Abstract
Until now, the real-time uptake and movement of manganese (Mn), an essential plant nutrient, has not been documented in plants. In this study, the real-time translocation of Mn in barley (Hordeum vulgare L. cv. Ehimehadaka no. 1) was visualized using the positron-emitting tracer 52Mn and a positron-emitting tracer imaging system (PETIS). PETIS allowed the non-destructive monitoring of Mn translocation in barley under various conditions. In all cases, 52Mn first accumulated in the discrimination center (DC) at the basal portion of the shoot, suggesting that this region may play an important role in Mn distribution in graminaceous plants. Manganese-deficient barley showed greater translocation of 52Mn from roots to shoots than did Mn-sufficient barley, demonstrating that Mn deficiency causes enhanced Mn uptake and loading into vascular bundles. In contrast, the translocation of 52Mn from roots to shoots was suppressed in Mn-excess barley. In these plants, the uptake of Mn may be suppressed or Mn may accumulate in the intercellular organelles of root cells, resulting in low rates of Mn translocation to shoots. In Mn-sufficient barley, the dark treatment did not suppress the translocation of 52Mn to the youngest leaf, suggesting that the translocation of Mn to the youngest leaf is independent of the transpiration stream. When 52Mn was supplied to the cut end of an expanded leaf, 52Mn was transported to the DC within 27 min and then retranslocated to roots and other leaves. Our results show that the translocation of Mn from the roots to the DC depends passively on water flow, but actively on the Mn transporter(s).
INTRODUCTION
Manganese (Mn) is an essential nutrient for plants and is required for a number of important cellular processes, including oxygen evolution during photosynthesis, detoxification of oxygen free radicals and CO2 fixation during C4 and Crassulacean acid metabolism (CAM) (CitationMarschner 1995). In soil, Mn is often present in forms that are unavailable to plants, particularly in high-pH soils, in which equilibrium conditions favor the oxidation of the plant-available Mn2+ to the unavailable Mn4+ (CitationWelch et al. 1991). When grown in such soils, crops may become Mn-deficient, resulting in reductions in growth and yield. In acidic and volcanic soils, Mn is reduced and, therefore, crop Mn toxicity is observed in many natural and agricultural systems (CitationCarver and Ownby 1995; CitationFoy et al. 1978).
Manganese is taken up by plants mainly in the form of Mn2+ ions (CitationFox and Guerinot 1998). Several genes have been reported to encode metal transporters that are able to transport Mn2+, such as OsNramp1, OsNramp3 (CitationBelouchi et al. 1997), AtNramp1, AtNramp3, AtNramp4 (CitationThomine et al. 2000), IRT1 (CitationEide et al. 1996; CitationKorshunova et al. 1999; CitationRogers et al. 2000) and AtOPT3 (CitationWintz et al. 2003). However, little is known about the translocation of Mn in intact plants after its absorption from roots. It has been proposed that Mn is transported to shoots via the xylem by the transpiration stream and root pressure, and then unloaded from the xylem into older leaves (CitationFisher 2000).
Recently, positron-emitting nuclides have been used in plants to study the distribution and translocation of 18F (CitationKume et al. 1997), [11C]methionine (CitationBughio et al. 2001; CitationNakanishi et al. 1999), 11C (CitationFujikake et al. 2003; CitationMatsuhashi et al. 2005), 13NO− 3 (CitationHayashi et al. 1997; CitationMatsunami et al. 1999; CitationOhtake et al. 2001), 13N (CitationKiyomiya et al. 2001b), H15 2O (CitationKiyomiya et al. 2001a; CitationMori et al. 2000; CitationNakanishi et al. 2002; CitationTsukamoto et al. 2004) and 52Fe, 52Mn and 62Zn (CitationWatanabe et al. 2001) using a positron-emitting tracer imaging system (PETIS). With this technique, γ-rays produced by positron-emitting nuclides are detected by scintillation detectors, allowing the real-time investigation of the movement of elements in intact plants. We have also examined the transport of 59Fe in barley (Hordeum vulgare L. cv. Ehimehadaka no. 1) using 59Fe(III)-epihydroxymugineic acid (epiHMA) and autoradiography detection (CitationMori 1998). To compare the translocation of 52Mn with the translocation of 59Fe and other positron-emitting nuclides, we initiated a study using barley plants. We have already reported the production of the metallic positron-emitting nuclides 52Mn, 52Fe and 62Zn and their potential use in PETIS (CitationWatanabe et al. 2001), but no physiological studies of metal translocation in plants have been reported. In the present study, we used PETIS to monitor the real-time translocation of 52Mn in intact barley plants under various conditions for the first time, and calculated the velocity of 52Mn translocation.
