Abstract
Silver nanoparticles (AgNPs) have been widely used in diverse fields due to their superior properties. Currently the biosynthesis of AgNPs is in the limelight of modern nanotechnology because of its green properties. However, relatively low yield and inefficiency diminish the prospect of applying these biosynthesized AgNPs. In this work, a rapid mass AgNP biosynthesis method using the cell-free extract of a novel bacterial strain, Lysinibacillus sphaericus MR-1, which has been isolated from a chemical fertilizer plant, is reported. In addition, the optimum synthesis conditions of AgNPs were investigated. The optimum pH, temperature, dosage, and reaction time were 12, 70 °C, 20 mM AgNO3, and 75 min, respectively. Finally, AgNPs were characterized by optical absorption spectroscopy, zeta potential and size distribution analysis, x-ray diffraction, electron microscopy, and energy-dispersive x-ray spectroscopy. The results revealed that these biosynthesized AgNPs were bimolecular covered, stable, well-dispersed face centered cubic (fcc) spherical crystalline particles with diameters in the range 5–20 nm. The advantages of this approach are its simplicity, high efficiency, and eco-friendly and cost-effective features.
1. Introduction
Over the last few decades, silver nanoparticles (AgNPs) have received substantial attention due to their attractive electronic, chemical, and optical properties [Citation1, Citation2], and they have been widely used in many fields, including catalysis, optical sensing, and electronics [Citation3, Citation4]. Currently multi-drug resistance is a growing problem in the treatment of infectious diseases. AgNPs have been applied to disinfection and therapeutics, such as infected burn and wound reduction, medical device sterilization, tumor therapy, and cardiovascular implants [Citation5, Citation6]. AgNPs are also incorporated into daily-life products, such as apparel, cosmetics, and plastics because of their antimicrobial properties [Citation7, Citation8]. The antimicrobial activity of AgNPs makes them an excellent choice for multiple uses in the medical field.
AgNPs are generally synthesized by physical and chemical methods [Citation9]. Although these two methods have been able to efficiently produce large quantities of AgNPs with a defined size and shape, they are expensive and often involve the use of toxic and hazardous chemicals, which pose an environmental risk [Citation10]. Furthermore, other problems have generally been associated with these two conventional synthetic routes, such as the aggregation of AgNPs [Citation11, Citation12]. Therefore, there is growing awareness of the need to develop environmentally friendly and sustainable methods. Microbial synthesis of nanoparticles is a green chemistry approach that interconnects nanotechnology and microbial biotechnology. Following the initial report on the formation of AgNPs in Pseudomonas stutzeri [Citation13], many reports on the synthesis of AgNPs using fungi or bacteria have appeared in the literature [Citation14]. Using microorganisms, especially their cell-free extracts, for the synthesis of AgNPs can be advantageous compared with other biological processes because microbial resources are abundant in nature, are easy to culture, and have the potential to be scaled up for large-scale synthesis. However, the biosynthesis of AgNPs by microorganisms has usually involved the use of a low concentration of Ag+(i.e., 1 mM) and a long reaction time (on the order of hours or days), which have been two major obstacles to rapid production [Citation15]. Syed and coworkers have reported the reduction of 1 mM of AgNO3 into AgNPs within 96 h via the mycelia of the thermophilic fungus Humicola sp. [Citation16]. Qian et al have investigated the assisted synthesis of AgNPs in 24 h via the cell filtrate of the endophytic fungus Epicoccum nigrum by using 1 mM of AgNO3 [Citation17]. Also, Malhotra and coauthors have revealed the biosynthesis of AgNPs in 16 h using 1 mM of AgNO3 via the cell-free supernatant of a novel marine strain of Stenotrophomonas [Citation18]. Consequently, for the purpose of commercial use, the inefficiency and low yield of AgNP biosynthesis need to be overcome urgently.
