Abstract
A future increase in heavy precipitation events is predicted in boreal regions. An irrigation experiment was conducted in Taiga forest in eastern Siberia to evaluate the effect of heavy precipitation on greenhouse gas ([GHG] CO2, CH4, and N2O) fluxes in the soil. The GHG fluxes on the soil surface were measured using a closed-chamber method and GHG production rates in the mineral soil were estimated using the concentration–gradient method based on Fick’s law. Irrigation water (20 mm day−1) was applied continuously for 6 days (120 mm in total; the same amount as summer precipitation in this region). Greenhouse gas production rates in the organic layer (O-layer) were defined as the difference between the GHG fluxes and the GHG production rates in the mineral soil. Carbon dioxide flux was measured both in root-intact (R s ) and trenched plots (R mw ). The root respiration rate (R r ) was calculated as the difference between R s and R mw . Considering the root distribution in the soil, we regarded the CO2 production rate in the mineral soil to be the microbial respiration rate in the mineral soil (R mm ) and microbial respiration rate in the O-layer (R mo ) as the difference between R mw and R mm . Irrigation increased both soil temperature and moisture in the irrigated plot. The R s , CH4 flux and N2O flux during the irrigation period were higher in the irrigated plot than that in the non-irrigated plot (P < 0.05; mean R s ± standard deviation [SD] (mg C m−2 h−1) were 171 ± 20 and 109 ± 11, mean CH4 flux ± SD (μg C m−2 h−1) were −5.4 ± 4.1 and −14.0 ± 6.5, and mean N2O flux ± SD (μg N m−2 h−1) were 1.6 ± 1.6 and 0.2 ± 1.1, respectively). Soil moisture had a positive effect on R mm and CH4 production rate in the O-layer, a negative effect on R r , and did not affect R mo , the CH4 production rate in the mineral soil or the N2O production rates in both the O-layer and the mineral soil. Soil temperature had a positive effect on R r and R mo . The increment of global warming potential of the soil mainly resulted from an increase in microbial respiration rates. Future changes in precipitation patterns in this region would accelerate decomposition of the soil organic matter.
Introduction
Forests in Russia contain 381 Pg of carbon (C), which accounts for 54% of the total C stock in forests in the northern hemisphere (CitationGoodale et al. 2002), and play an important role as a C sink. Taiga forest near Yakutsk in eastern Siberia lying on permafrost keeps the soil temperature low (CitationEugster et al. 2000), and the decomposition rate *of soil organic matter is very slow (CitationRodionow et al. 2006). In addition, the amount of precipitation in this region is very low (CitationJapan Meteorological Agency 2004).
The Intergovernmental Panel on Climate Change (CitationIPCC 2007) predicts that an increase in air temperature, in the amount of precipitation and in heavy precipitation events will occur in the permafrost region as a result of global warming caused by an increase in the concentration of greenhouse gases (GHGs). In Siberia, heavy precipitation events exceeding 20 mm day−1 significantly increased during 1936–1994 (CitationEasterling et al. 2000). An increase in air temperature will accelerate the growth of vegetation and increase the C storage of biomass and litter (CitationSirotenko and Abashina 2008). In contrast, increased soil temperature accompanied by thawing of permafrost will accelerate the decomposition rate of soil organic matter, enhancing global warming (CitationRodionow et al. 2006). It is still unknown what effects a change in precipitation pattern will have on the plant growth rate and the decomposition rate of soil organic matter in this region.
Many field studies have shown that precipitation or irrigation on dry soil increased the rate of soil respiration owing to an increase in soil moisture (e.g. CitationMillard et al. 2008). CitationOberbauer et al. (1992) reported that precipitation events that raised the water table were found to strongly reduce soil respiration in the permafrost region in Alaska. However, very few studies have attempted to divide the effect of precipitation on soil respiration into microbial respiration and root respiration, which are the components of soil respiration (CitationBond-Lamberty et al. 2004). Dividing soil respiration is important to evaluate the effect of heavy precipitation on plant physiology and the decomposition rate of soil organic matter. Previous studies observed large increases in root and microbial respiration as a result of adding water to dry soil (e.g. CitationBorken et al. 2003; CitationMillard et al. 2008). However, the response of root and microbial respiration to heavy precipitation in the permafrost region is not clear.
In the permafrost region, heavy precipitation would affect not only the CO2 flux from the soil, but also the exchange of the GHGs CH4 and N2O. In general, natural oxic soil absorbs CH4 (CitationPotter et al. 1996). CitationStriegl et al. (1992) reported that precipitation on dry soil increased CH4 consumption by the soil. CitationLiu et al. (2008) reported that CH4 fluxes showed little difference between irrigated and non-irrigated soils in an Inner Mongolian steppe soil. Citationvan Huissteden et al. (2008) reported that CH4 consumption in a forest near Yakutsk remained stable even when the water table fluctuated from 9 to 27 cm. This indicates that methanotrophs occur in the uppermost soil and fluctuations in soil moisture do not affect CH4 consumption in the forests of this region.
Very few studies have reported the effect of precipitation on N2O emission from boreal forests. CitationRodionow et al. (2006) reported that emission of N2O is negligible from natural Siberian soils. Forest soils near Yakutsk emit or absorb little N2O (CitationMorishita et al. 2007; CitationTakakai et al. 2008). CitationDu et al. (2006) reported that N2O emission was stimulated by precipitation events in natural grassland in Inner Mongolia.
The purpose of the present study was to evaluate the effect of heavy precipitation on GHG fluxes from soil in Taiga forest near Yakutsk by conducting an irrigation experiment. In the present study, we measured GHG production rates both in the O-layer and in the mineral soil, and microbial respiration and root respiration separately to evaluate the effect of precipitation on the global warming potential of the soil in this ecosystem in detail.
Materials and methods
Site description
The present study was conducted during July 2004 in a 180-year-old larch forest on sandy loam soil underlain by permafrost (Typic Haploturbels; CitationSoil Survey Staff 2010) in the Spasskaya-Pad experimental forest (62°15′N, 129°37′E) of the Institute for Biological Problems of Cryolithozone, near Yakutsk, Russia. The site is located on a gently sloped (1–2° decline toward north) terrace approximately 200 m in elevation situated on the left bank of the Lena River. The mean annual temperature is −10.0 °C and the mean annual precipitation is approximately 237 mm (CitationJapan Meteorological Agency 2004). The amount of precipitation in 2004 preceding the experiment was lower than the mean precipitation for 30 years (), indicating that the forest floor was relatively dry during the experiment. The forest is mainly occupied by larch trees (Larix cajanderi). The forest floor is covered by shrubby vegetation, including Vaccinium vitis-idaea and Arctous erythrocarpa, and by a thick 10 cm organic layer (O-layer).
The amount of precipitation from 17 June to 26 July 2003 was recorded using a rain gauge (Young, Traverse, Michigan, USA) placed at the top of a 32-m high scaffolding tower built by CitationOhta et al. (2001).
