 520 ml aqueous solution of 3 wt% H2SO4 and 0.5 wt% H2O2 was added to the final slurry for further decomposition and removal of impurities. The mixture was stirred for 10 h, and then left stagnant for about 2 days and finally its supernatant was removed. This initial purification step was repeated nine times. The resulting mixture was finally washed seven times with ultrapure water (18 MΩ cm) to completely remove the impurity ions. Each washing step involved mixing with 1000 ml ultrapure water for about 10 h, then 2–14 days stagnancy (depending on ionic concentration), removing the supernatant and addition of ultrapure water. This final time-consuming washing step was repeated seven times so that a very low ionic conductivity of 5.5 mS m−1 was achieved. Such low ionic concentration ensures a high colloidal stability for the GO nanosheets, which have a large amount of oxygen-containing functional groups.
Preparation of aqueous FL-GO suspension.
In order to prepare a highly stable, aqueous suspension of GO nanosheets, some amount of the purified graphite oxide slurry was mixed with ultrapure water and probe-sonicated for 1 h while continuously stirred using a magnetic stirrer. This sonication step led to the exfoliation of some particles and extraction of extremely thin GO nanosheets, which are negatively charged in water independent of the pH (figure ). The sonicated solution was centrifuged for 5 min at 5000 rpm and the top 70% of the centrifuge cells were then collected for further centrifuging purification. This separation process was repeated three times to entirely remove the non-exfoliated particles and aggregates. Looking at various TEM images (figures –), the final supernatant should have contained only FL-GO nanosheets. Next, pH of this solution was adjusted to ∼4.4 using NaOH solution and bath-sonicated for 1 h. At this pH, zeta potential of FL-GO nanosheets are high enough (− 43 mV) to allow their long-term stability in water even at high concentrations. This aqueous solution containing a high concentration of well-dispersed FL-GO nanosheets was stable over months with no trace of sedimentation. Note that such aqueous FL-GO suspension is black when concentrated but light-yellow when diluted (see figure (b)).
In order to determine the pH-dependence of zeta potential and colloidal stability of GO nanosheets in water, a highly diluted solution was needed. In this regard, a few droplet of the three-time centrifuged, concentrated supernatant was diluted with 200 ml of ultrapure water and bath-sonicated for 2 h. According to the zeta potential measurements (Zetasizer Nano-Z, Malvern Instruments Ltd, UK) and sedimentation tests and also the zeta potential–pH relationship of 3YSZ nanoparticles in water (e.g. +40 mV at pH ∼4.3), pH ∼ 4.4 was chosen for the concentrated, three-time centrifuged FL-GO aqueous solution.
Preparation of FL-GO/3YSZ composite powder.
This step has been thoroughly described in section 3.1. Here, we just describe the preparation of stable aqueous 3YSZ suspension and a precise determination of composition of the composite powder. A predetermined load of commercially available tetragonal 3YSZ powder (TZ-3Y-E grade, Tosoh, Japan) with a mean particle size of ∼70 nm was first mixed with 200 ml ultrapure water and ball-mixed for 2 h in a plastic container using 3 mm ZrO2 balls. This step was performed to break the rough aggregates of 3YSZ powder. Next, the resulting concentrated slurry was diluted with 1 l of ultrapure water and the pH was adjusted to ∼4.3 using HCl. Ultimately, the slurry was probe-sonicated for 2 h while vigorously stirred using a magnetic stirrer. The resulting aqueous suspension was highly stable and ready for mixing with the aqueous FL-GO suspension with a similar pH, as described in section 3.1.
TG experiment (SII EXSTAR 6000, TG/DTA 6200) was used to precisely determine the FL-GO content of the composite powder; measuring the weight loss in air after 393 K (absorbed water weight was excluded from the measurement) (figure (b)). To ensure the water removal, samples were held at 393 K for an hour. Two TG runs were performed and the results were almost the same (2.65 and 2.68 wt%) showing the exceptional dispersion uniformity of FE-GO nanosheets throughout the 3YSZ powders.