In this study, a novel approach to the highly efficient and rapid biosynthesis of metallic AgNPs using the cell-free extract of a novel bacterial strain, Lysinibacillus sphaericus MR-1, was investigated. The cell-free extract of L. sphaericus MR-1 reduced the high concentration of Ag+ into AgNPs within several tens of minutes through the use of 20 mM of AgNO3. The biosynthesized AgNPs were characterized by various techniques such as ultraviolet-visible absorption spectroscopy (UV–vis), Fourier transform infrared spectroscopy (FTIR), x-ray diffraction (XRD), field-emission scanning electron microscopy (FESEM) combined with energy-dispersive x-ray spectroscopy (EDX), and high-resolution transmission electron microscopy (HRTEM).
2. Material and methods
2.1. Strain, medium, and chemicals
The strain was isolated from soil samples collected from a chemical fertilizer plant in Huaian by the dilution plate technique on a nutrient agar plate (1% peptone, 0.3% beef extract, 0.5% NaCl, 2% agar, pH 7.5) at 37 °C. It was identified by 16 S rRNA gene sequencing performed by Sangon Biotech (Shanghai) Co., Ltd A yeast extract, peptone, and KNO3 (YPK) medium (pH 7.5), which consisted of 0.15% yeast extract, 0.25% peptone, and 0.1% KNO3, was employed to culture the isolate. AgNO3 was purchased from Sinopharm Chemical Reagent Co., Ltd All the reagents were of analytical grade and were used as purchased without any further purification.
2.2. Cell-free extract preparation
For inoculum preparation, a single clone of the isolate was transferred from the nutrient agar plate into 100 ml of sterile nutrient broth in a 250 ml Erlenmeyer flask. The flask was incubated at 37 °C for 12 h on a rotary shaker at 200 rpm. Then 10 ml of mid-log phase culture (optical density at 600 nm OD600 = 1, monitored by UV–vis spectrophotometer) were inoculated into 1000 ml Erlenmeyer flasks containing 500 ml of YPK medium. The inoculated flasks were incubated at 37 °C and shaken at 200 rpm again for 36 h, and then the cell-free extract was obtained by centrifugation (10 000 rpm, 10 min at room temperature) and decantation.
2.3. Biosynthesis of AgNPs
For the biosynthesis of the AgNPs, 0.085 g of solid AgNO3 was added into 100 ml of cell-free extract in 250 ml Erlenmeyer flasks. The reaction was carried out at 45 °C overnight in the dark on the rotary shakers. The YPK medium with the solid AgNO3 and the cell-free extract without the addition of the AgNO3 were treated under the same conditions as the controls. The visual color change in the reaction mixture from light yellow to dark brown was observed overnight with reference to the controls. The formation of silver nanoparticles was confirmed by UV–vis spectrophotoscopy.
2.4. Optimization of the AgNP synthesis conditions
The effect of three variables (substrate concentration, pH, and temperature) on the production of AgNPs was optimized by varying one parameter at a time, such as the substrate concentration (1–20 mM solid AgNO3), pH (6, 7, 8, 8.5, 9, 10, 11, 12, and 13), and temperature (20, 30, 35, 40, 45, 50, 60, 70, 80, and 90 °C). After biosynthesis, 1 ml of the AgNP mixture was taken out and centrifuged, and the pellets were washed three times. Then the pellets were re-suspended in 1 ml of deionized water with pipettes, and the solution was diluted with 10 folders and characterized with UV–vis. Under optimal conditions, the efficiency of the AgNP synthesis was evaluated by characterizing the samples made at different time intervals (0, 15, 30, 45, 60, 75, 90, 120, 180, and 240 min).
2.5. Characterization of AgNPs
The AgNP mixture was centrifuged at 10 000 rpm for 30 min to isolate the AgNPs from free proteins or other compounds present in the solution, following which the pellets were re-dispersed in sterile distilled water to get rid of any uncoordinated biological molecules. The process of centrifugation and re-dispersion in sterile distilled water was repeated three times to ensure better separation of free entities from the mixture. The localized surface plasmon resonance (SPR) of the AgNPs was characterized with a UV–vis spectrophotometer (UV-2401PC, Shimadzu, Japan) at a resolution of 1 nm in a wavelength range between 300 and 600 nm. The hydrodynamic diameter and the zeta potential of the AgNPs were measured by dynamic light scattering (DLS) using a Malvern Zetasizer Nano ZS 90 (Worcestershire, UK). HRTEM images were recorded using a Tecnai G2 F30 S-TWIN microscope operated at an accelerating voltage of 200 kV. Samples for HRTEM imaging were prepared by placing a drop of the solution sample in deionized water onto a carbon-coated Cu grid, drying it in air, and loading it into the electron microscope chamber.
