Abstract
In the present study, characterization of greenhouse gas emissions, such as CO2, CH4 and N2O, from a multi-soil-layering (MSL) system during wastewater treatment was conducted, using a laboratory scale system (width 50 × depth 10 × height 120 cm). Net fluxes of CO2, CH4 and N2O from the system were in the range of 21.2–48.0 g m−2 day−1, −66.4–0.02 mg m−2 day−1 and 41–246 mg m−2 day−1, respectively. Carbon dioxide emission generally increased as the air temperature increased. The net flux of CO2 was not appreciably different among the treatments, even though the hydraulic loading rates (HLR) differed widely. These findings indicated that the decomposition of organic matter loaded through wastewater was more efficient in the low HLR treatments. Mean CH4 emission was lower than the amount of CH4 brought by the influent. Methane was consumed in the MSL system during most of the study period, and the consumption tended to be more efficient in the treatments at high HLRs. Wastewater quality was not markedly related to the characteristics of the CO2 and CH4 emissions. Nitrous oxide was mainly released into the treated water as dissolved gas. Nitrous oxide emission per 1,000 L of wastewater decreased as the HLRs increased in the wastewater containing a high level of contaminants (HWW), while in the case of wastewater containing a low level of contaminants (LWW), such a decrease in the emission was not observed. Mean N2O conversion rates of the LWW treatments ranged from 1.10 to 1.79% and were higher than those of the HWW treatments, which ranged from 0.19 to 1.95%. Nitrous oxide emission could be reduced in the HWW treatments.
INTRODUCTION
The characteristics and results of various types of wastewater treatments using a multi-soil-layering (MSL) system have been reported in several papers (CitationLuanmanee et al. 2002; CitationMasunaga et al. 2002a, Citation2003; CitationWakatsuki et al. 1993). These authors reported extensively the characteristics of the MSL system and its applicability to various types of wastewater or polluted water treatments. However, the mechanisms of wastewater treatment inside the MSL system have not yet been fully elucidated, although attempts had been made in a number of recent papers (CitationMasunaga et al. 2002b; CitationSato et al. 2002a; CitationSato 2005a,Citationb). The fate of the contaminants removed in the MSL system has also not been fully clarified. While phosphorus was absorbed in the MSL system, organic matter and nitrogen were reported to be decomposed and released from the system (CitationLuanmanee et al. 2001; CitationSato et al. 2002b). However, these authors did not determine the forms of the decomposed organic matter and nitrogen. Transformation of organic matter and nitrogen during wastewater treatment could lead to the release of greenhouse gases such as CH4 and N2O (CitationCzepiel et al. 1995; CitationIntergovernmental Panel on Climate Change 1995). Therefore, because domestic wastewater and its treatment process play an important role in various sources of greenhouse gases, there has been a growing concern about greenhouse gas emissions from wastewater treatment systems. As large quantities of carbon and nitrogen flow into domestic wastewater treatment systems, significant amounts of CH4 and N2O emissions are likely to occur. Emissions of these greenhouse gases vary with the treatment conditions in sewage treatment plants based on the active sludge method (CitationItokawa et al. 1996; CitationKimochi et al. 1998). However, there have been very few studies on greenhouse gas emissions from the soil trench system, which is a soil-based wastewater treatment system (CitationKong et al. 2002), although there are several reports on greenhouse gas emissions from reclaimed effluent applied to the soil (i.e. secondary treated sewage) (CitationMaster et al. 2003). As the amount of wastewater discharged into a MSL system is usually 10–100-fold higher than that in the soil trench system or the reclaimed effluent applied to land, it can be assumed that the characteristics of greenhouse gas emissions from a MSL system will be different.
The purpose of the present study was to characterize and evaluate greenhouse gas emissions, such as CO2, CH4 and N2O, from a MSL system during wastewater treatment. Characterization of the removal efficiency of contaminants, such as organic matter, nitrogen and phosphorus, has been reported in a previous paper using the same wastewater treatment experiments (CitationMasunaga et al. 2007).