MATERIALS AND METHODS
Plant materials
Barley (Hordeum vulgare L. cv. Ehimehadaka no. 1) seeds were grown hydroponically in modified Kasugai's medium: 0.7 mmol L−1 K2SO4, 0.1 mmol L−1 KCl, 0.1 mmol L−1 KH2PO4, 2.0 mmol L−1 Ca(NO3)2, 0.5 mmol L−1 MgSO4, 10 µmol L−1 H3BO3, 0.5 µmol L−1 MnSO4, 0.2 µmol L−1 CuSO4, 0.5 µmol L−1 ZnSO4, 0.01 µmol L−1 (NH4)Mo6O24, and 0.1 µmol L−1 Fe-EDTA as described previously (CitationMori and Nishizawa 1987). Manganese-deficient plants were generated by culturing in a medium lacking Mn for 2 weeks prior to beginning the 52Mn-absorption experiments. Manganese-excess plants were produced by culturing in medium containing 100 µmol L−1 Mn for 1 week before beginning the absorption experiments. All absorption experiments were conducted approximately 3 weeks after germination, and the sizes and root weights of plants of both types were almost the same.
Production of 52Mn
52Mn (half-life 5.59 days) was produced by the natCr(p, xn)52Mn reaction, in which a 1.5-mm-thick chromium foil (natural isotopic composition, 99.9% purity; Goodfellow Metals, Cambridge, UK) is bombarded with a 20-MeV proton beam (generated by the Takasaki Ion Accelerators for Advanced Radiation Application [TIARA] AVF cyclotron; Gunma, Japan; CitationWatanabe et al. 2001). Approximately 5 MBq of 52Mn was produced using a beam current of 1 µA for 2.4 h. 52Mn was radiochemically separated from the target using the method described by CitationWatanabe et al. (2001).
PETIS and PMPS
Plant samples were placed between two opposed 2-D block detectors consisting of Bi4Ge3O12 scintillator arrays. The 52Mn produced was used as a positron-emitting tracer. Two annihilation γ-rays emitted from the decaying positrons were detected simultaneously (CitationKume et al. 1997; CitationUchida et al. 2004). The original position of the annihilation was localized at the intersection of the object plane determined by a line connecting the two detection points on the detectors. The field of view was 143 mm × 215.6 mm, and the spatial resolution was 2.4 mm. After automatic correction for decay and relative detection efficiencies within the field of view, the resulting image was displayed on a monitor. After the imaging procedure was completed, the regions of interest (ROIs; , ) within the data obtained were set and the radioactivity behavior over time within each ROI was extracted from the data. Two pairs of positron multi-probe system (PMPS) detectors were used for time-course tracer analysis of 52Mn. Although the principles of PMPS are similar to those of PETIS, PMPS detectors directly measure the radioactivity time-course but bypass the imaging procedure.
Absorption and translocation of 52Mn in plants
To study the absorption of 52Mn by roots, the roots of a single plant were placed in a polyethylene bag containing 15 mL of 5× modified Kasugai's solution lacking Mn. To maintain the geometry, the plant and the bag were placed between two acrylic boards centered between the PETIS detectors in a chamber at 25°C, 65% humidity, and with a light density of 320 µmol m−2 s−1. 52Mn2+ (250–900 kBq) in 1 mL water was added to the culture with gentle aeration for immediate mixing. The PETIS detectors were focused on the basal part of the shoot. After a 6–10 h trace analysis, the plant was removed from the polyethylene bag and the roots were gently washed for 1 min in 50 mL 5× modified Kasugai's solution. Next, the plant was exposed to a bio-imaging analyzer system (BAS) imaging plate (Imaging Plate BAS-MP2040S, Fujifilm, Tokyo, Japan) to obtain an autoradiographic image. After 30 min, the plate was scanned using a bio-imaging analyzer system (BAS-1500, Fujifilm, Tokyo, Japan). In the dark-treatment experiment, the plants were cut into parts after the PETIS and autoradiography analyses. The radioactivity of each part was then determined by γ-ray
Figure 1 (a,b) 52Mn translocation from the roots of (a) Mn-deficient and (b) Mn-sufficient (control) barley plants. Images were obtained by autoradiography. Rectangles show the area measured by the positron-emitting tracer imaging system (PETIS). DC, discrimination center; YL, youngest leaf. Scale bars are 4 cm. These images cannot be compared to each other. (c) Time-course of radioactivity in Mn-deficient (left) and control (right) barley as measured by PETIS. The images were taken at 15-min and 2-h intervals (0–120 min and 2–8 h, respectively). Arrowheads indicate the DC. Scale bars are 1 cm. (d) Time-course of radioactivity in the DC of Mn-deficient (purple dots) and control (orange dots) barley. The maximum measured radioactivity was defined as 100, and all measurements were converted relative to the maximum. (e) Time-course of radioactivity in the DC and at a position 3.41 cm above the DC in the main shoot of Mn-deficient barley (indicated as “shoot” in [c]). Arrows indicate the arrival time of 52Mn. The arrival time was defined as the time at which radioactivity began to be detected.