Then the purified pellets were freeze-dried, and the powders were subjected to FTIR and XRD measurement. The Fourier transform infrared spectra were recorded by a Nicolet 5700, Thermo Electron Co., USA, in the range 400–4000 cm−1 at a resolution of 4 cm−1. X-ray powder diffraction measurements were carried out on a Bruker D8 Advance (Germany) instrument in Bragg–Brentano mode with Cu Kα radiation from 35 to 90° 2θ. These purified solid AgNPs were further ultrasonically dispersed with ethanol, dropped on glass slides, air dried, and examined by FESEM combined with EDX (Quanta 250, USA). The numerical data of these characterizations were processed by the software package Origin Pro 8.5.
3. Results
3.1. Morphology and molecular analysis of the isolate
The pure pale-white bacterial X11 (figure (a)) obtained on the nutrient agar plate was identified as L. sphaericus based on molecular analysis through 16 S rRNA sequencing studies (figure (b)) and was named L. sphaericus MR-1.
3.2. Biosynthesis of AgNPs
In the experiment, the formation of AgNPs was visually confirmed by the color change of the mixture from pale yellow to dark brown. This change did not occur in the negative controls (figure (a)). The preliminary investigation of the biosynthesized AgNPs was carried out by UV–vis spectroscopic analysis. It can be readily observed that the characteristic surface plasmon resonance band peak of the mixture was at 416 nm (figure (b)), indicating the presence of AgNPs [Citation19].
Figure 2. (a) Color change of reaction mixture containing different constituents (1. YPK medium; 2. YPK medium with 5 mM of AgNO3; 3. L. sphaericus MR-1 (X11) cell-free extract; 4. L. sphaericus MR-1 (X11) cell-free extract with 5 mM AgNO3). After overnight incubation, only the L. sphaericus MR-1 (X11) cell-free extract with 5 mM of AgNO3 showed an obvious color change from clear pale yellow to dark brown, confirming the formation of silver nanoparticles. (b) UV–vis spectrum of the reaction mixture containing different constituents, only the L. sphaericus MR-1 (X11) cell-free extract after the overnight incubation of the 5 mM of AgNO3 showed a peak at 416 nm, further confirming the formation of silver nanoparticles.
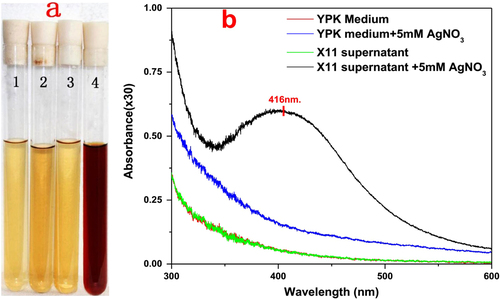
3.3. Optimization of the AgNP synthesis conditions
While the effect of temperature on the synthesis of AgNPs was being investigated, it was found that the maximum absorbance of the reaction mixture had increased from 20 to 70 °C, whereas it had decreased from 70 to 90 °C. As shown in the inset of figure (a), a nearly linear relationship between the maximum absorbance and the temperature in the range 20–70 °C was presented. The result suggested that moderately elevated temperature accelerated the reduction process.
Figure 3. (a) Effect of temperature on silver nanoparticle synthesis; inset shows a nearly linear relationship between maximum absorbance and a temperature in the range 20–70 °C. (b) Effect of pH on AgNP synthesis by L. sphaericus MR-1 cell-free extract, which showed that alkaline pH was a necessary condition of this biosynthesis procedure and pH 12 was an optimum condition; the inset shows the relationship between the maximum absorbance and pH in the range 6–13.