MATERIALS AND METHODS
Description of the experimental conditions
shows the structure of the laboratory-scale MSL system used in the present study. Acrylic boxes, height 139 cm × width 50 cm × depth 10 cm in size, were prepared. The MSL system consisted of soil mixture layers and zeolite layers with a diameter of 1–3 mm. The soil mixture layers were composed of volcanic ash soil rich in organic matter classified into Andisol (CitationUnited States Department of Agriculture (USDA) 1999), sawdust and granular metal iron at a volume ratio of 75%, 12.5% and 12.5%, respectively. These layers were 8 cm thick and were covered with a 2 cm thick layer of powder charcoal and were arranged in a brick-layer-like pattern surrounded by zeolite layers. The top of the MSL system was fully covered by an acrylic chamber, height 20 cm × width 50 cm × depth 10 cm, 10,000 cm3 in size for the collection of the greenhouse gases emitted from the system to the atmosphere. The chamber was closed only during the period of gas sampling. Although two aeration pipes were installed in the system, aeration was not applied during this experiment.
Domestic wastewater from a housing development was diluted (8 or 4-fold) with well water. These two types of wastewater contained relatively low or high levels of contaminants and were hereafter designated as LWW and HWW, respectively. The mean properties of LWW and HWW were as follows: 32.1 and 69.5 mg L−1 for Biochemical Oxygen Demand (BOD), 6.3 and 9.6 mg L−1 for the total nitrogen content (T-N) and 0.76 and 1.47 mg L−1 for the total phosphorus content (T-P). Detailed data were presented in table 1 of our previous paper (CitationMasunaga et al. 2007). Each type of wastewater was discharged into the MSL system continuously for 24 h at hydraulic loading rates (HLRs) of 500, 1,000, 1,250, 1,500 and 2,000 L m−2 day−1 using tube pumps (IWAKI PST-1120, Asahi Techno Glass Co Ltd, Tokyo, Japan). Therefore, the number of total treatments was 10 with a combination of five HLRs and two grades of wastewater. Hereafter, the treatments are designated as L-500 to L-2000 and H-500 to H-2000. During the 14-month period of the wastewater treatment experiment, clogging occurred during some of the treatments. At that time, wastewater discharge into the clogged system was interrupted for 1–2 or 8 weeks for recovery. Details on the clogging were described in our previous paper (CitationMasunaga et al. 2007).
Sampling and analyses
The emissions of the greenhouse gases CO2, CH4 and N2O released into the atmosphere (EA) were determined using a static chamber technique from the top of the system. A 200-mL gas sample was placed into a 1-L Tedlar bag at 0 and 30 min for CO2 and CH4 and at 0, 1, 2 and 3 h for N2O after the chamber lid was closed. The gas emission was calculated based on the changes in the gas concentration in the chamber in relation to the closure time.
The dissolved gas (CO2, CH4 and N2O) concentrations in both the wastewater and the treated water were determined using a headspace equilibration technique (CitationLiikanen et al. 2002; CitationSenga et al. 2001). The gas emission from treated water (ET) was calculated as the product of the dissolved gas concentration and the volume of the treated water released from the system. For the determination of dissolved CO2 and CH4 concentrations, a 25-mL water sample was introduced into a 60-mL plastic syringe equipped with a silicon stopper without any gas-phase, and 1 mL of a 0.1 mol L−1 HgCl2 solution was immediately added to the sample to stop biological activity. Then 1 mL of a 0.5 mol L−1 H2SO4 solution was added to lower the pH and to convert all the aqueous carbonate species to H2CO3. Twenty-five milliliters of 99.9% grade N2 gas was added and the syringe was shaken for one minute and was kept at 20°C for equilibration. Carbon dioxide and CH4 concentrations in the gas-phase were then determined. For the determination of the dissolved N2O concentration, a water sample was placed into a 70-mL glass vial, and the vial was then sealed with a butyl-rubber stopper and an aluminum cap without any gas phase, followed by the injection of 1.9 mL of formaldehyde (1% final concentration) to stop biological activity. The headspace contained 30 mL of 99.9% grade N2 gas. After the glass vial was shaken for one minute and kept at 25°C for equilibration, the N2O concentration in the gas-phase was determined. The dissolved gas concentrations were calculated according to Henry's law using the solubility constants of CO2 and CH4 (CitationLide 2001) and the solubility formula of N2O (CitationWeiss and Price 1980). Sampling of the gases and water, which usually started at 10:00 hours, was conducted over a period of 10 months at intervals of approximately 2 weeks. For water sampling, wastewater was collected from the storage tank and the treated water was collected immediately after it was drained from the system for dissolved gas analyses for the oxidation–reduction potential (ORP) measurement (TOA HM-14P, Tokyo, Japan).