![Figure 1 (a,b) 52Mn translocation from the roots of (a) Mn-deficient and (b) Mn-sufficient (control) barley plants. Images were obtained by autoradiography. Rectangles show the area measured by the positron-emitting tracer imaging system (PETIS). DC, discrimination center; YL, youngest leaf. Scale bars are 4 cm. These images cannot be compared to each other. (c) Time-course of radioactivity in Mn-deficient (left) and control (right) barley as measured by PETIS. The images were taken at 15-min and 2-h intervals (0–120 min and 2–8 h, respectively). Arrowheads indicate the DC. Scale bars are 1 cm. (d) Time-course of radioactivity in the DC of Mn-deficient (purple dots) and control (orange dots) barley. The maximum measured radioactivity was defined as 100, and all measurements were converted relative to the maximum. (e) Time-course of radioactivity in the DC and at a position 3.41 cm above the DC in the main shoot of Mn-deficient barley (indicated as “shoot” in [c]). Arrows indicate the arrival time of 52Mn. The arrival time was defined as the time at which radioactivity began to be detected.](/cms/asset/9500275f-15f5-4ab8-bfb0-6a4439038958/tssp_a_10382448_o_f0001g.gif)
Figure 2 (a,b) 52Mn translocation from the roots of (a) Mn-excess and (b) control barley. Images were obtained by autoradiography. Rectangles show the area measured by the positron-emitting tracer imaging system (PETIS). Scale bars are 4 cm. These images cannot be compared to each other. (c) Time-course of radioactivity in Mn-excess (left) and control (right) barley as measured by PETIS. The images were taken at 1-h intervals. DC, discrimination center. Scale bars are 1 cm. (d) Time-course of radioactivity in the DC of Mn-excess (purple dots) and control (orange dots) barley. The maximum measured radioactivity was defined as 100, and all measurements were converted relative to the maximum.
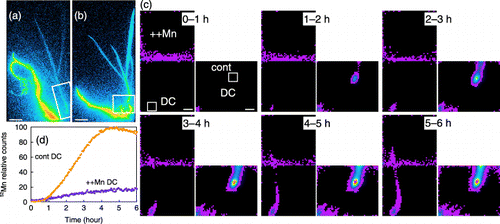
To study the translocation of 52Mn from a leaf, the second youngest leaf was used because this leaf is the longest. The leaf tip was cut in distilled water to prevent the introduction of air into the exposed leaf tissues. The cut end was then submerged in 3 mL of 5× modified Kasugai's solution lacking Mn in a vial. 52Mn2+ (1.88 MBq) in 1 mL water was added to the vial, and the PMPS detectors were focused on the basal part of the shoot. After a 6-h trace analysis, autoradiography was carried out.
Each experiment was repeated at least three times to confirm the reproducibility of the results.