Sintering of FL-GO/3YSZ composite powder.
Consolidation of FL-GO/3YSZ powder was performed by SPS [Citation58, Citation59, Citation96] (Dr Sinter SPS-511S; Sumitomo Coal Mining Co., Tokyo, Japan) in graphite mold and ∼5 Pa vacuum atmosphere, in the following condition: 50 °C min−1 heating rate until reaching 1573 K, 12 min holding at 1573 K under continues 90 MPa stress and finally cooling inside the chamber until RT. Temperature inside a deep side-hole of the graphite die reaching 1 mm behind the powder was considered as the operating temperature, and measured using an optical pyrometer. Apparent density of the spark plasma sintered nanocomposite, 5.816 g cm−3 was measured by Archimedes principle with water as the immersion medium. The nanocomposites successfully passed several free-fall tests from heights up to 3 m, which indicates their great mechanical reliability.
Note that the weight of FL-GO nanosheets must have been considerably reduced after SPS due to significant loss of atomic oxygen (see XPS results in figure ), therefore, the real FL-GO loading of the spark plasma sintered nanocomposite should be much lower than that of composite powder, 2.65 wt%.
Characterizations.
For structural observation, we used an FE-SEM operated at 15 kV (JSM-6500F, JEOL, Tokyo, Japan), a conventional LaB6-gun TEM (JEM-2100, JEOL, Tokyo, Japan) and a field-emission TEM operated at 200 kV (JEM-2100F, JEOL, Tokyo, Japan) with STEM mode and energy dispersive spectroscopy (EDS) analyzer enabling high-resolution STEM-EDS elemental mapping. On the TEM specimen, conventional grinding followed by polishing and dimple grinding/polishing steps (Gatan 656, USA) were performed to prepare a ∼15 μm thick flat plate. The plate was then mounted on a hollow molybdenum mesh for the final thinning using a precision ion polishing system (PIPS; dual penning argon-ion gun, Gatan 691, USA).
High-resolution XPS spectra were recorded on a PHI Quantera SXM spectrometer (ULVAC-PHI, Japan) using monochromatic x-ray Al Kα radiation (0.2 mm diameter; 18 kV, 50 W). Energy calibration was performed using the characteristic Zr 3d5/2 peak of ZrO2 at 183.2 eV [Citation97]. Raman spectra were recorded at RT on a micro-Raman spectrometer (Horiba Jobin–Yvon Model T64000; Villeneuve d'Ascq, France) using a 514.532 nm laser beam. The spectra, which are normalized to the G-band intensity, were collected from many different parts of the specimen and they are highly comparable.
The Seebeck coefficient, S and electrical conductivity, σ were simultaneously measured by the standard four-probe method using the popular TE measurement system, ZEM-3 (ULVAC-RIKO, Inc., Japan) at RT, 373, 523 and 673 K, in helium atmosphere. Note that the bar sample (2 × 2 × 10 mm3) was mechanically connected to the probes without using any conductive pastes; i.e. partially exposed, reduced FL-GO nanosheets on the surface were in good contact with the probes and therefore, electricity could be transferred along the 10 mm bar sample due to their connective 3D network. Laser flash method (ULVAC TC-7000, Japan) was used to simultaneously measure the thermal diffusivity and specific heat capacity of the 10 mm disc samples (1.5 mm thick) at RT, 373, 473, 523, 573, 623 and 673 K, in argon atmosphere. The RT thermal conductivity of the pure, fully dense 3YSZ sample (6.027 g cm−3) spark plasma sintered at 1573 K under 50 MPa for 10 min was also measured for comparison. Thermal conductivity (in W m−1 K−1) at each temperature was then calculated by multiplying the density (5.816 g cm−3) to the corresponding measured thermal diffusivity (in m2 s−1) and specific heat capacity (in J g−1 K−1) values. Ultimately, the power factor (in μW m−1 K−2) and the dimensionless figure-of-merit, ZT values were calculated as S2σ and S2σT/κ, respectively.