Layout of the experimental plots
An irrigated plot and a non-irrigated plot of the same size (14 m × 14 m) were established. The non-irrigated plot was established in an area more than 50 m apart from the irrigated plot and was not affected by the irrigation (). Four subplots, measuring 0.16 m2, were set up in spring 2003 in each irrigated and non-irrigated plot. Around each subplot, the outside edges of the 0.16 m2 were trenched. The trenches were approximately 30 cm deep into the soil, which is deep enough to prevent the invasion of roots of Siberian larch; we estimated from our soil profile examination that approximately 97% of fine roots (<2 mm in diameter) are distributed within the −10 to 26 cm depth of the soil (; CitationOno 2003). The trenches were lined with plastic sheets before backfilling to prevent root growth. The above-ground parts of all vegetation were removed from the trenched plots.
Irrigation was carried out between 17.00 and 21.00 hours from 17 to 22 July 2004. Irrigation was accomplished using a rubber hose from a height of approximately 1.5 m. Irrigation of 20 mm per day was conducted every day and a total of 120 mm of irrigation water was applied. The amount of irrigation was approximately equal to the average amount of natural precipitation, including heavy precipitation events, during the summer season (June–September). Lena River water was used for the irrigation and the temperature of the irrigated water was approximately 15°C.
Figure 1 Seasonal variation in air temperature and precipitation in Yakutsk. Open and closed circles indicate the average air temperature for each month over 30 years and in 2004, respectively. Open and closed bars indicate the average precipitation for each month over 30 years and the amount of precipitation for each month in 2004, respectively.
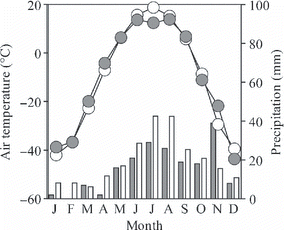
Figure 2 Location map of the study site and the layout of the measurement plots. Microbial respiration in the whole soil was measured in the trenched plots. Fluxes and concentrations of greenhouse gases in soil air were measured near the trenched plots.
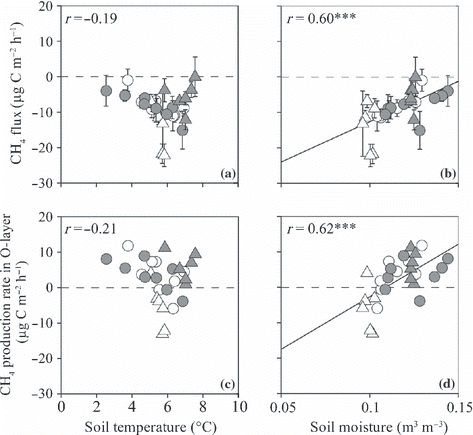
Table 1 Soil physical and chemical properties at the study site
Field measurements
The CO2 fluxes from the surface of the O-layer (R s ) were measured using a closed-chamber method (CitationKusa et al. 2008; CitationMorishita et al. 2005, 2006, 2007; CitationTakakai et al. 2008) from 1 to 23 July 2004. To measure R s , including root and microbial respiration, four stainless steel cylinders, approximately 20 cm in diameter and 25 cm height, were installed at a depth of 4 cm into the soil both in the irrigated and non-irrigated plots at least 24 h before measurement. The above-ground parts of all plants inside the cylinders were removed carefully to exclude plant respiration. Before beginning the measurements, an air sample inside the cylinder was transferred to a Tedlar bag (OMI Odorair Service, Shiga, Japan) as a 0-min sample, and an acrylic lid with silicone gasket and an inflatable plastic bag to adjust the air pressure inside the chamber was placed on the cylinder immediately. Six minutes later, a 500 mL air sample from inside the chamber was taken out. Each chamber remained open for more than 10 min after completion of the CO2 flux measurement to allow for a complete exchange of air inside the chamber. Gas samples were then taken to measure CH4 and N2O fluxes on the surface of the O-layer. At 0, 30 and 60 min after the top of the chamber was again closed with a lid, a 20 mL gas sample from inside the chamber was taken into a 10 mL vacuum vial and the vial was sealed with a butyl rubber stopper (SVF-10; Nichiden-Rika, Kobe, Japan).
One stainless steel cylinder was installed into the middle of each trenched plot in both the irrigated and non-irrigated plots in four replicates to measure the rate of microbial respiration in the whole soil (R mw ). The measurements were carried out on the same day as the gas sampling. The procedure for measuring R mw was the same as that used to measure CO2.
Stainless steel pipes, 9 mm in diameter, were installed at depths of 10, 20, 30 and 50 cm (four pipes at each depth) out of the trenched plot in both the irrigated and non-irrigated plots in the spring of 2003 to collect air samples from the soil. The pipes were sealed by three-way cocks to allow the gas concentration in the pipes to equilibrate with the soil air. From each of the pipes installed at each depth and each treatment, air samples (50 mL) were taken into a Tedlar bag during the chamber measurements. All samples taken from the same depth and treatment were mixed into a Tedlar bag. Air samples of 50 mL from each of the Tedlar bags were diluted with CO2-free air for CO2 analysis, and 20 mL was transferred to 10 mL glass bottles for CH4 and N2O analysis.
These measurements were conducted between 10.00 and 18.00 hours to avoid diurnal effects on fluxes. We did not carry out measurements within 12 h of irrigation to avoid effects of the physical movement of water in the soil.
During the chamber measurements, the air and soil temperature and the soil moisture around each chamber were recorded manually. The air temperature around each chamber was measured with a digital thermometer to calculate GHG fluxes. Two replicate soil temperature measurements at a depth of 10 cm near each chamber, which is the boundary between the O-layer and the mineral soil, were also taken using digital thermometers. Two replicate soil moisture measurements as volumetric water content at a soil depth of 0–10 cm (O-layer) from each chamber base were taken with a calibrated time domain reflectometer (TDR) sensor (TRIME-como; Tohoku Electronic Industrial Company, Sendai, Japan).
Soil temperature and moisture were recorded automatically approximately 5 m away from each chamber in both the irrigated and non-irrigated plots (CitationLopez et al. 2007). Soil temperature was measured using calibrated thermistors at depths of 10, 20, 30, 40 and 60 cm (T 10, T 20, T 30, T 40, and T 60, respectively; 104ET, Ishizuka Denshi, Tokyo, Japan). Because of some sensor troubles with a thermistor at the 20 cm depth, we excluded the T 20 data from the analysis. The thermistors were calibrated using an ice-water bath with a precision of 0.02°C at 0°C; the overall probe accuracy for the temperature range of −20 to 30°C was calculated to be better than ±0.09°C. T 10 was recalibrated using soil temperature measured manually around the chambers. Soil moisture was measured using the frequency domain reflectometry (FDR) method at depths of 0–10, 10–20, 20–30, 30–40 and 50–60 cm (W10, W20, W30, W40 and W60, respectively; EnviroSMART, Sentek, South Australia, Australia). The FDR sensors were calibrated separately for depths of 0–10 cm and for depths below 10 cm (mineral soil layer). For this purpose, we collected 11 in situ soil samples of each soil depth with various moisture conditions and determined the volumetric water content gravimetrically to construct a calibration curve. W10 was recalibrated using soil moisture measured manually around the chambers. Soil temperature was recorded every 30 s and stored every 1 h on average, whereas soil moisture was recorded every 1 h. The soil temperature and moisture recorded at the time of gas sampling were used for the statistical analyses.