RESULTS
Mn2+ translocation in Mn-deficient and Mn-sufficient barley plants
To examine the effect of Mn deficiency on the absorption and translocation of Mn in intact plants, 52Mn2+ was supplied to the roots of both Mn-deficient and Mn-sufficient (control) barley plants, and the translocation of 52Mn was monitored using PETIS and autoradiography (). Autoradiography images showed that the discrimination center (DC), which is located in the basal part of the shoot, was strongly labeled in both Mn-deficient and control plants (). In Mn-deficient barley, the youngest leaf was the most strongly labeled shoot (). shows a montage of PETIS images. The radioactivity observed in images of shoots of Mn-deficient barley was higher than that of control barley shoots in a 10-h experiment, showing that more 52Mn was translocated to shoots in Mn-deficient barley than in control barley. In Mn-deficient barley, an image of the DC (, arrowhead) appeared within 75–90 min, and an image of the shoots appeared within 2–4 h (). In contrast, in control barley, images of the DC and shoots appeared within 90–105 min and 4–6 h, respectively. The time-course of radioactivity in the DCs (indicated in , squares) was extracted from the PETIS data (). During the 10-h experiment, the 52Mn counts in the DCs of Mn-deficient barley were higher than those in the DCs of control barley. This result shows that Mn accumulated in the DC in higher amounts in Mn-deficient barley than in control barley. The times of arrival of 52Mn to the DC and the main part of the shoot (indicated as “shoot” in , 3.41 cm above the DC) in Mn-deficient barley were calculated using the results of the time-course of 52Mn radioactivity. The accumulation of 52Mn in the DCs of Mn-deficient barley began at 42 min, and in the main shoot at 99 min (, arrows). Therefore, the speed of 52Mn translocation from the DCs to the main shoots of Mn-deficient barley was 0.060 cm min−1 (= 3.41 cm/[99 − 42] min). It was not possible to calculate the 52Mn translocation speed in control barley because of the very low levels of 52Mn transport to shoots.
Mn2+ translocation in Mn-excess barley
52Mn2+ was supplied to the roots of Mn-excess and Mn-sufficient (control) barley plants, and images of the shoots (indicated in , rectangles) were captured. The montage images revealed the radioactivity of 52Mn translocated to the shoots of Mn-excess and control barley in a 6-h experiment (). The radioactivity recorded in images of shoots of Mn-excess barley was lower than that in control barley shoots. This result shows that Mn was translocated to the shoots in lower amounts in Mn-excess barley than in control barley under these experimental conditions. The time-course of 52Mn radioactivity in the DCs (, squares) of Mn-excess and control barley showed that the DCs of Mn-excess barley accumulated approximately 20% of the 52Mn accumulated by the DCs of control plants in a 6-h experiment ().
Effect of light on Mn translocation
The effect of light on 52Mn translocation was examined by carrying out the same experiment in darkness. The time-course of 52Mn radioactivity in the DC (, squares) was extracted from the PETIS data (). In a 10-h experiment, the 52Mn counts in the DC were lower in darkness than in the light, showing that darkness suppressed 52Mn translocation to the DC. The 52Mn content of the youngest leaf (the fifth leaf) after 10 h of absorption was not suppressed in darkness, but the 52Mn content of older leaves (fourth, third and second leaves) decreased ().
Mn2+ translocation from a leaf
The translocation of Mn from a single leaf of an intact plant was examined by supplying 52Mn2+ to the cut end of the second youngest leaf of an Mn-sufficient barley plant. 52Mn was translocated from the cut end of the second-youngest leaf to the DC, other leaves and roots (). The DC and root tips were particularly strongly labeled. The time-course of radioactivity in the DC revealed that accumulation of 52Mn in the DC began at
Figure 3 (a,b) 52Mn translocation from the roots of control barley in (a) darkness and (b) light. Images were obtained by autoradiography. Leaf numbering: 4, the second-youngest leaf on the main shoot; 3, the third leaf; 2, the second leaf; 1, the first leaf. The youngest leaf, the fifth leaf, is present inside the leaf sheath. DC, discrimination center. Scale bars are 4 cm. (c) Time-course of radioactivity in the DC (as marked in [a,b]) of barley in darkness (•) and light (○). The maximum measured radioactivity was defined as 100, and all measurements were converted relative to the maximum. (d) 52Mn content in the DC and leaves of barley in darkness (▪) and light (□).