Summary
We propose a new TE concept, in which TZP ceramics with excellent mechanical properties and low thermal conductivities are used as a host matrix to protect the novel 1D and/or 2D TE nanostructures and their fragile 3D networks, especially in mechanically harsh environments. We also demonstrate an experimental proof-of-principle verification of our concept in reduced GO–3 mol% Y2O3–ZrO2 (3YSZ or Y-TZP) matrix nanocomposite system. In the nanostructured GO–3YSZ system, the Seebeck coefficient, power factor and TE figure-of-merit, ZT values are comparable to the majority of those reported for pure carbon nanostructures and their polymer–matrix nanocomposites, which suggests that our protective TZP matrix does not degrade the intrinsic TE property of the reduced GO network. These preliminary results are promising and encouraging to start research on similar mechanically reliable TZP-matrix TE-nanocomposites containing more effective TE-nanostructures. In this regard, we propose a scalable approach for fabrication of similar dense TE-nanocomposites composed of other 1D and/or 2D TE-nanostructures, which involves an aqueous colloidal approach and a subsequent SPS.
Acknowledgments
ME conceived the idea, designed the research, performed and analyzed all the experiments and wrote the paper. WWW and YS discussed the nanocomposite processing. MK discussed the thermoelectric behavior. Yukihiro Isoda of NIMS is acknowledged for measuring the thermal conductivities. XPS and SEM characterizations were performed at Materials Analysis Station of NIMS-Sengen, and special thanks go to Akihiro Tanaka for recording and analyzing the XPS spectra. TEM characterizations were performed at TEM Station, NIMS-Sengen, and Noriko Isaka is gratefully acknowledged for technical assistance. Raman spectroscopy was performed at MANA, NIMS-Namiki. All the authors have read, discussed and commented on the manuscript. ME gratefully acknowledges the International Center for Young Scientists (ICYS) of NIMS for the research grant.
References
- SnyderG JTobererE S 2008 Nature Mater. 7 105 10.1038/nmat2090
- DresselhausM SChenGTangM YYangR GLeeHWangD ZRenZ FFleurialJ PGognaP 2007 Adv. Mater. 19 1043 10.1002/adma.200600527
- PoudelB 2008 Science 320 634 10.1126/science.1156446
- SmithG EWolfeR 1962 J. Appl. Phys. 33 841 10.1063/1.1777178
- MishraS KSatpathySJepsenO 1997 J. Phys.: Condens. Matter 9 461 10.1088/0953-8984/9/2/014
- CaoY QZhaoX BZhuT JZhangX BTuJ P 2008 Appl. Phys. Lett. 92 143106 10.1063/1.2900960
- HeremansJ PJovovicVTobererE SSaramatAKurosakiKCharoenphakdeeAYamanakaSSnyderG J 2008 Science 321 554 10.1126/science.1159725