Physical and chemical conditions of the soil and water samples
To analyze the physical properties of the soil and water samples, three soil cores 100 cm3 in size were sampled from each layer in 2003. With these undisturbed cores, we measured the volume of the air-filled pore space (AFPS) using a three phase meter (Model DIK-1110; Daiki Rika Kogyo Co., Saitama, Japan). The gas tortuosity factor (D/D 0) of the undisturbed soil cores was measured and calculated using the method developed by CitationOsozawa and Kubota (1987) under steady-state conditions using O2 as the diffusing gas. The cores were oven-dried (105°C) for 48 h to obtain bulk density and water-filled pore space (WFPS). The porosity was calculated as the sum of WFPS and AFPS. The length of the fine roots (<2 mm in diameter) in each layer was calculated using a theoretical equation after CitationOno (2003) as follows:
where R is the length of the fine roots in a unit volume of each layer (cm cm−3; ), n is the number of the cut ends of fine roots appearing on each layer in the soil profile and S is the area of each layer in the soil profile (cm−2).
Fresh soil samples were sieved (2 mm) and used for the chemical analysis. The concentrations of NO3 −-N in a 1:20 for the O-layer and a 1:10 for the mineral soil : deionized water mixture were measured using ion chromatography (TOA DIC Analyzer ICA-2000; TOA DKK, Tokyo, Japan). The concentrations of NH4 +-N in a 1:20 for the O-layer and a 1:10 for the mineral soil : 1 mol L−1 KCl solution mixture were measured using colorimetry (the indophenol-blue method) with a UV-VIS spectrophotometer (UV mini 1240; Shimadzu, Kyoto, Japan).
Irrigated water was sampled directly from the rubber hose that was used to apply the irrigation water. Rain water was sampled using eight rain gutters (0.12 m2 each) that had been placed around the irrigated and non-irrigated plots.
The concentrations of NH4 +-N and NO3 −-N in the irrigation and rain water were also measured. The contents of NH4 +-N and NO3 −-N in the soil were calculated by multiplying the bulk density by the NH4 +-N or NO3 −-N concentration of each layer of the soil. The contents of NH4 +-N and NO3 −-N in the irrigation and rain water were calculated by multiplying the amount of irrigation or rain water by the NH4 +-N or NO3 −-N concentrations of the water.
Analysis of the CO2, CH4 and N2O concentrations
The CO2 concentration was determined with a portable infrared CO2 gas analyzer (ZFP9GC11; Fuji Electric Systems, Tokyo, Japan). The CH4 and N2O concentrations were analyzed using gas chromatography equipped with a flame ionization detector and an electron capture detector, respectively (GC-8A and GC-14B; Shimadzu).
Estimation of soil-gas diffusivity and calculation of flux, respiration and production rates of greenhouse gases
Greenhouse gas fluxes, measured using a closed-chamber method, were calculated as follows:
where F c is the flux of GHGs obtained using the closed-chamber method (μg C or N m−2 h−1), ρ is the gas density of GHGs (CO2-C, CH4-C, 0.536 × 103 g m−3; N2O-N, 1.25 × 103 g m−3), V/A is the height of the chamber (m), dc/dt is the ratio of change in the gas concentration (c) inside the chamber per unit time (t) during the sampling period (m3 m−3 h−1) and T is the air temperature (ºC).
We estimated the flux of each GHG from the mineral soil to the O-layer using a soil gradient method (CitationKusa et al. 2008). The soil-gas diffusivities were estimated using the soil AFPS (). Estimated diffusivities were corrected for temperature effects using DT/D25 = [(273 + T)/298]1.75 (CitationReid et al. 1977). Greenhouse gas flux from the mineral soil to the O-layer was determined from Fick’s law as:
where F d is the flux obtained from Fick’s law (μg C or N cm−2 s−1), D is the soil gas coefficient (cm2 s−1) at the surface of the mineral soil calculated using the gas tortuosity factor (D/D 0) and the molecular diffusivity of each GHG in air (D0CO2 = 0.138, D0CH4 = 0.191, D0N2 O = 0.143) and dc/dz is the ratio of change in the gas concentration (c) along the soil depth (z) (μg C or N cm−3 cm−1). We made quadratic functions of depth (10, 20, 30 and 50 cm) to each GHG concentration to calculate dc/dz at the 10-cm depth, the boundary between the O-layer and the mineral soil, as the slope of the tangent to the quadratic functions at the 10-cm depth (CitationTakle et al. 2004). We did not use the GHG concentration data at the 0-cm depth, ambient air, to make the quadratic functions because there is always some variation in the gas concentrations owing to the turbulent mixing of ambient air with to some extent the topmost O-layer (CitationPihlatie et al. 2007).
Figure 3 Effect of soil air-filled pore space on the measured gas tortuosity factor (diffusivity relative to that for still air). The different symbols distinguish measurements on soil cores from different layers.
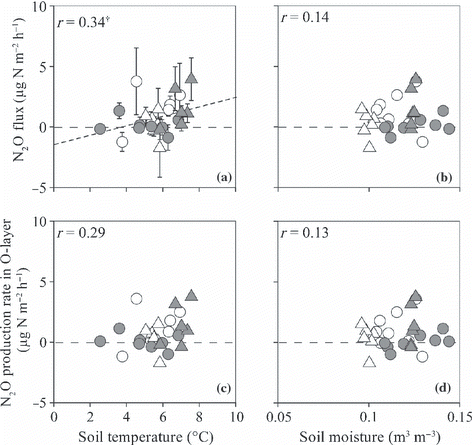
The root respiration rate (R r ) of each measurement was calculated as the difference between the CO2 flux from the surface of the O-layer in the root-intact (R s ) and trenched plots (the microbial respiration rate in the whole soil [R mw ]) of each measurement. One negative value of R r was eliminated. We regarded the estimated GHG fluxes from the mineral soil into the O-layer as the GHG production rates in the mineral soil, assuming no movement of GHGs in the permafrost. The GHG production rates in the O-layer were calculated as the difference between the GHG fluxes from the surface of the O-layer and the production rates of GHG in the mineral soil to avoid problems caused by the turbulent mixing of ambient air and the unevenly distributed O-layer constituents (CitationDavidson and Trumbore 1995; CitationPihlatie et al. 2007; CitationSchwendenmann and Veldkamp 2006). For CO2, we regarded the CO2 production rates in the mineral soil as the microbial respiration rates in the mineral soil (R mm ) assuming that almost all R r was occurring in the O-layer because in the most part the fine roots were distributed in the O-layer (). The microbial respiration rate in the O-layer (R mo ) was calculated as the difference between R mw and R mm .