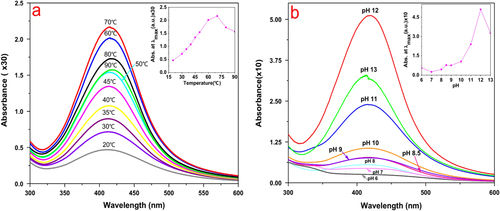
In general, the reduction reaction of metallic ions is sensitive to the pH of a solution [Citation20, Citation21]. The current study involved a systematic analysis of pH-dependent changes in the reaction mixture for the synthesis of AgNPs. It was observed that the maximum absorbance had increased when pH increased from 6 to 12 (figure (b)). The result indicated that an alkaline pH favored the formation of AgNPs.
In addition, we evaluated the effect of different concentrations of AgNO3 on the synthesis of AgNPs. The maximum synthesis of AgNPs occurred with respect to Ag+ concentration in the range 18–20 mM (figure (a)), and a few earlier researchers also showed that optimum AgNP accumulation occurred under this condition [Citation22].
Figure 4. (a) Effect of concentration of AgNO3 on AgNP synthesis by L. sphaericus MR-1 cell-free extract, which showed that 18–20 mM was an optimum condition; the inset shows the relationship between the maximum absorbance and a concentration of AgNO3 in the range 1–20 mM. (b) Time evolution of AgNP synthesis under the optimal condition; the inset shows the linear relationship between the maximum absorbance and the reduction time.
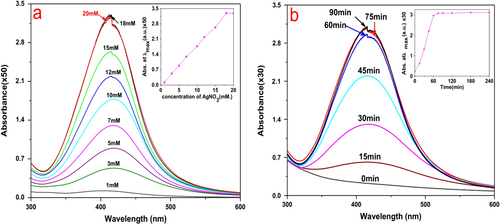
The UV–vis spectrum of AgNP synthesis under optimal conditions as a function of time is shown in figure (b). After 45, 60, and 75 min, the reduction was 71.5%, 96%, and 100% completed, respectively. It can be seen that the reduction was quite rapid and was much faster than previously reported bioreduction processes, which were on the order of hours or days, and was comparable to or faster than many chemical or physical methods [Citation15, Citation23].
3.4. Characterization of AgNPs
The typical XRD pattern of the biosynthesized AgNPs is shown in figure (a). Five diffraction peaks at 2θ values of 38.116°, 44.277°, 64.426°, 77.472°, and 81.536°, corresponding to the d-spacing values 2.359, 2.044, 1.445, 1.231, and 1.180 Å of the AgNPs, were observed. They were assigned to the (1 1 1), (2 0 0), (2 2 0), (3 1 1), and (2 2 2) crystalline planes of the face centered cubic (fcc) crystalline structure of metallic silver, respectively (JCPDS file no. 00-004-0783). The broad nature of the XRD peaks could be attributed to the nanocrystalline nature of the AgNPs. The small peaks appeared to have possibly originated from the AgCl or Ag2O crystals in the sample. The surface chemistry of the biosynthesized AgNPs was investigated using FTIR spectroscopy (figure (b)). The peaks seen at 3739.3, 3410.9, 2947.9, 2436.0, 1602.6, and 1383.1 cm−1 were assigned to the O–H, N–H, –O–CN, –CN, C=C, and C–O groups, respectively [Citation24–Citation26]. The two bands observed at 1047.1 and 827.7 cm−1 can be attributed to the –O– stretching vibrations of aromatic and aliphatic amines [Citation27, Citation28]. These functional groups may have an effective role in the green synthesis of AgNPs. The overall result confirmed the presence of bimolecular in the sample. It is reported that proteins can bind to nanoparticles through either free amine groups or cysteine residues in the proteins [Citation29]. Therefore, reduction and stabilization of the AgNPs by proteins in the cell-free extract may have occurred in this procedure.
Figure 5. (a) X-ray diffraction pattern of silver nanoparticles synthesized by cell-free extract of L. sphaericus MR-1, which showed the (2 0 0), (2 2 0), (3 1 1), and (222) crystallographic planes of face centered cubic (fcc) AgNPs. The small peaks marked with (∗) may originate from the AgCl or Ag2O crystals in the sample. (b) FTIR spectrum of silver nanoparticles synthesized by cell-free extract of L. sphaericus MR-1.