- DughaishZ H 2002 Physica B 322 205 10.1016/S0921-4526(02)01187-0
- SlackG AHussainM A 1991 J. Appl. Phys. 70 2694 10.1063/1.349385
- BhandariabC MRoweaD M 1980 Contemp. Phys. 21 219 10.1080/00107518008210957
- ViningC BLaskowWHansonJ OVan der BeckR RGorsuchP D 1991 J. Appl. Phys. 69 4333 10.1063/1.348408
- NolasG SMorelliD TTrittT M 1999 Annu. Rev. Mater. Sci. 29 89 10.1146/annurev.matsci.29.1.89
- KawaharadaYKurosakiKUnoMYamanakaS 2001 J. Alloys Compounds 315 193 10.1016/S0925-8388(00)01275-5
- LambertonG ABhattacharyaSLittletonR TKaeserM ATedstromR HTrittT MYangJNolasG S 2002 Appl. Phys. Lett. 80 598 10.1063/1.1433911
- SharpJ WJonesE CWilliamsR KMartinP MSalesB C 1995 J. Appl. Phys. 78 1013 10.1063/1.360402
- ShenQChenLGotoTHiraiTYangJMeisnerG PUherC 2001 Appl. Phys. Lett. 79 4165 10.1063/1.1425459
- CulpS RPoonS JHickmanNTrittT MBlummJ 2006 Appl. Phys. Lett. 88 042106 10.1063/1.2168019
- XiaYBhattacharyaSPonnambalamVPopeA LPoonS JTrittT M 2000 J. Appl. Phys. 88 1952 10.1063/1.1305829
- TerasakiISasagoYUchinokuraK 1997 Phys. Rev. B 56 R12685 10.1103/PhysRevB.56.R12685
- LiSFunahashiRMatsubaraIUenoKYamadaH 1999 J. Mater. Chem. 9 1659 10.1039/a904413b
- ShikanoMFunahashiR 2003 Appl. Phys. Lett. 82 1851 10.1063/1.1562337
- FunahashiRMatsubaraISodeokaS 2000 Appl. Phys. Lett. 76 2385 10.1063/1.126354
- OhtaSNomuraTOhtaHKoumotoK 2005 J. Appl. Phys. 97 034106 10.1063/1.1847723
- LeeK HKimS WOhtaHKoumotoK 2006 J. Appl. Phys. 100 063717 10.1063/1.2349559
- TsubotaTOhtakiMEguchiKAraiH 1997 J. Mater. Chem. 7 85 10.1039/a602506d
- OhtakiMTsubotaTEguchiKAraiH 1996 J. Appl. Phys. 79 1816 10.1063/1.360976
- HicksL DHarmanT CSunXDresselhausM S 1996 Phys. Rev. B 53 R10493 10.1103/PhysRevB.53.R10493
- HicksL DDresselhausM S 1993 Phys. Rev. B 47 12727 10.1103/PhysRevB.47.12727
- HarmanT CTaylorP JWalshM PLaForgeB E 2002 Science 297 2229 10.1126/science.1072886
- VenkatasubramanianRSiivolaEColpittsTO'quinnB 2001 Nature 413 597 10.1038/35098012
- JoshiG 2008 Nano Lett. 8 4670 10.1021/nl8026795
- KogaTCroninS BDresselhausM SLiuJ LWangK L 2000 Appl. Phys. Lett. 77 1490 10.1063/1.1308271
- LeeS MCahillD GVenkatasubramanianR 1997 Appl. Phys. Lett. 70 2957 10.1063/1.118755
- XiaoN 2011 ACS Nano 5 2749 10.1021/nn2001849
- ZhaoW 2012 Energy Environ. Sci. 5 5364 10.1039/c1ee01931g
- MasudaYHamadaYSeoW SKoumotoK 2006 J. Nanosci. Nanotechnol. 6 1632 10.1166/jnn.2006.224
- ZhuP XTakeuchiTOhtaHSeoW SKoumotoK 2005 Mater. Trans. 46 1453 10.2320/matertrans.46.1453
- ZhaoX BJiX HZhangY HZhuT JTuJ PZhangX B 2005 Appl. Phys. Lett. 86 062111 10.1063/1.1863440