The following equations were applied to the measured temperature and CO2 flux or production rate data (F CO2 ) to calculate the normalized CO2 flux or the production rate (F CO2,b) corresponding to the base temperature (T b, 5°C in the present study):
where a and b are fitted constants and T is the soil temperature at the 10-cm depth (CitationTakakai et al. 2008).
Negative fluxes and production rates of CH4 and N2O represent downward movement or net consumption of the gases.
To evaluate the relative significance of CO2, CH4 and N2O, their global warming potentials ([GWP] mg CO2-eq m−2 h−1) expressed in CO2-equivalent values were calculated by multiplying the CO2, CH4 and N2O fluxes by 44/12, 16/12 × 25 and 44/28 × 298, respectively (100-year time horizon; CitationIPCC 2007).
Statistical analyses
Two-way anovas or paired t-tests were carried out to compare the average GHG fluxes, GHG production, soil temperature or soil moisture obtained from the irrigated and non-irrigated plots before and during the irrigation period.
Linear or non-linear regression analyses were carried out to examine the relationship between the measured GHG flux and production, soil temperature and moisture. Spearman’s correlation analyses were carried out to examine the relationship between W20 and other variables because of the non-normal distribution of W20.
A multiple regression analysis was used to evaluate the contributions of R r , R mo , R mm , CH4 production in the O-layer and the mineral soil, and N2O production in the O-layer and the mineral soil to GWP.
Significance for all statistical analyses was accepted at P = 0.05. All statistical analyses were carried out using the statistical package R, version 2.9.1 (CitationR Development Core Team 2009).
Results
Physical and chemical properties of the soil
The soil physical and chemical properties are shown in . The dry bulk density in the O-layer was 0.41 and the density in the mineral soil ranged from 1.1 to 1.9 Mg m−3, and increased as the depth increased. The porosity in the mineral soil ranged from 0.34 to 0.55 m3 m−3 and D/D 0 in the mineral soil before the irrigation experiment ranged from 0.01 to 0.11 m2 s−1, and both decreased with an increase in depth. The NO3 −-N and NH4 +-N concentrations in each layer ranged from 0.02 to 0.23 mg kg soil−1 and from 0.00 to 0.02 g kg soil−1, respectively, and both were highest in the O-layer, whereas there was no trend in the mineral soil. The NH4 +-N contents in the O-layer and in the mineral soil (1–62 cm) were 816 and 9,160 mg m−2, respectively, and the NO3 −-N contents were 9 and 82 mg m−2, respectively. The estimated length of fine roots in each layer ranged from 0 to 80 m L−1 and 86% of the fine roots were distributed in the O-layer.
Precipitation, soil temperature and soil moisture
A total of 11.4 mm of precipitation occurred during the 30 days before the irrigation period. The amount of precipitation from 17 June to 27 July was 19.8 mm.
The soil temperature at depths of 10, 30, 40 and 60 cm (T 10, T 30, T 40 and T 60, respectively) during the flux measurements ranged from −0.5 to 7.6 °C in the irrigated plot and from −0.0 to 6.9 °C in the non-irrigated plot (). Before the irrigation period, the average soil temperature at each depth in the irrigated plot was significantly lower than that in the non-irrigated plot (; P < 0.01). During the irrigation period, the average T 10 in the irrigated plot was significantly higher than that in the non-irrigated plot (P < 0.01), the average T 60 in the irrigated plot was significantly lower than that in the non-irrigated plot (P < 0.001), and the average T 30 and T 40 did not differ between the irrigated and non-irrigated plots.
Soil moisture in the O-layer (W10) during the flux measurements ranged from 0.11 to 0.14 m3 m−3 in the irrigated plot and from 0.10 to 0.13 m3 m−3 in the non-irrigated plot (). Soil moisture at depths of 10–20, 20–30, 30–40 and 50–60 cm (W20, W30, W40, and W60, respectively) ranged from 0.13 to 0.31 m3 m−3 in the irrigated plot and from 0.10 to 0.16 m3 m−3 in the non-irrigated plot. Soil moisture increased with an increase in depth in both plots. Although the average soil moisture at each depth in the irrigated plot was significantly higher than that in the non-irrigated plot, both before and during the irrigation period (; P < 0.01), soil moisture in the irrigated plot tended to increase with an increase in irrigation (). W20 in the irrigated plot was significantly higher during the irrigation period than before the irrigation period and W20 in the non-irrigated plot was significantly lower during the irrigation period than before the irrigation period (both at P < 0.001).
Figure 4 (a,b) Temporal variation in soil temperature at 10, 30, 40 and 60 cm depths (T10, T30, T40, and T60, respectively) and (c,d) soil moisture at 0–10, 10–20, 20–30, 30–40 and 50–60 cm depths (W10, W20, W30, W40, and W60, respectively) of the non-irrigated (a,c) and irrigated plots (b,d). Arrows indicate the irrigation events for the irrigated plot. Open bars represent the precipitation amount.
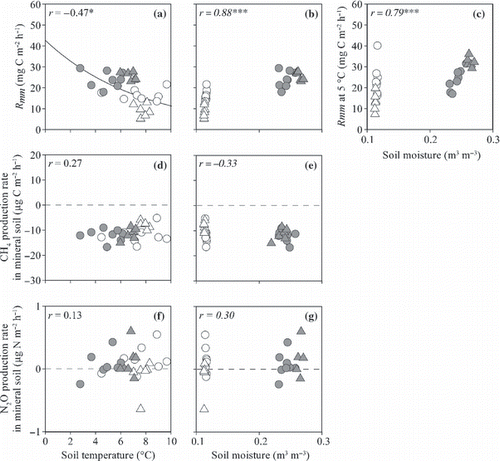
Table 2 Average greenhouse gas fluxes, production, global warming potentials and soil temperature and moisture during each period of measurement
Nitrogen supply by irrigation and precipitation
The NH4 +-N and NO3 −-N concentrations in the irrigation water were 0.09 and 0.10 mg L−1 and the concentrations in the rain water were 0.14 and 0.005, respectively. The NH4 +-N and NO3 −-N supply to the soil by irrigation were 11 and 12 mg m−2 and by rain water were 2.8 and 0.1, respectively. A total of 13.8 mg m−2 of NH4 +-N and 12.1 of NO3 −-N were added to the soil over the measurement period.