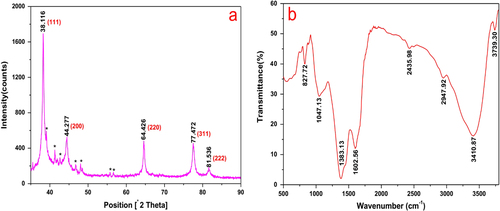
The average size distribution of silver nanoparticles in colloidal solution was found to be 14.8 ± 1.2 nm (figure (a)). A negative zeta potential of about −41.6 ± 0.5 mV was observed in the current study that represents the ideal surface charge (figure (b)). A high absolute value of zeta potential denotes a high electrical charge on the surface of AgNPs, which can cause a strong repulsive force among the particles to prevent agglomeration and which thus might be responsible for the stable nature of the AgNPs.
Figure 6. Dynamic light scattering measurements for (a) particle size distribution analysis and (b) zeta potential measurements of AgNPs.
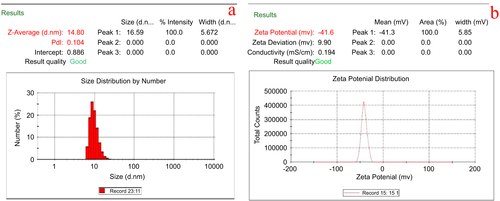
An FESEM image of the AgNPs synthesized under optimum conditions using 20 mM of silver nitrate solution revealed that the particles were spherical and well dispersed and had diameters of 5–20 nm (figure (a)). The EDX spectrum (figure (b)) confirmed the formation of AgNPs. The presence of Cl may have come from the glass slides used for the EDX sample preparation. The HRTEM images (figure ) further demonstrated the nature of AgNPs. The particle diameter was around 5–10 nm. The size seemed a little smaller than that revealed by the FESEM image and size distribution analysis, which might be explained as a result of the bimolecular coating on the AgNPs.
Figure 7. (a) FESEM image of silver nanoparticles, which were 5–20 nm in size, spherical, and well dispersed. (b) EDX spectrum of samples recorded in the area-profile mode, which clearly shows the Ag signals.
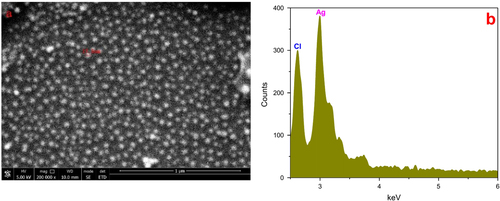
Figure 8. (a) Overview TEM image of AgNPs biosynthesized using the cell-free extract of L. sphaericus MR-1, showing that the particles are well dispersed (scale bar: 200 nm). (b) Typical TEM image of as-biosynthesized AgNPs (scale bar: 20 nm). (c) Typical HRTEM image of a single AgNP (scale bar: 5 nm). (d) typical selected area electron diffraction pattern of AgNPs biosynthesized using the cell-free extract of L. sphaericus MR-1 (scale bar: 10 1/nm).