![Figure 3 (a,b) 52Mn translocation from the roots of control barley in (a) darkness and (b) light. Images were obtained by autoradiography. Leaf numbering: 4, the second-youngest leaf on the main shoot; 3, the third leaf; 2, the second leaf; 1, the first leaf. The youngest leaf, the fifth leaf, is present inside the leaf sheath. DC, discrimination center. Scale bars are 4 cm. (c) Time-course of radioactivity in the DC (as marked in [a,b]) of barley in darkness (•) and light (○). The maximum measured radioactivity was defined as 100, and all measurements were converted relative to the maximum. (d) 52Mn content in the DC and leaves of barley in darkness (▪) and light (□).](/cms/asset/7ec94e14-5200-4d65-a76a-2ccd242bff0c/tssp_a_10382448_o_f0003g.gif)
DISCUSSION
Accumulation of minerals and metabolites in the DC of graminaceous plants
The time-course of 52Mn translocation was traced in intact barley using PETIS, and the speed of 52Mn translocation was then calculated. This is the first report on the real-time imaging of Mn uptake and translocation in intact plants. In all experiments, 52Mn primarily accumulated in the DC, a region that contains the shoot meristem (CitationMori 1998). 52Mn first accumulated in the DC and was then distributed to all plant tissues (). A similar result was reported in wheat (Triticum aestivum cv. Aroona) using 54Mn (CitationPearson and Rengel 1995). Similarly, we reported that radioactivity accumulated in the DCs of barley and rice and was then distributed to shoots, as determined in studies using 11C-methionine (CitationBughio et al. 2001; CitationNakanishi et al. 1999), 13NH+ 4 (CitationKiyomiya et al. 2001a) and H15 2O (CitationKiyomiya et al. 2001b; CitationMori et al. 2000; CitationNakanishi et al. 2002; CitationTsukamoto et al. 2004). Therefore, it is likely that not only Mn but also other minerals and metabolites accumulate in the DC after absorption from the roots of graminaceous plants. Taken together, these data suggest that the DC may play an important role in distributing minerals and metabolites in graminaceous plants.
The speed of 52Mn translocation from the DC to shoots in barley was approximately 0.060 cm min−1 (). In other studies in which the translocation of other nutrients was examined in barley and rice, the transport rates from the DCs to shoots were 1 cm min−1 (11C-methionine; CitationBughio et al. 2001; CitationNakanishi et al. 1999), 8.6 cm min−1 (13N-glutamine; CitationKiyomiya et al. 2001a) and 1.9–1.1 cm min−1 (H15 2O; CitationKiyomiya et al. 2001b). Therefore, the speed of translocation of the substrate depends on the substrate itself. The translocation of 52Mn from the DC to shoots in Mn-deficient barley (0.060 cm min−1) was slower than the translocation of H15 2O, 11C-methionine or 13N-glutamine, which suggests that substrates that are required for plant growth in large quantities are translocated more rapidly than those that are required in small quantities.
Effects of Mn status on Mn absorption and translocation from roots
More 52Mn was translocated to shoots in Mn-deficient barley than in Mn-sufficient barley (), suggesting that the demand for Mn affects its translocation. Similarly, after labeling a single wheat root with 54Mn for 2 h, the main stem, leaves and tillers (other than the oldest leaf) of low-Mn plants received more 54Mn than the control plants (CitationPearson and Rengel 1995). These results are consistent with our data. In addition, an image of the
Figure 4 (a) 52Mn translocation from a leaf tip (arrowhead) to other tissues in a control barley plant. Images were obtained by autoradiography. Leaf numbering: 4, the youngest leaf on the main shoot; 3, the third leaf; 2, the second leaf; 1-2, 1-1, younger and older leaf on the first tiller, respectively. Scale bar is 4 cm. (b) Time-course of radioactivity in the discrimination center (DC). The maximum measured radioactivity was defined as 100, and all measurements were converted relative to the maximum.
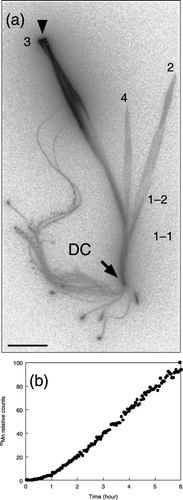
52Mn was translocated preferentially to younger leaves in Mn-deficient barley (), and the DC may play a critical role in this process. Similarly, when 59Fe-epiHMA was supplied to barley roots, 59Fe was also translocated preferentially to younger leaves (CitationMori 1998). Manganese may be translocated to younger leaves through a pathway similar to that of Fe. Although the expression of an Mn transporter in barley shoots has not been reported, a number of Mn or Mn-chelate complex transporters that are expressed in shoots of other plant species, including AtNramp3, AtNramp4 (CitationThomine et al. 2000), AtOPT3 (CitationStacey et al. 2002), MtZIP4, MtZIP7 (CitationLópez-Millán et al. 2004), and OsYSL2 (CitationKoike et al. 2004), have been reported. MtZIP4, MtZIP7 and OsYSL2 are not induced by Mn deficiency, and the expression of AtNramp3, AtNramp4 and AtOPT3 does not appear to be regulated by Mn deficiency. Genes encoding Mn transporters may be induced in the DC during Mn deficiency, and the resulting enhanced Mn transport may lead to the regulation of Mn distribution in plants.