Greenhouse gas flux from the surface of the O-layer
The CO2 flux from the surface of the O-layer in the root-intact plot (R s ) ranged from 59 to 200 mg C m−2 h−1 in the irrigated plot and from 70 to 142 in the non-irrigated plot, and both fluctuated with variation in the soil temperature (,). Before the irrigation period the average R s (mean ± standard deviation [SD]) in the irrigated plot (106 ± 32 mg C m−2 h−1; ) did not differ from that in the non-irrigated plot (116 ± 21). During the irrigation period, the average R s in the irrigated plot (171 ± 20) was significantly higher than that in the non-irrigated plot (109 ± 11; P < 0.001). The CO2 flux from the surface of the O-layer in the trenched plot (microbial respiration rate in whole soil; R mw ) ranged from 50 to 132 mg C m−2 h−1 in the irrigated plot and from 54 to 109 in the non-irrigated plot, and accounted for 42–108% (mean ± SD; 67 ± 12%) of R s (). This percentage was high at the beginning of the measurement period and decreased with time. Before the irrigation period, the average R mw (mean ± SD) in the irrigated plot (67 ± 15 mg C m−2 h−1; ) was significantly lower than that in the non-irrigated plot (80 ± 15; P < 0.05). During the irrigation period, the average R mw in the irrigated plot (122 ± 10) was significantly higher than that in the non-irrigated plot (63 ± 6; P < 0.001).
The ranges of the root respiration rate (R r ), calculated from the difference between R s and R mw , were 26–90 and 12–53 mg C m−2 h−1 in the irrigated and non-irrigated plots, respectively, accounting for 17–58% (mean ± SD; 35 ± 9%) of R s (). This percentage was low at the beginning of the measurement period and increased with an increase in soil temperature in the non-irrigated plot, whereas irrigation depressed the rate in the irrigated plot. The average R r in the irrigated plot did not differ from that in the non-irrigated plot before or during the irrigation period ().
Figure 5 Temporal variation in (a) CO2 fluxes from the surface of the O-layer (Rs), (b) microbial respiration from the surface of the O-layer (Rmw) and (c) root respiration (Rr). Open symbols indicate data from the non-irrigated plot and closed symbols indicate data from the irrigated plot. Vertical bars denote the standard error of each parameter. Arrows indicate the irrigation events for the irrigated plot.
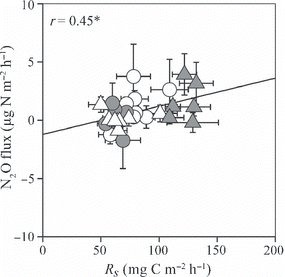
The CH4 flux from the surface of the O-layer ranged from −15 to −0 μg C m−2 h−1 in the irrigated plot and from −22 to −1 in the non-irrigated plot (). Before the irrigation period, the average CH4 flux (mean ± SD) in the irrigated plot (−8.3 ± 3.4 μg C m−2 h−1; ) did not differ from that in the non-irrigated plot (−8.3 ± 3.5). During the irrigation period, the CH4 flux in the irrigated plot (−5.4 ± 4.1) was significantly higher than that in the non-irrigated plot (−14.0 ± 6.5; P < 0.001).
The N2O flux from the surface of the O-layer ranged from −0.9 to 3.9 μg N m−2 h−1 in the irrigated plot and from −0.7 to 3.8 in the non-irrigated plot (). Before the irrigation period, the average N2O flux (mean ± SD) from the irrigated plot (0.13 ± 0.63 μg N m−2 h−1; ) did not differ from that in the non-irrigated plot (1.21 ± 1.56 μg N m−2 h−1). During the irrigation period, the N2O flux from the irrigated plot (1.58 ± 1.64 μg N m−2 h−1) was significantly higher than that from the non-irrigated plot (0.21 ± 1.12 μg N m−2 h−1; P < 0.05).
Greenhouse gas concentration in the soil
The ranges of CO2 concentration of soil air at depths of 10, 20, 30 and 50 cm were 720–4,740 p.p.m.v in the irrigated plot and 900–3,600 p.p.m.v in the non-irrigated plot (). The CO2 concentrations increased with an increase in depth in both plots.
The ranges of CH4 concentration of soil air at depths of 10, 20, 30 and 50 cm were 0.30–2.07 p.p.m.v in the irrigated plot and 0.34–2.16 p.p.m.v in the non-irrigated plot (). The CH4 concentration decreased with an increase in depth in both plots.
The ranges of N2O concentration of soil air at depths of 10, 20, 30 and 50 cm were 0.30–0.33 p.p.m.v in the irrigated plot and 0.29–0.33 p.p.m.v in the non-irrigated plot (). The N2O concentration was independent of the depth of soil in both plots.
Figure 6 Temporal variation in (a) CH4 fluxes from the surface of the O-layer and (b) N2O fluxes from the surface of the O-layer of the non-irrigated (open symbols) and irrigated plots (closed symbols). Positive values indicate net emission and negative values indicate net consumption. Vertical bars denote the standard error of each parameter. Arrows indicate the irrigation events for the irrigated plot.
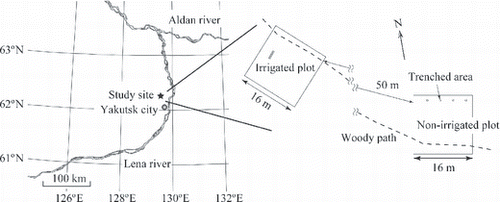
Figure 7 Temporal variation in (a,b) CO2, (c,d) CH4 and (e,f) N2O concentrations (conc.) in soil air at 10, 20, 30 and 50 cm depths of the non-irrigated (a,c,e) and irrigated plots (b,d,f). Data from 10, 20, 30 and 50 cm depths are indicated by open circles, closed circles, open triangles and closed triangles, respectively. Arrows indicate the irrigation events for the irrigated plot.
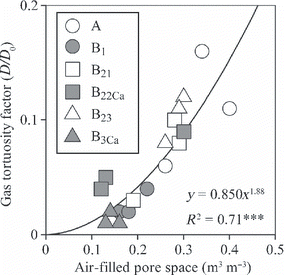
Greenhouse gas production rate
The ranges of the microbial respiration rate in the O-layer (R mo ) were 29–105 and 40–80 mg C m−2 h−1 in the irrigated and non-irrigated plots, respectively, and accounted for 26–62% (mean ± SD; 50 ± 9%) of R s (). This percentage was high at the beginning of the measurement period and decreased with an increase in soil temperature in the non-irrigated plot, whereas irrigation enhanced the rate in the irrigated plot. Before the irrigation period, the average R mo (mean ± SD) in the irrigated plot (44 ± 16 mg C m−2 h−1; ) was significantly lower than that in the non-irrigated plot (64 ± 13; P < 0.001). During the irrigation period, the average R mo in the irrigated plot (97 ± 8 mg C m−2 h−1) was significantly higher than that in the non-irrigated plot (54 ± 5; P < 0.001). The microbial respiration rate in the mineral soil (R mm ) ranged from 18 to 29 mg C m−2 h−1 in the irrigated plot and from 5 to 22 mg C m−2 h−1 in the non-irrigated plot, and accounted for 5–49% (mean ± SD; 16 ± 9%) of R s (). This percentage was high at the beginning of the measurement period and decreased with time. The average R mm values before and during the irrigation period (mean ± SD) in the irrigated plot were 24 ± 4 and 25 ± 2 mg C m−2 h−1 and both values were significantly higher than the values recorded in the non-irrigated plot (17 ± 3 and 9 ± 3 mg C m−2 h−1, respectively; P < 0.01; ).