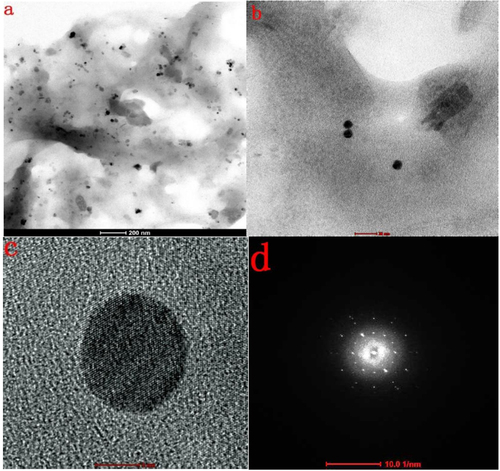
4. Discussion
Biogenic silver nanoparticles are an interesting alternative to chemically and physically produced AgNPs due to their green properties. But to be commercially available and able to compete with chemically and physically synthesized AgNPs, the production of biogenic AgNPs needs to be improved. This can be achieved by carefully choosing biological resources such as plants and microorganisms. Some plants have been used to produce AgNPs with high yield. For example, Maria et al used the extract of the stem bark of Z. xylopyrus obtained by the reflex extraction method to synthesize AgNPs. Under the condition of pH 11, 28 ± 2 °C, and 10 mM AgNO3, the extract reduced about 60% of the AgNO3 into 60–70 nm AgNPs after 24 h [Citation30]. However, large-scale production of biogenic AgNPs by the microbial method has not been explored so far. In this paper, the cell-free extract of Lysinibacillus sphaericus MR-1 was used to synthesize AgNPs. Under the condition of pH 12, 70 °C, and 20 mM of AgNO3, the extract reduced AgNO3 into 5–20 nm AgNPs in 75 min. Since the reducing agent (cell-free extract of L. sphaericus MR-1) can be easily obtained through fermentation and this microorganism resource is a renewable green material, this method for biosynthesis of AgNPs can be suitably scaled up for large-scale commercial synthesis.
In his review Berry concluded that L. sphaericus bacteria were usually used as an insect pathogen because of their parasporal crystal (BT) endotoxins [Citation31]. Also, Fayaz et al investigated the synergistic effect of biosynthesized AgNPs and antibiotics [Citation32]. Since AgNPs have a significant antimicrobial effect, the discovery of the considerable capability of this strain to synthesize AgNPs may open the door to developing multifunctional agriculture products. For example, a product functions as an insecticide and at the same time an anti-pathogen. Liong et al and Otsuka et al used modified silver nanoparticles synthesized by a chemical method as drug carriers [Citation33, Citation34]. Since the AgNPs synthesized by the cell-free extract of L. sphaericus MR-1 were covered by bimolecular, such AgNPs can be used as drug carriers in the field of biomedicine because of the functional groups on their surface.
5. Conclusions
In this study, we report rapid mass biosynthesis of AgNPs with the features of simplicity, high reduction rate, and high yield. A novel bacterial strain, L. sphaericus MR-1, was isolated from the soil of a chemical fertilizer plant. The cell-free extract of the L. sphaericus MR-1 reduced a high concentration of Ag+ to AgNPs rapidly with high yield. Under the condition pH 12, 70 °C, and 20 mM of AgNO3, the reduction was completed within 75 min. These AgNPs were characterized by various techniques, including UV–vis, XRD, FTIR, FESEM-EDX, HRTEM, zeta potential, and size distribution analysis. Results revealed that these AgNPs were monodispersed, stable, spherical in the range of 5–20 nm, and coated with biological molecules. The molecules covering the surface provide ample room for functionalized modifications, which indicates that AgNPs possess significant potential for application in the field of biomedicine.
Acknowledgments
This work was supported by the Chinese National Science Foundation (Grant No. 20971050) and Jiangsu Province College Students’ Practice and Innovation Training (Grant No. 201411049019Z). We are grateful to Mr Mujian Xia from Jiangsu Provincial Key Laboratory for interventional medical devices for FESEM images and helpful discussion. Also, we acknowledge the support extended by the Analysis Testing Center of the Department of Life Science and Chemical Engineering, HuaiYin Institute of Technology.