In Mn-excess barley, less 52Mn was translocated to shoots than in control barley (). Excess Fe and cadmium have been shown to inhibit Mn2+ accumulation (CitationHernandez et al. 1998; CitationRoomizadeh and Karimian 1996). It has also been reported that transporters such as the Arabidopsis CAX2 (CitationHirschi et al. 2000), ECA1 (CitationWu et al. 2002) and ShMTP1 (CitationDelhaize et al. 2003) pump Mn2+ into internal organelles to prevent the toxic effects of excess Mn2+ and are able to promote Mn tolerance under conditions of Mn toxicity. In the present study, radioactivity resulting from 52Mn taken up into root cells of Mn-excess and control barley could not be measured because it is impossible to distinguish 52Mn inside the cell from 52Mn in the apoplastic space and cell wall. Therefore, it is possible that the absorption of 52Mn is suppressed by excess Mn, or that more 52Mn accumulates in root cells of Mn-excess barley than in the control.
Effects of light on Mn translocation
Interestingly, the translocation of 52Mn to the youngest leaf was not suppressed in darkness (), whereas 52Mn translocation to the older leaves was suppressed. We previously reported that the flow of H15 2O was immediately and completely stopped by a dark treatment during 30 min of absorption, suggesting that the transpiration stream in the xylem is completely inhibited in darkness (CitationKiyomiya et al. 2001b; CitationMori et al. 1999). This finding indicates that the transpiration stream has little effect on Mn translocation to the youngest leaf, but a strong effect on Mn translocation to older leaves. CitationTanner and Beevers (2001) reported that when transpiration was experimentally dissociated from the mineral supply of sunflowers (Helianthus annuus) grown in hydroculture, and mineral nutrients were provided only at night, the growth of the plants was similar to that of the control group, which received nutrients only during the day. They concluded that convective water transport in the xylem, brought about by root pressure, the resultant guttation called “growth water” and Münch's phloem counterflow, is sufficient for long-distance mineral supply and that transpiration is not required for this function. Similarly, the translocation of mineral nutrients, including Mn, to the youngest leaf appears to proceed regardless of the activity of the transpiration stream.
Mn2+ translocation from a barley leaf
When 52Mn was supplied to the cut end of an expanded leaf, 52Mn was transported to the DC within 25 min and then retranslocated to roots and other leaves (). In particular, 52Mn was preferentially translocated to strong sink organs, such as younger leaves and root tips. Similarly, CitationVose (1963) reported that 54Mn was translocated to the stem, the youngest leaf and the roots when applied as a drop to the fourth leaves of Mn-deficient oat plants whose flag leaves had emerged. This author also suggested that the root can function as a reservoir of available Mn. We reported the transport of 59Fe in barley using 59Fe(III)-epiHMA (CitationMori 1998). When 59Fe-epiHMA was supplied to cut barley leaves, 59Fe was translocated through the DC to other new chlorotic leaves and root tips within 45 min, as detected by autoradiography. The translocation of 52Mn from shoots to roots showed similar characteristics to the transport of 59Fe. It has been observed that many Mn2+ transporters have broad substrate specificities and some transporters are also capable of transporting not only Mn2+ but also Fe2+ and other nutrients (CitationPittman 2005). Therefore, the similar translocation of 52Mn and 59Fe from shoots to roots may be caused by transporters that are capable of transporting both Mn2+ and Fe2+. In contrast, we reported that 11C-methionine is not translocated from the leaves to the roots of Fe-deficient barley (CitationNakanishi et al. 1999). These results indicate that not all minerals and metabolites are translocated from the leaves to the roots, suggesting that translocation from shoots to roots does not proceed through passive diffusion but is instead actively regulated, and the DC may contribute to this regulation.
ACKNOWLEDGMENTS
This research was supported by the Universities and Japan Atomic Energy Research Institute (JAERI) Joint Research Project. The Research Centre for Nuclear Science and Technology, University of Tokyo, is also acknowledged. We express our sincere regrets for the loss of S. Kiyomiya, the third author, who passed away on 16 August 2001.
REFERENCES
- Marschner , H . 1995 . Mineral Nutrition of Higher Plants , 2nd edn , London : Academic Press .
- Welch , RM , Allaway , WH , House , WA and Kubota , J . 1991 . “ Geographic distribution of trace element problems ” . In Micronutrients in Agriculture , 2nd edn , Edited by: Mortvelt , JJ , Cox , FR , Shuman , LM and Welch , RM . 31 – 57 . Madison : Soil Science Society of America .