The concentrations of the gases were determined using gas chromatography (GC). The CO2 and CH4 concentrations were measured using a gas chromatograph (GC, Shimadzu GC-14A, Kyoto, Japan) equipped with a flame ionization detector (FID), a thermal conductivity detector (TCD), and a 2-m stainless column packed with divinylbenzene–ethylvinvylbenzen–ethylene glycol dimethacrylate (50/80 mesh). The temperatures of injection, column, FID and TCD were 80, 60, 100, 80°C, respectively. A carrier gas, ultrapure N2, flowed at a rate of 60 mL min−1. The nitrous oxide concentration was measured using a GC (Shimadzu GC-8A) equipped with an electron capture detector (ECD) and a 2-m stainless column packed with Unideads C (80/100mesh). Temperatures of injection and ECD were 200 and 300°C, respectively. A carrier gas, ultrapure grade N2, flowed at a rate of 50 mL min−1.
It was assumed that approximately 90% of the CO2 dissolved in the water samples occurred as HCO1 3 because the pH values of the water samples were almost neutral (CitationMasunaga et al. 2007). However, we expressed the amount of all the aqueous carbonate species as CO2 (dissolved CO2) in the present paper because we considered that this form would be suitable for evaluating the characteristics of the emission of total CO2 produced in the wastewater treatment processes in the MSL system.
Net gas (CO2, CH4 and N2O) flux was calculated as: net gas flux = EA+ ET − [amount of dissolved gas in the wastewater], and the N2O conversion rate was defined and calculated as: N2O conversion rate = [net N2O flux] / [total amount of nitrogen loaded].
RESULTS AND DISCUSSION
Variations in CO2, CH4 and N2O concentrations in water and gas emissions
shows the changes in the air temperature and ORP of treated water, and the concentrations of the CO2, CH4 and N2O gases dissolved in LWW and HWW and in the L-500 and L-2000 and H-500 and H-2000 waters. shows the variation in the gas fluxes from the top surface of the system into the air (EA). Although the data of the other treatments are not shown in ,, the pattern of the fluctuations in the graphs was generally identical. The L-2000 and H-2000 wastewater treatments were interrupted around February to May because of clogging (). Although the influent stopped in the system during that period, the EA values of the gases were measured and are shown in .
Figure 2 (a) Variations in air temperature and oxidation–reduction potential (ORP) of treated water and concentrations of (b) CO2, (c) CH4 and (d) N2O dissolved in the L-500, L-2000, H-500 and H-2000 treatment waters, and in wastewater containing low (LWW) and high (HWW) levels of contaminants. The period during which the influent stopped because of clogging in the L-2000 and H-2000 treatments is shown in (c).
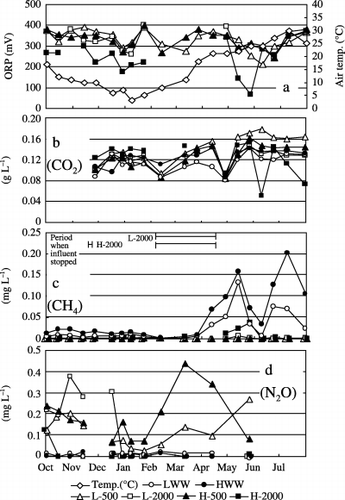
The air temperature fluctuated from 3°C in February to 30°C in July over the study period. The ORP values ranged from −41 to 377 mV for the wastewater and from 66 to 398 mV for the treated water. The ORP values in the treated water were generally lower in the HWW treatments and in the treatments at higher HLRs (). The relationship between the air temperature and ORP was not clear. Within the range of observed air temperatures, the atmospheric-equilibrium concentrations (AEC) of CO2, CH4 and N2O in pure water were estimated to be approximately 1.26–0.49 mg L−1 for CO2, 0.07–0.04 g L−1 for CH4 and 0.81–0.30 g L−1 for N2O (at 0–30°C), based on the global mean concentrations of these gases in 2002 (CitationJapan Meteorological Agency 2004).
Dissolved CO2 concentrations in the water samples ranged from 0.08 to 0.14 mg L−1 for wastewater and from 0.05 to 0.18 mg L−1 for treated water. These concentrations were approximately 100-fold as high as
Figure 3 (a) Variations in the emission rates of CO2, (b) CH4 and (c) N2O into the atmosphere during the L-500, L-2000, H-500 and H-2000 treatments. The period during which the influent stopped because of clogging in the L-2000 and H-2000 treatments is shown in (c).