References
- AustinL AMackeyM ADreadenE CEl-SayedM A 2014 The optical, photothermal, and facile surface chemical properties of gold and silver nanoparticles in biodiagnostics, therapy, and drug delivery Arch. Toxicol. 88 1391 1417 1391–417 10.1007/s00204-014-1245-3
- McConnellW PNovakJ PBrousseauL CFuiererR RTenentR CFeldheimD L 2000 Electronic and optical properties of chemically modified metal nanoparticles and molecularly bridged nanoparticle arrays J. Phys. Chem. B 104 8925 8930 8925–30 10.1021/jp000926t
- MajdalawiehAKananM CEl-KadriOKananS M 2014 Recent advances in gold and silver nanoparticles: synthesis and applications J. Nanosci. Nanotechnol. 14 4757 4780 4757–80 10.1166/jnn.2014.9526
- PrathnaTRaichurA MChandrasekaranNMukherjeeA 2014 Recent developments on biosynthesis of noble metal nanoparticles: synthesis, characterization and potential applications Rev. Adv. Sci. Eng. 3 239 249 239–49 10.1166/rase.2014.1067
- ChaloupkaKMalamYSeifalianA M 2010 Nanosilver as a new generation of nanoproduct in biomedical applications Trends Biotechnol. 28 580 588 580–8 10.1016/j.tibtech.2010.07.006
- Siripattanakul-RatpukdiSFürhackerM 2014 Review: issues of silver nanoparticles in engineered environmental treatment systems Water Air Soil Poll. 225 1 18 1–18 10.1007/s11270-014-1939-4
- KokuraSHandaOTakagiTIshikawaTNaitoYYoshikawaT 2010 Silver nanoparticles as a safe preservative for use in cosmetics Nanomedicine: Nanotechnol., Biol. Med. 6 570 574 570–4 10.1016/j.nano.2009.12.002
- Marambio-JonesCHoekE M 2010 A review of the antibacterial effects of silver nanomaterials and potential implications for human health and the environment J. Nanopart. Res. 12 1531 1551 1531–51 10.1007/s11051-010-9900-y
- Abou El-NourK M MEftaihaA AAl-WarthanAAmmarR A A 2010 Synthesis and applications of silver nanoparticles Arab J. Chem. 3 135 140 135–40 10.1016/j.arabjc.2010.04.008
- PouloseSPandaTNairP PTheodoreT 2014 Biosynthesis of silver nanoparticles J. Nanosci. Nanotechnol. 14 2038 2049 2038–49 10.1166/jnn.2014.9019
- AbbasiEMilaniMFekri AvalSKouhiMAkbarzadehATayefi NasrabadiHNikasaPJooS WHanifepourYNejati-KoshkiK 2014 Silver nanoparticles: synthesis methods, bio-applications and properties Crit. Rev. Microbiol. 1 8 1–8 10.3109/1040841X.2014.912200
- LiXXuHChenZ-SChenG 2011 Biosynthesis of nanoparticles by microorganisms and their applications J. Nanomater. 2011 270974 10.1155/2011/270974
- KlausTJoergerROlssonEGranqvistC-G 1999 Silver-based crystalline nanoparticles, microbially fabricated Proc. Natl Acad. Sci. USA 96 13611 13614 13611–4 10.1073/pnas.96.24.13611
- NarayananK BSakthivelN 2010 Biological synthesis of metal nanoparticles by microbes Adv. Colloid. Interfac. 156 1 13 1–13 10.1016/j.cis.2010.02.001
- VivekanandhanSSchreiberMMasonCMohantyA KMisraM 2014 Maple leaf Acer sp. extract mediated green process for the functionalization of ZnO powders with silver nanoparticles Colloid. Surface. B 113 169 175 169–75 10.1016/j.colsurfb.2013.08.033
- SyedASaraswatiSKunduG CAhmadA 2013 Biological synthesis of silver nanoparticles using the fungus Humicola sp. and evaluation of their cytoxicity using normal and cancer cell lines Spectrochim. Acta. A 114 144 147 144–7 10.1016/j.saa.2013.05.030
- QianYYuHHeDYangHWangWWanXWangL 2013 Biosynthesis of silver nanoparticles by the endophytic fungus Epicoccum nigrum and their activity against pathogenic fungi Bioproc. Biosyst. Eng. 36 1613 1619 1613–9 10.1007/s00449-013-0937-z