Figure 8 Temporal variation in (a) microbial respiration rate in the O-layer and the mineral soil (Rmo are indicated by circles and Rmm are indicated by triangles, (b) CH4 production rates in the O-layer and the mineral soil (circles and triangles, respectively) and (c) N2O production rates in the O-layer and the mineral soil (circles and triangles, respectively) of the non-irrigated (open symbols) and irrigated plots (closed symbols). Positive values indicate net emission and negative values indicate net consumption. Arrows indicate the irrigation events for the irrigated plot.
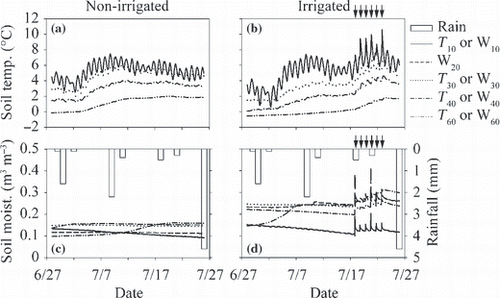
Table 3 Average global warming potentials of root respiration (Rr), microbial respiration in the O-layer (Rmo), microbial respiration in the mineral soil (Rmm), and CH4 and N2O production of each part of the soil in each period (expressed in CO2-equivalent; mg CO2-eq m−2 h−1) from the study site
The CH4 production rate in the O-layer ranged from −4 to 11 μg C m−2 h−1 in the irrigated plot and from −13 to 12 in the non-irrigated plot (). Before the irrigation period, the average CH4 production rate in the O-layer (mean ± SD) in the irrigated plot (3.6 ± 4.3 μg C m−2 h−1; ) did not differ from that in the non-irrigated plot (3.8 ± 5.4). During the irrigation period, the CH4 production rate in the irrigated plot (6.1 ± 4.0) was significantly higher than that in the non-irrigated plot (−5.7 ± 6.3; P < 0.05). The CH4 production rate in the mineral soil ranged from −17 to −8 μg C m−2 h−1 in the irrigated plot and from −17 to −5 in the non-irrigated plot (). The average CH4 production rate in the mineral soil in the irrigated plot did not differ from that in the non-irrigated plot before and during the irrigation period ().
Figure 9 (a,b) CO2 fluxes from the surface of the O-layer (Rs), (d,e) respiration from the surface of the O-layer (Rmw), (g,h) root respiration (Rr), (j,k) microbial respiration rate in the O-layer (Rmo) and (c,f,i,l) normalized Rs, Rmw, Rr and Rmo plotted against soil temperature at a depth of 10 cm (a,d,g,j) and moisture at the 0–10 cm depth (b,c,e,f,h,i,k,l). Open and closed circles indicate data from the non-irrigated and irrigated plots before irrigation and open and closed triangles indicate data from non-irrigated and irrigated plots during irrigation. †P < 0.1; *P ≤ 0.05; **P < 0.01; ***P < 0.001. Error bars indicate the standard error of each parameter.
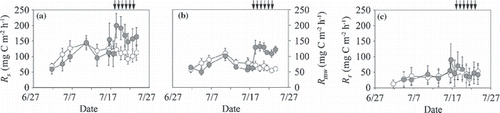
The N2O production rate in the O-layer ranged from −1.0 to 3.8 μg N m−2 h−1 in the irrigated plot and from −1.7 to 3.6 μg N m−2 h−1 in the non-irrigated plot. The N2O production rate in the mineral soil ranged from −0.2 to 0.6 μg N m−2 h−1 in the irrigated plot and from −0.6 to 0.5 in the non-irrigated plot (). Average N2O production rates in both the O-layer and the mineral soil in the irrigated plot did not differ from the rates in the non-irrigated plot before and during the irrigation period ().
Global warming potentials
Before the irrigation period, the GWP of the soil (mean ± SD) in the irrigated plot (390 ± 117 mg CO2-eq m−2 h−1) did not differ from that in the non-irrigated plot (425 ± 78). During the irrigation period, the GWP in the irrigated plot (628 ± 75) was significantly higher than that in the non-irrigated plot (398 ± 39; P < 0.001; ). Carbon dioxide production accounted for more than 99% of the GWP in both the irrigated and non-irrigated plots both before and during the irrigation period. The GWP increment resulting from irrigation was mainly caused by an increase in microbial respiration. R mo made the greatest contribution to GWP in both the irrigated and non-irrigated plots both before and during the irrigation period. The GWP by only CH4 and N2O production during the irrigation period in the non-irrigated plot was negative (−0.20 mg CO2-eq m−2 h−1) because CH4 consumption by the soil offset the GWP by N2O production. However, the GWP in the irrigated plot was positive (0.56 mg CO2-eq m−2 h−1), mainly owing to an increase in GWP by N2O production, which could not be offset by CH4 consumption.
Controlling factors for greenhouse gas fluxes
Non-linear regressions revealed a significant positive correlation between T 10 and R s , R r , R mw and R mo (r = 0.87, 0.53, 0.68 and 0.72, respectively, P < 0.01; ). No significant correlation between T 10 and R mm was recorded (r = 0.11; ). Normalized R mw and R mm values were significantly and positively correlated with W10 and W20, respectively, whereas normalized R r was significantly and negatively correlated with W10 (r = 0.64, 0.76 and −0.38, respectively, P ≤ 0.05; ). Normalized R mo had a weak positive correlation with W10 (r = 0.36, P = 0.07; ). Both the CH4 flux from the surface of the O-layer and the CH4 production rate in the O-layer had significant positive correlations with W10 (r = 0.60 and 0.62, respectively, P < 0.001; ). The CH4 production rate in the mineral soil was not correlated with either T 10 or W20 (). Each N2O flux or N2O production rate did not significantly correlate with either soil temperature or moisture ( ). The N2O flux from the surface of the O-layer had a significant positive correlation with R mw (r = 0.45, P < 0.05; ).
We carried out multiple regression analyses to evaluate the contributions of R r , R mo , R mm , CH4 production in the O-layer and the mineral soil, and N2O production in the O-layer and the mineral soil to GWP (). The results showed that R mo had the largest effect on the GWP followed by R r and R mm . This indicates that microbial activity plays an important role in controlling GWP of the soil in this region. The production rates of CH4 and N2O had little effect on the GWP.
Discussion
Effects of irrigation on soil temperature and moisture
Both soil temperature at a depth of 10 cm (temperature of the boundary between the O-layer and the mineral soil [T 10]) and soil moisture in the O-layer (W10) increased with irrigation (). The increase in T 10 results from the higher temperature of the irrigation water (approximately 15°C) than T 10 in the irrigated plot before the irrigation period (4.5°C on average). The temperature of the raindrops should be in the balance between latent heat by evaporation of raindrops and sensible heat from the atmosphere to raindrops (CitationOgura 1999). CitationAnderson et al. (1998) verified that measured raindrop temperature and wet-bulb temperature were almost equal. The mean temperature of raindrops in July in Yakutsk is calculated to be 14°C from mean air temperature (18.7°C) and mean relative humidity (60%) in July in Yakutsk (CitationJapan Meteorological Agency 2004). These values indicate that an increase in soil temperature as a result of heavy precipitation would occur in this region. Permafrost creates a strong heat sink in the summer that keeps soil temperature low (CitationEugster et al. 2000) and therefore soil temperature would increase greatly with heavy precipitation events in this region. The very low amount of precipitation in one event (2.2 mm in maximum) might be the reason why the precipitation during the experimental period did not affect the soil temperature ().