- SanderM SPrietoA LGronskyRSandsTStacyA M 2002 Adv. Mater. 14 665 10.1002/1521-4095(20020503)14:9%3C665::AID-ADMA665%3E3.0.CO;2-B
- KongDDangWChaJ JLiHMeisterSPengHLiuZCuiY 2010 Nano Lett. 10 2245 10.1021/nl101260j
- HeremansJThrushC M 1999 Phys. Rev. B 59 12579 10.1103/PhysRevB.59.12579
- LinY MSunXDresselhausM S 2000 Phys. Rev. B 62 4610 10.1103/PhysRevB.62.4610
- YadavG GZhangGQiuBSusorenyJ ARuanXWuY 2011 Nanoscale 3 4078 10.1039/c1nr10624d
- MaoYBanerjeeSWongS S 2003 Chem. Commun. 2003 408 10.1039/B210633G
- XuGHuangXZhangYDengSWeiXShenaGHanG 2013 CrystEngComm 15 7206 10.1039/c3ce40571k
- NiuJYanP XSeoW SKoumotoK 2012 J. Nanosci. Nanotechnol. 12 2685 10.1166/jnn.2012.5676
- YadavG GDavidAFavaloroTYangHShakouriACaruthersJWuY 2013 J. Mater. Chem. A 1 11901 10.1039/c3ta12096a
- ZhaoL DZhanB PLiJ FZhouMLiuW SLiuJ 2008 J. Alloys Compounds 455 259 10.1016/j.jallcom.2007.01.015
- GuptaT KLangeF FBechtoldJ H 1978 J. Mater. Sci. 13 1464 10.1007/BF00553200
- HanninkR H JKellyP MMuddleB C 2000 J. Am. Ceram. Soc. 83 461 10.1111/j.1151-2916.2000.tb01221.x
- LuthardtR GHolzhüterMSandkuhlOHeroldVSchnappJ DKuhlischEWalterM 2002 J. Dental Res. 81 487 10.1177/154405910208100711
- LimargaA MShianSBaramMClarkeD R 2012 Acta Mater. 60 5417 10.1016/j.actamat.2012.06.054
- DenryaIKellyJ R 2008 Dental Mater. 24 299 10.1016/j.dental.2007.05.007
- ManiconeP FIommettiP RRaffaelliL 2007 J. Dentistry 35 819 10.1016/j.jdent.2007.07.008
- SakkaY 2006 J. Ceram. Soc. Japan 114 371 10.2109/jcersj.114.371
- GrassoSSakkaYMaizzaG 2009 Sci. Tech. Adv. Mater. 10 053001 10.1088/1468-6996/10/5/053001
- OrrùRLicheriRLocciA MCincottiACaoG 2009 Mater. Sci. Eng. R 63 127 10.1016/j.mser.2008.09.003
- EstiliMKawasakiASakkaY 2012 Adv. Mater. 24 4322 10.1002/adma.201201134
- EstiliMSakkaYKawasakiA 2013 Nanotechnology 24 155702 10.1088/0957-4484/24/15/155702
- HeJLiuY 2011 J. Mater. Res. 26 1762 10.1557/jmr.2011.108
- WaliaSBalendhranSNiliHZhuiykovSRosengartenGWangQ HBhaskaranMSriramSStranoM SKalantarzadehK 2013 Prog. Mater. Sci. 58 1443 10.1016/j.pmatsci.2013.06.003
- OhtaHSugiuraKKoumotoK 2008 Inorg. Chem. 47 8429 10.1021/ic800644x
- BarsoumM W 2000 Prog. Solid State Chem. 28 201 10.1016/S0079-6786(00)00006-6
- AksitMToledoD PRobinsonR D 2012 J. Mater. Chem. 22 5936 10.1039/c2jm15550h
- MiaoLTanemuraSHuangRLiuC YHuangC MXuG 2010 ACS Appl. Mater. Interfaces 2 2355 10.1021/am100365y
- WangYFanH J 2010 J. Phys. Chem. C 114 13947 10.1021/jp105367r
- SevincliHCunibertiG 2010 Phys. Rev. B 81 113401 10.1103/PhysRevB.81.113401
- NiX XLiangG CWangJ SLiB W 2009 Appl. Phys. Lett. 95 192114 10.1063/1.3264087