- MalhotraADolmaKKaurNRathoreY SAshishSMayilraj ChoudhuryA R 2013 Biosynthesis of gold and silver nanoparticles using a novel marine strain of Stenotrophomonas Bioresour. Technol. 142 727 731 727–31 10.1016/j.biortech.2013.05.109
- AmendolaVBakrO MStellacciF 2010 A study of the surface plasmon resonance of silver nanoparticles by the discrete dipole approximation method: effect of shape, size, structure, and assembly Plasmonics 5 85 97 85–97 10.1007/s11468-009-9120-4
- YinTWalkerH WChenDYangQ 2014 Influence of pH and ionic strength on the deposition of silver nanoparticles on microfiltration membranes J. Membrane. Sci. 449 9 14 9–14 10.1016/j.memsci.2013.08.020
- BadawyA M ELuxtonT PSilvaR GScheckelK GSuidanM TTolaymatT M 2010 Impact of environmental conditions (pH, ionic strength, and electrolyte type) on the surface charge and aggregation of silver nanoparticles suspensions Environ. Sci. Technol. 44 1260 1266 1260–6 10.1021/es902240k
- GurunathanSKalishwaralalKVaidyanathanRVenkataramanDPandianS RMuniyandiJHariharanNEomS H 2009 Biosynthesis, purification and characterization of silver nanoparticles using Escherichia coli Colloid. Surface. B 74 328 335 328–35 10.1016/j.colsurfb.2009.07.048
- SongK CLeeS MParkT SLeeB S 2009 Preparation of colloidal silver nanoparticles by chemical reduction method Korean. J. Chem. Eng. 26 153 155 153–5 10.1007/s11814-009-0024-y
- TamulyCHazarikaMBorahSDasM RBoruahM P 2013 In situ biosynthesis of Ag, Au and bimetallic nanoparticles using Piper pedicellatum C.DC: green chemistry approach Colloid. Surface. B 102 627 634 627–34 10.1016/j.colsurfb.2012.09.007
- CruzDFaleP LMouratoAVazP DSerralheiroM LLinoA R 2010 Preparation and physicochemical characterization of Ag nanoparticles biosynthesized by Lippia citriodora (Lemon Verbena) Colloid. Surface. B 81 67 73 67–73 10.1016/j.colsurfb.2010.06.025
- VigneshwaranNAshtaputreN MVaradarajanP VNachaneR PParalikarK MBalasubramanyaR H 2007 Biological synthesis of silver nanoparticles using the fungus Aspergillus flavus Mater. Lett. 61 1413 1418 1413–8 10.1016/j.matlet.2006.07.042
- SathiyanarayananGKiranG SSelvinJ 2013 Synthesis of silver nanoparticles by polysaccharide bioflocculant produced from marine Bacillus subtilis MSBN17 Colloid. Surface. B 102 13 20 13–20 10.1016/j.colsurfb.2012.07.032
- SumanT YRadhika RajasreeS RKanchanaAElizabethS B 2013 Biosynthesis, characterization and cytotoxic effect of plant mediated silver nanoparticles using Morinda citrifolia root extract Colloid. Surface. B 106 74 78 74–8 10.1016/j.colsurfb.2013.01.037
- MandalSPhadtareSSastryM 2005 Interfacing biology with nanoparticles Curr. Appl. Phys. 5 118 127 118–27 10.1016/j.cap.2004.06.006
- MariaB S 2014 Synthesis of silver nanoparticles using medicinal Zizyphus xylopyrus bark extract Appl. Nanosci. 10.1007/s13204-014-0372-8
- BerryC 2012 The bacterium, Lysinibacillus sphaericus, as an insect pathogen J. Invertebr. Pathol. 109 1 10 1–10 10.1016/j.jip.2011.11.008
- FayazA MBalajiKGirilalMYadavRKalaichelvanP TVenketesanR 2010 Biogenic synthesis of silver nanoparticles and their synergistic effect with antibiotics: a study against gram-positive and gram-negative bacteria Nanomedicine: Nanotechnol., Biol. Med. 6 103 109 103–9 10.1016/j.nano.2009.04.006
- LiongMAngelosSChoiEPatelKStoddartJ FZinkJ I 2009 Mesostructured multifunctional nanoparticles for imaging and drug delivery J. Mater. Chem. 19 6251 6257 6251–7 10.1039/b902462j
- OtsukaHNagasakiYKataokaK 2003 PEGylated nanoparticles for biological and pharmaceutical applications Adv. Drug. Deliver. Rev. 55 403 419 403–19 10.1016/S0169-409X(02)00226-0