Effects of irrigation on CO2 flux and CO2 production rate
The increase in R mo as a result of irrigation was affected by the soil temperature in our study (); this result is consistent with a previous study (CitationBoone et al. 1998). In contrast, the normalized R mo value had only a weak positive effect on soil moisture (). These results indicate that the increase in R mo was mainly caused by the increase in soil temperature as a result of irrigation. In general, the microbial respiration rate increases immediately after irrigation in dry conditions, whereas the microbial respiration rate did not vary or decrease with irrigation in wet conditions (CitationHoward and Howard 1993). The weak relationship between soil moisture and normalized R mo indicated that the O-layer at this site was relatively dry, but not severely dry. CitationWaldrop and Zak (2006) mentioned that there was a trend that soil amended with NO3 −-N (7 mg kg soil−1) could enhance the soil C decomposition rate 1.5-fold. Comparing the supply of NH4 +-N and NO3 −-N to the soil by irrigation (11 and 12 mg N m−2, respectively) with the NH4 +-N and NO3 −-N contents in the O-layer (826 and 9 mg N m−2, respectively), NH4 +-N supply could be negligible, whereas the NO3 −-N supply was equivalent to 1.3-fold the NO3 −-N content in the O-layer. However, an increase in NO3 −-N concentrations in the O-layer as a result of irrigation could not exceed 0.3 mg kg soil−1 (). Therefore, it appears that the NO3 −-N amendment of soil by the irrigation water barely affected the R mo .
There was no significant correlation between the soil temperature and R mm (). In contrast, both R mm and normalized R mm were significantly and positively affected by soil moisture (). W20 in the irrigated plot was significantly higher during the irrigation period than before the irrigation period, and W20 in the non-irrigated plot was significantly lower during the irrigation period than before the irrigation period (both at P < 0.001). This indicates that the change in W20 could be the reason for the lower R mm during the irrigation period in the non-irrigated plot compared with that in the irrigated plot, even if there were no differences between R mm in the non-irrigated and irrigated plots before the irrigation period. The NH4 +-N and NO3 −-N contents in the mineral soil (9,160 and 82 mg N m−2, respectively) were much higher than those in the O-layer (826 and 9 mg N m−2, respectively), so that the effect of inflow of N from the O-layer into the mineral soil as a result of irrigation to R mm was negligible.
As a result, the positive correlation between soil temperature and R mw () was derived from the positive correlation between soil temperature and R mo . In contrast, the positive correlation between soil moisture and normalized R mw () was derived mainly from the positive correlation between soil moisture and R mm . These results indicated that a future increase in heavy precipitation events in summer, resulting in increases in soil temperature and moisture, could accelerate the decomposition of soil C in the O-layer as well as in the mineral soil.
Figure 10 (a,b) CH4 fluxes from the surface of the O-layer and (c,d) the CH4 production rate in the O-layer plotted against soil temperature at a depth of 10 cm (a,c) and moisture at the 0–10 cm depth (b,d). Open and closed circles indicate data from non-irrigated and irrigated plots before irrigation and open and closed triangles indicate data from non-irrigated and irrigated plots during irrigation. ***P < 0.001. Error bars indicate the standard error of each parameter.
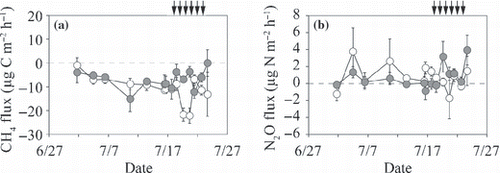
Figure 11 (a,b) N2O fluxes from the surface of the O-layer and (c,d) the N2O production rate in the O-layer plotted against soil temperature at a depth of 10 cm (a,c) and moisture at the 0–10 cm depth (b,d). Open and closed circles indicate data from non-irrigated and irrigated plots before irrigation and open and closed triangles indicate data from non-irrigated and irrigated plots during irrigation. †P < 0.1. Error bars indicate the standard error of each parameter.
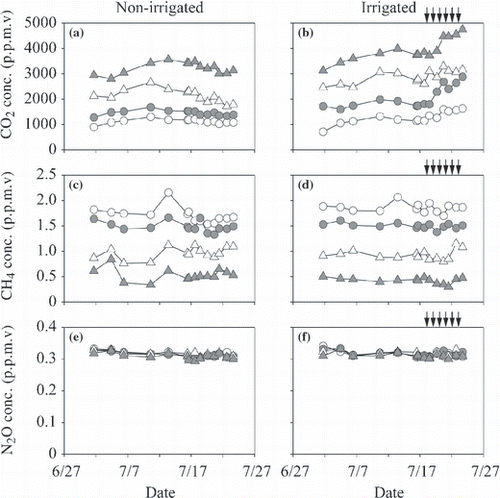
Figure 12 (a,b) Microbial respiration rate in mineral soil and (c,d) CH4 and (e,f) N2O production rates in mineral soil plotted against soil temperature at a depth of 10 cm (a,c,e) and moisture at the 0–10 cm depth (b,d,f). Open and closed circles indicate data from non-irrigated and irrigated plots before irrigation and open and closed triangles indicate data from non-irrigated and irrigated plots during irrigation. ***P < 0.001. The coefficient values written in italics were calculated using Spearman’s correlation analysis.
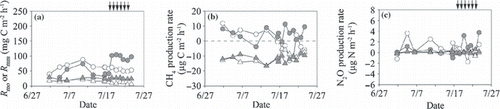
Irrigation did not increase the root respiration rate (R r ) in our study, and a negative correlation between normalized R r and soil moisture and a positive correlation between R r and soil temperature were observed (,). A very few studies reported the effect of irrigation or precipitation accompanied by an increase in soil moisture on R r . R r was depressed by surplus water owing to reduced soil oxygen diffusion (CitationBouma and Bryla 2000). A positive relationship between soil temperature and R r has been reported in many studies (CitationAtkin et al. 2000; CitationPregitzer et al. 2000). The increase in the percentage of R r to R s with soil temperature in the non-irrigated plot in our study strongly supported a positive correlation between soil temperature and R r . These results indicate that the negative effect of the increase in soil moisture as a result of irrigation on R r offset the positive effect of the increase in soil temperature on R r in this ecosystem. It is suggested that the soil in active layer investigated was too wet not to increase R r accompanied by an increase in soil temperature because of a shortage of oxygen during the irrigation period.