- Carver , BF and Ownby , JD . 1995 . Acid soil tolerance in wheat . AdvAgron , 54 : 117 – 173 .
- Foy , CD , Chaney , RL and White , MC . 1978 . The physiology of metal toxicity in plants . AnnuRevPlant Physiol , 29 : 511 – 566 .
- Fox , TC and Guerinot , ML . 1998 . Molecular biology of cation transport in plants . AnnuRevPlant Physiol. PlantMolBiol , 49 : 669 – 696 .
- Belouchi , A , Kwan , T and Gros , P . 1997 . Cloning and characterization of the OsNrampfamily from Oryza sativa, a new family of membrane proteins possibly implicated in the transport of metal ions . Plant MolBiol , 33 : 1085 – 1092 .
- Thomine , S , Wang , R , Ward , JM , Crawford , NM and Schroeder , JI . 2000 . Cadmium and iron transport by members of a plant metal transporter family in Arabidopsiswith homology to Nrampgenes . PNAS , 97 : 4991 – 4996 .
- Eide , D , Broderius , M , Fett , J and Guerinot , ML . 1996 . A novel iron-regulated metal transporter from plants identified by functional expression in yeast . PNAS , 93 : 5624 – 5628 .
- Korshunova , YO , Eide , D , Clark , WG , Guerinot , ML and Pakrasi , HB . 1999 . The IRT1 protein from Arabidopsis thalianais a metal transporter with a broad substrate range . Plant MolBiol , 40 : 37 – 44 .
- Rogers , EE , Eide , DJ and Guerinot , ML . 2000 . Altered selectivity in an Arabidopsismetal transporter . PNAS , 97 : 12 356 – 12 . 360
- Wintz , H , Fox , T Wu , YY . 2003 . Expression profiles of Arabidopsis thalianain mineral deficiencies reveal novel transporters involved in metal homeostasis . JBiolChem , 278 : 47 644 – 47 . 653
- Fisher , DB . 2000 . “ Long-distance transport ” . In Biochemistry and Molecular Biology of Plants , Edited by: Buchanan , BB , Gruissem , W and Jones , RL . 730 – 784 . Rockville : American Society of Plant Biologists .
- Kume , T , Matsuhashi , S Shimazu , M . 1997 . Uptake and transport of positron-emitting tracer (18F) in plants . ApplRadiatIsotopes , 48 : 1035 – 1043 .
- Bughio , N , Nakanishi , H Kiyomiya , S . 2001 . Real time [11C]methionine translocation in barley in relation to mugineic acid phytosiderophore biosynthesis . Planta , 213 : 708 – 715 .
- Nakanishi , H , Bughio , N Matsuhashi , S . 1999 . Visualizing real time [11C]methionine translocation in Fe-sufficient and Fe-deficient barley using a positron-emitting tracer imaging system (PETIS) . JExpBot , 50 : 637 – 643 .
- Fujikake , H , Yamazaki , A Ohtake , N . 2003 . Quick and reversible inhibition of soybean root nodule growth by nitrate involves a decrease in sucrose supply to nodules . JExpBot , 54 : 1379 – 1388 .
- Matsuhashi , S , Fujimaki , S , Kawachi , N , Sakamoto , K , Ishioka , NS and Kume , T . 2005 . Quantitative modeling of photoassimilate flow in an intact plant with the Positron Emitting Tracer Imaging System (PETIS) . Soil SciPlant Nutr , 51 : 417 – 423 .
- Hayashi , H , Okada , Y Mano , H . 1997 . Detection and characterization of nitrogenncirculation through the sieve tubes and xylem vessels of rice plants . Plant Soil , 196 : 233 – 237 .
- Matsunami , H , Arima , Y Watanabe , K . 1999 . Positional relationship between 13N-nitrate and rizobium infective site on a signal roots of common bean and soybean: An application of positron emitting tracer imaging system . Soil SciPlant Nutr , 45 : 955 – 962 .
- Ohtake , N , Sato , T Hujitake , H . 2001 . Rapid N transport to pods and seeds in N-deficient soybean plants . JExpBot , 52 : 277 – 283 .
- Kiyomiya , S , Nakanishi , H Uchida , H . 2001b . Light activates H15 2O flow in rice: Detailed monitoring using a positron-emitting tracer imaging system (PETIS) . PhysiolPlant , 113 : 359 – 367 .
- Kiyomiya , S , Nakanishi , H Uchida , H . 2001a . Real time visualization of 13N-translocation in rice under different environmental conditions using positron-emitting tracer imaging system (PETIS) . Plant Physiol , 125 : 1743 – 1754 .