- EsfarjaniKZebarjadiMKawazoeY 2006 Phys. Rev. B 73 085406 10.1103/PhysRevB.73.085406
- NaguibMMashtalirOCarleJPresserVLuJHultmanLGogotsiYBarsoumM W 2012 ACS Nano 6 1322 10.1021/nn204153h
- KhazaeiMAraiMSasakiTChungC YVenkataramananN SEstiliMSakkaYKawazoeY 2013 Adv. Funct. Mater. 23 2185 10.1002/adfm.201202502
- LiuJSunJGaoL 2011 Nanoscale 3 3616 10.1039/c1nr10386e
- MengC ZLiuC HFanS S 2010 Adv. Mater. 22 535 10.1002/adma.200902221
- KunadianIAndrewsRMengüçM PQianD 2009 Carbon 47 589 10.1016/j.carbon.2008.10.043
- ChenJ 2012 ACS Appl. Mater. Interfaces 4 81 10.1021/am201330f
- ZhangQWangWLiJWangLZhuMJiangW 2013 J. Mater. Chem. A 1 12109 10.1039/C3TA12353G
- ChoiYKimYParkS GKimY GSungB JJangS YKimW 2011 Org. Electron. 12 2120 10.1016/j.orgel.2011.08.025
- EstiliMTakagiKKawasakiA 2008 Scr. Mater. 59 703 10.1016/j.scriptamat.2008.05.042
- EstiliMTakagiKKawasakiA 2010 Mater. Sci. Forum 631–632 225 10.4028/www.scientific.net/MSF.631-632.225
- EstiliMSakkaYWuW WNishimuraTYoshidaHKawasakiA 2013 J. Am. Ceram. Soc. 96 1904 10.1111/jace.12332
- EstiliMKwonHKawasakiAChoSTakagiKKikuchiKKawaiM 2010 J. Nucl. Mater. 398 244 10.1016/j.jnucmat.2009.10.039
- WalkerL SMarottoV RRafieeM AKoratkarACorralE L 2011 ACS Nano 5 3182 10.1021/nn200319d
- WangKWangYFanZYanJWeiT 2011 Mater. Res. Bull. 46 315 10.1016/j.materresbull.2010.11.005
- EstiliMKawasakiA 2010 Adv. Mater. 22 607 10.1002/adma.200902140
- EstiliMKawasakiAPittini-YamadaYUtkeIMichlerJ 2011 J. Mater. Chem. 21 4272 10.1039/c0jm03906c
- YangKHeJSuZReppertJ BSkoveM JTrittT MRaoA M 2010 Carbon 48 756 10.1016/j.carbon.2009.10.022
- LiJWangLHeTJiangW 2009 Carbon 47 1135 10.1016/j.carbon.2008.12.041
- StankovichaSDikinD APinerR DKohlhaasK AKleinhammesAJiaYWuYNguyenS TRuoffR S 2007 Carbon 45 1558 10.1016/j.carbon.2007.02.034
- GaoXJangJNagaseS 2010 J. Phys. Chem. C 114 832 10.1021/jp909284g
- RamírezCVega-DiazS MMorelos-GómezAFigueiredoF MTerronesMOsendiM IBelmonteMMiranzoP 2013 Carbon 57 425 10.1016/j.carbon.2013.02.015
- EstiliMKawasakiASakamotoHMekuchiYKunoMTsukadaT 2008 Acta Mater. 56 4070 10.1016/j.actamat.2008.04.029
- PimentaM ADresselhausGDresselhausM SCançadoL GJorioASaitoR 2007 Phys. Chem. Chem. Phys. 9 1276 10.1039/b613962k
- CançadoL GTakaiKEnokiTEndoMKimY AMizusakiHSpezialiN LJorioAPimentaM A 2008 Carbon 46 272 10.1016/j.carbon.2007.11.015
- HirataMGotouTHoriuchiSFujiwaraMOhbaM 2004 Carbon 42 2929 10.1016/j.carbon.2004.07.003
- HummersW SOffemanR E 1958 J. Am. Chem. Soc. 80 1339 10.1021/ja01539a017
- EstiliMKawasakiA 2008 Scr. Mater. 58 906 10.1016/j.scriptamat.2008.01.016
- WangS JOngC K 2002 Appl. Phys. Lett. 80 2541 10.1063/1.1467970