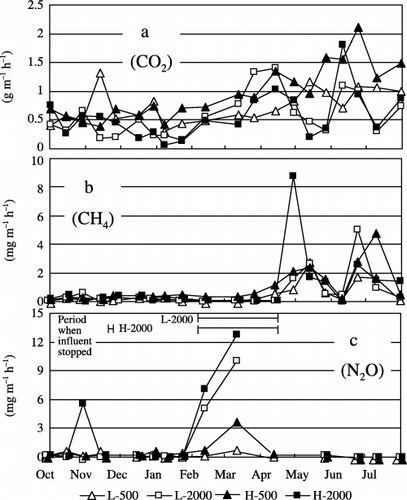
Dissolved CH4 concentrations in the treated water were in the range of not detected (ND)−0.04 g L−1 and remained very low compared with those in the wastewater, which ranged from 0.002 to 0.20 g L−1 throughout the experimental period (). The CH4 concentration in the wastewater was 5000-fold higher than the AEC (maximum) in July. Because of this high degree of supersaturation, it is possible that dissolved CH4 was released into the atmosphere as soon as the wastewater was discharged into the MSL system. The EA values of CH4 (ND−8.81 mg m−2 h−1) apparently increased from April to July, showing a very similar pattern to that of the CH4 concentration in LWW and HWW (,). When the wastewater was not discharged because of clogging during the L-2000 and H-2000 treatments, CH4 was not released from the system (). CitationSparrow and Cochran (1995) reported the effect of soil temperature on CH4 consumption. The temperature of the MSL system probably changed with changes in the air temperature. However, the CH4 emission from the MSL system did not appear to be influenced by changes in the temperature in the system.
Dissolved N2O concentrations in the treated water were in the range of ND−0.44 µg L−1and tended to decrease as the air temperature decreased from October to February () and then increased when the air temperature increased from February. The dissolved N2O concentration in the treated water was approximately 1000-fold higher than the AEC (maximum) in March. In contrast, the dissolved N2O concentrations in the wastewater were in the range of ND−0.02 g L−1 and remained very low throughout the experimental period. The EA values of N2O ranged from ND to 12.8 mg m−2 h−1 and were relatively high from February to March, especially when clogging occurred during the L-2000 and H-2000 treatments. Accumulation of organic matter and nitrogen in the system in the cold season and the increase in the air temperature from February to March may have stimulated the denitrification process and N2O production in the MSL system. Although the ORP value of treated water is often related to the conditions of nitrogen treatment in a MSL system (CitationMasunaga et al. 2007), the relationship between the ORP values and N2O concentrations in the treated water was not clear in the present study.
Characteristics of CO2, CH4 and N2O emissions in relation to HLR
shows the ET and EA values of CO2, CH4 and N2O per 1,000 L of wastewater, while shows the net fluxes of the gases in the steady state of the wastewater treatments. Most of the CO2 released from the system originated from the wastewater (). Carbon dioxide was released from the system mainly in a dissolved form in the treated water (ET). Although the
Figure 4 Mean amounts of (a) CO2, (b) CH4 and (c) N2O emitted into the atmosphere (EA) and treated water (ET) per 1,000 L of wastewater. The values for wastewater containing low (LWW) and high (HWW) levels of contaminants correspond to the amounts of gases dissolved in 1,000 L of wastewater. Vertical bars indicate the standard errors of the total values (EA + ET) during the experiment.