- Mori , S , Kiyomiya , S Nakanishi , H . 2000 . Visualization of 15O-water flow in tomato and rice in the light and dark using a positron-emitting tracer imaging system (PETIS) . Soil SciPlant Nutr , 46 : 975 – 979 .
- Nakanishi , H , Kiyomiya , S , Tsukamoto , T , Tsukada , H , Uchida , H and Mori , S . 2002 . Water (H15 4O) flow in rice is regulated by the concentration of nutrient as monitored by positron multi-probe system (PMPS) . Soil SciPlant Nutr , 48 : 759 – 762 .
- Tsukamoto , T , Uchida , H Nakanishi , H . 2004 .
- Watanabe , S , Ishioka , NS Osa , A . 2001 . Production of positron emitters of metallic elements to study plant uptake and distribution . RadiochimActa , 89 : 853 – 858 .
- Mori , S . 1998 . “ Iron transport in graminaceous plants ” . In Iron Transport and Storage in Microorganisms, Plants and Animals, Vol 35, Metal Ions in Biological Systems , Edited by: Sigel , A and Sigel , H . Vol 35 , 215 – 237 . New York : Marcel Dekker .
- Mori , S and Nishizawa , KN . 1987 . Methionine as a dominant precursor of phytosiderophores in Graminaceae plants . Plant Cell Physiol , 28 : 1081 – 1092 .
- Uchida , H , Okamoto , T Ohmura , T . 2004 . A compact planar positron imaging system . NuclInstrumMethA , 516 : 564 – 574 .
- Pearson , JN and Rengel , Z . 1995 . Uptake and distribution of 65Zn and 54Mn in wheat grown at sufficient and deficient levels of Zn and Mn I. During vegetable growth . JExpBot , 46 : 833 – 839 .
- Stacey , MG , Koh , S , Becker , J and Stacey , G . 2002 . AtOPT3, a member of the ologopeptide transporter family, is essential for embryo development in Arabidpsis . Plant Cell , 14 : 2799 – 2811 .
- López-Millán , AF , Ellis , DR and Grusak , MA . 2004 . Identification and characterization of several new members of the ZIP family of metal ion transporters in Medicago truncatula . Plant MolBiol , 54 : 583 – 596 .
- Koike , S , Inoue , H Mizuno , D . 2004 . OsYSL2 is a rice metal-nicotianamine transporter that is regulated by iron and expression in the phloem . Plant J , 39 : 415 – 424 .
- Hernandez , LE , Lozano-Rodriguez , E , Garate , A and Carpena-Ruiz , R . 1998 . Influence of cadmium on the uptake, tissue accumulation and subcellular distribution of manganese in pea seedlings . Plant Sci , 132 : 139 – 151 .
- Roomizadeh , S and Karimian , N . 1996 . Manganese–iron relationship in soybean grown in calcareous soils . JPlant Nutr , 19 : 397 – 406 .
- Hirschi , KD , Korenkov , VD , Wilganowski , NL and Wagner , GJ . 2000 . Expression of Arabidopsis CAX2in tobacco. Altered metal accumulation and increased manganese tolerance . Plant Physiol , 124 : 125 – 134 .
- Wu , Z , Liang , F Hong , B . 2002 . An endoplasmic reticulum-bound Ca2+/Mn2+pump, ECA1, supports plant growth and confers tolerance to Mn2+stress . Plant Physiol , 130 : 128 – 137 .
- Delhaize , E , Kataoka , T , Hebb , DM , White , RG and Ryan , RR . 2003 . Genes encoding proteins of cation diffusion facilitator family that confer manganese tolerance . Plant Cell , 15 : 1131 – 1142 .
- Tanner , W and Beevers , H . 2001 . Transpiration, a prerequisite for long-distance transport of minerals on plants? . PNAS , 98 : 9443 – 9447 .
- Vose , PB . 1963 . The translocation and redistribution of manganese in Avena . JExpBot , 14 : 448 – 457 .
- Pittman , JK . 2005 . Managing the manganese: molecular mechanisms of manganese transport and homeostasis . New Phytol , 167 : 733 – 742 .
- Tsukamoto , T , Uchida , H and Nakanishi , H . 2004 . H15 4O translocation in rice was enhanced by 10 µM 5-aminolevulinic acid as monitored by positron emitting tracer imaging system (PETIS) . Soil SciPlant Nutr , 50 : 1085 – 1088 .