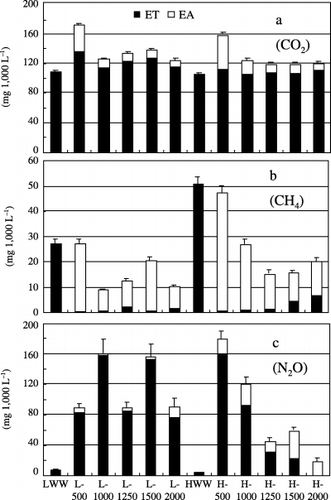
Table 1 Net fluxes (mean ±#standard error) of CO2, CH4 and N2O from the systems in the steady state of the wastewater treatments†
Methane emission from the system was lower than the amount originally contained in LWW and HWW, except for the L-500 and H-500 treatments, and was mainly released as EA (), indicating that CH4 was consumed in the MSL system, based on the net emission (). Although it has been reported that N fertilization frequently reduced CH4 consumption in soil (CitationKing and Schnell 1994; CitationMosier et al. 1991), N supply into the MSL system through LWW and HWW discharge did not appear to affect CH4 consumption in the system. CitationKong et al. (2002) reported that the CH4 emission from a soil trench system increased with a decrease in ORP in the system when the duration of the treatment period increased. In the MSL system, the ORP value of treated water generally remained above 250 mV (). Although ORP inside the MSL system was not directly measured, it was assumed that the system remained under aerobic and oxidative conditions. The high ORP value appeared to contribute to efficient CH4 oxidation. However, in the L-500 and H-500 treatments, the emission was relatively high (), although the net fluxes were almost zero (), presumably because the gas exchanges between the system and the atmosphere were more frequent in the lower HLR treatment. In the MSL system, the water content increased when the HLR increased (; CitationMasunaga et al. 2007), and a greater amount of organic matter remained undecomposed and accumulated at higher HLRs. The accumulation of water or organic matter in the system possibly closed the pore spaces in the water permeable layer, especially in the top layer where most of the suspended solid (SS) was removed. This may have prevented gas exchanges between the system and the atmosphere at HLRs above 1,000 L m−2 day−1. Methane gas released from the wastewater into the gas phase inside the MSL system might have remained for a longer period of time because of the less frequent gas exchanges, and it was efficiently oxidized in the gas phase inside the MSL system. Although the ET values tended to increase at higher HLRs, especially in the HWW treatments, probably because of the shorter water retention time, the CH4 flux was clearly lower at HLRs above 1,000 L m−2 day−1 compared with the values in the L-500 and H-500 treatments ().
Nitrous oxide was mainly released from the system as ET in all the treatments, except for the H-1500 and H-2000 treatments. The EA values in the HWW treatments were higher than those in the LWW treatments. The emission per 1,000 L of wastewater tended to decrease as HLR increased in the HWW treatments, while it was not affected by the HLR changes in the LWW treatments. Net N2O flux was relatively high at high HLRs in the LWW treatments compared with the HWW treatments at high HLRs. Nitrous oxide is produced by both nitrification and denitrification processes. In the wastewater treatments of the MSL system, the HWW treatments, especially at higher HLRs, led to a lower N2O emission (), which might result from the lower ORP values in the system (; CitationMasunaga et al. 2007). The conditions in the HWW treatments at higher HLRs probably led to a decrease of N2O production by the enhancement of the reduction of N2O to N2 in the denitrification process. The ET values of N2O were significantly reduced in the H-1250 H-1500 and H-2000 treatments ().
Nitrogen removal characteristics and N2O emission
shows the nitrogen removal efficiency and the N2O conversion rate in each treatment. Nitrogen removal rates tended to be high at high HLRs and in the HWW treatments. The N2O emission was also higher at higher
Table 2 Characteristics of nitrogen treatment and N2O conversion rates in the multi-soil-layering system
REFERENCES
- Luanmanee , S , Boonsook , P , Attanandana , T and Wakatsuki , T . 2002 . Effect of organic components and aeration regime on efficiency of multi-soil-layering system for domestic wastewater treatment . Soil SciPlant Nutr , 48 : 125 – 134 .
- Masunaga , T , Sato , K and Wakatsuki , T . 2002a . Removal of simazin, fenitrothion, napropamid and tetrachloroethylene by multi-soil-layering method . JJpn SocWater Env , 25 : 361 – 366 . (in Japanese with English abstract)
- Masunaga , T , Sato , K , Zennami , T , Fujii , S and Wakatsuki , T . 2003 . Direct treatment of polluted river water by the multi-soil-layering method . JWater EnvTech , 1 : 97 – 104 .
- Wakatsuki , T , Esumi , H and Omura , S . 1993 . High performance and N and P-removable on-site domestic wastewater treatment system by multi-soil-layering method . WatSciTech , 27 : 31 – 40 .
- MasunagaT SatoK WakatsukiT . Environmental remediation using purification function of soil by multi-soil-layering system . Proceedings of the 17th World Congress of Soil Science, Symposium 55 . August 14–21 2002 , Bangkok, Thailand.
- Sato , K , Masunaga , T and Wakatsuki , T . 2002a . Treatment of bio-genic wastewater by multi-soil layering method – effect of various positions of aeration using small model system . JJpn SocWater Env , 25 : 234 – 241 . (in Japanese with English abstract)
- Sato , K , Masunaga , T and Wakatsuki , T . 2005a . Water movement characteristics in a multi-soil-layering system . Soil SciPlant Nutr , 51 : 75 – 82 .
- Sato , K , Masunaga , T and Wakatsuki , T . 2005b . Characterization of treatment processes and mechanisms of COD, phosphorous, and nitrogen removal in a multi-soil-layering system . Soil SciPlant Nutr , 51 : 213 – 221 .
- Luanmanee , S , Attanandana , T , Masunaga , T and Wakatsuki , T . 2001 . The efficiency of a multi-soil-layering system on domestic wastewater treatment during the ninth and tenth years of operation . EcoEng , 18 : 185 – 199 .
- SatoK IhaY LuanmaneeS MasunagaT WakatsukiT . Long term on-site experiments and mass balances in waste water treatment by multi-soil-layering system . Proceedings of the 17th World Congress of Soil Science, Symposium 55 . August 14–21 2002 , Bangkok, Thailand.
- Czepiel , P , Crill , P and Harriss , R . 1995 . Nitrous oxide emission from municipal wastewater treatment . EnvironSciTechnol , 29 : 2352 – 2356 .
- Intergovernmental Panel on Climate Change . 1995 . Climate Change 1995, The Science of Climate Change , London : Cambridge University Press .
- Itokawa , H , Hanaki , K and Matsuo , T . 1996 . Nitrous oxide emission during nitrification and denitrification in a full scale night soil treatment plant . Water SciTechnol , 34 : 277 – 284 .
- Kimochi , Y , Inamori , Y and Matsumura , M . 1998 . Influence of nitrogen loading on N2O emission and nitrogen removal from the DO controlled intermittent aeration activated sludge process . JJpnSocWatEnv , 21 : 163 – 169 . (in Japanese with English abstract)
- Kong , HN , Kimochi , Y , Mizuochi , M , Inamori , R and Inamori , Y . 2002 . Study of the characteristics of CH4and N2O emission and methods of controlling their emission in the soil-trench wastewater treatment process . SciTotal Env , 290 : 59 – 67 .
- Master , Y , Lauglin , RJ , Shaviv , U , Stevens , RJ and Shaviv , A . 2003 . Gaseous nitrogen emissions and mineral nitrogen transformations as affected by reclaimed effluent application . JEnvironQual , 32 : 1204 – 1211 .
- United States Department of Agriculture (USDA) . 1999 . Soil Taxonomy , Washington : USDA .
- Masunaga , T , Sato , K , Mori , J , Shirahama , M , Kudo , H and Wakatsuki , T . Characteristics of wastewater treatment by a multi-soil-layering system in relation to wastewater quality and hydraulic loading rates . Soil Sci. Plant Nutr. , 53 206 – 214 .
- Liikanen , A , Flojt , L and Martikainen , P . 2002 . Gas dynamics in eutrophic lake sediments affected by oxygen, nitrate, and sulfate . JEnvironQual , 31 : 338 – 349 .
- Senga , Y , Seike , Y , Mochida , K , Fujinaga , K and Okumura , M . 2001 . Nitrous oxide in brackish lakes Shinji and Nakaumi, Japan . Limnology , 2 : 129 – 136 .
- Lide , DR . 2001 . CRC Handbook of Chemistry and Physics , 81st edn , Boca Raton : CRC press .
- Weiss , RF and Price , BA . 1980 . Nitrous oxide solubility in water and seawater . MarChem , 8 : 347 – 359 .
- Japan Meteorological Agency . 2004 . Climate Change Monitoring Report 2003 , Tokyo : Japan Meteorological Agency .
- Sparrow , EB and Cochran , VL . 1995 . “ Effect of soil depth and temperature on CH4consumption in subarctic agricultural soils ” . In Soil Management and Greenhouse Effect , Edited by: Lai , R , Kimble , J , Levine , E and Stewart , BA . 197 – 204 . Boca Raton : CRC Press .
- King , GM and Schnell , S . 1994 . Effect of increasing atmospheric methane concentration on ammonium inhibition of soil methane consumption . Nature , 370 : 282 – 284 .
- Mosier , A , Schimel , D , Valentine , D , Bronson , K and Parton , W . 1991 . Methane and nitrous oxide emissions in native, fertilized and cultivated grasslands . Nature , 350 : 330 – 332 .