The R s value recorded in the present study was positively correlated with soil temperature, which increased with an increase in irrigation (). A positive relationship between soil temperature and R s has been reported in many studies (CitationBoone et al. 1998; CitationDavidson et al. 1998; CitationXu and Qi 2001). In contrast, normalized R s was not affected by an increase in soil moisture as a result of irrigation (). We concluded that an increase in soil moisture as a result of irrigation in the present study accelerated R mw , but depressed R r . Consequently, the soil moisture appeared to have no influence on R s . This indicates that the permafrost in this region has the potential to supply an adequate amount of water to plants (CitationSugimoto et al. 2002), but would not have the potential to maintain a high soil moisture, which would depress decomposition of the soil organic matter.
Figure 13 CO2 fluxes from the surface of the O-layer (Rs) plotted against N2O fluxes from the surface of the O-layer. Open and closed circles indicate data from non-irrigated and irrigated plots before irrigation and open and closed triangles indicate data from non-irrigated and irrigated plots during irrigation. *P < 0.05. Vertical and horizontal bars denote the standard error of the Rs and N2O fluxes, respectively.
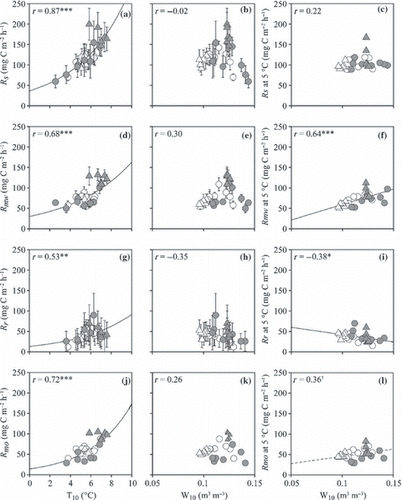
Table 4 Standardized partial regression coefficients obtained from a multiple regression analysis to evaluate the contributions of root respiration rate (Rr), microbial respiration rate from the O-layer (Rmo), microbial respiration rate from the mineral soil (Rmm), CH4 production in the O-layer and the mineral soil, and N2O production in the O-layer and the mineral soil to global warming potentials
Effects of irrigation on CH4 flux and CH4 production rate
The CH4 flux from the surface of the O-layer in the present study (from −2 to −0 μg C m−2 h−1) ranged over a higher rate than values reported from forests in northern Europe (from −143 to −1 μg C m−2 h−1; CitationSmith et al. 2000). Outside the permafrost region in Russia, CitationNakano et al. (2004) reported a CH4 flux ranging from −210 to −69 μg C m−2 h−1 in western Siberia and CitationMorishita et al. (2005) reported a CH4 flux of −63 μg C m−2 h−1 near Khabarovsk. These fluxes are much lower than the fluxes recorded in the present study. In contrast, in a permafrost region of Russia, CitationMorishita et al. (2006) reported that the CH4 flux ranged from −3.4 to −1.6 μg C m−2 h−1 in central Siberia and CitationTakakai et al. (2008) recorded a range from −10 to 3.4 μg C m−2 h−1 near Yakutsk; these results are similar to our results. CitationFlessa et al. (2008) mentioned that the lower CH4 consumption in soils with continuous permafrost can be explained by factors that hamper the diffusion of atmospheric CH4 into the soil. However, Citationvan Huissteden et al. (2008) observed CH4 fluxes ranging from −301 to −278 μg C m−2 h−1 in a forest only 1 km from our study site. The reason for this variation is unknown.
The CH4 production rate in the mineral soil was lower than that in the O-layer in the present study. This result is similar to results reported in previous studies (CitationDong et al. 1998; CitationSaari et al. 1998).
The CH4 production rate in the mineral soil did not differ between the irrigated and non-irrigated plots, despite a difference in soil moisture in these plots (,). The CH4 production rate in soil is the net result of simultaneously occurring production and consumption of CH4 within the soil (CitationButterbach-Bahl and Papen 2002; CitationYavitt et al. 1995). This indicates that the potential for both gross CH4 production and consumption rates in the mineral soil did not change with the increase in soil moisture resulting from irrigation.
In contrast, the increase in the CH4 production rate in the O-layer as a result of irrigation was affected by the soil moisture in our study (). In the irrigated plot, the CH4 production rates in the O-layer were positive during the irrigation period. This indicates that the increase in CH4 production in the O-layer as a result of irrigation in our study is caused by a change in the balance between gross CH4 production and consumption rates in the O-layer as a result of an increase in soil moisture. The CH4 production rates in the O-layer were estimated from two types of fluxes measured with different methods, so these rates include various assumptions. However, there is a possibility that a significantly higher CH4 flux from the surface of the O-layer in the irrigated plot compared with that in the non-irrigated plot during the irrigation period () resulted from a change in the CH4 production rate in the O-layer, because the CH4 production rate in the mineral soil was not affected by the irrigation. The effect of the addition of NH4 + in the irrigation water should not be significant because the NH4 + content in the O-layer was much higher than that added by the irrigation (826 and 11 mg N m−2, respectively). In general, a negative correlation between soil moisture and CH4 flux is observed under dry conditions and a positive correlation between them is observed under wet conditions (CitationCurry 2007; CitationStriegl et al. 1992). In our study, soil dryness was not a limiting factor for CH4 consumption.
Effects of irrigation on N2O flux and N2O production rate
The N2O flux from the surface of the O-layer (from −1.7 to 3.9 μg N m−2 h−1) ranged over a lower rate than previously reported values in boreal forests (from −4.9 to 22.3; CitationDalal and Allen 2008). Previous studies conducted near Yakutsk also reported very low values (from −2.1 to 4.6; CitationMorishita et al. 2007; CitationTakakai et al. 2008). These low values show characteristics of an N-limited region (CitationHatano et al. 2001; CitationSchulze et al. 1995). The much larger N2O production in the O-layer than in the mineral soil observed in the present study was consistent with previous studies (CitationSaari et al. 1997).
A number of studies have reported a positive correlation between soil moisture and temperature and N2O flux from the surface of the O-layer in natural forests (e.g. CitationDong et al. 1998; CitationFest et al. 2009). Although the N2O flux during the irrigation period in our study was higher in the irrigated plot than in the non-irrigated plot, the N2O fluxes did not correlate with either soil moisture or temperature (,). Only a weak positive correlation between the N2O flux from the surface of the O-layer and soil temperature was found (P = 0.08). The N2O production rates in the O-layer and the mineral soil did not differ between the irrigated and non-irrigated plots both before and during the irrigation period and did not correlate significantly with either soil moisture or soil temperature. In contrast, the N2O flux from the surface of the O-layer was positively correlated with R mw (P < 0.05; ). This indicates that the main cause of an increase in the N2O flux from the surface of the O-layer as a result of irrigation was not a direct effect of either soil temperature or moisture, but rather an indirect effect of microbial activities. Comparing NH4 +-N and NO3 −-N supply to the soil by irrigation (11 and 12 mg N m−2, respectively) with the NH4 +-N and NO3 −-N contents in the O-layer, which produced most of the N2O for the whole soil (826 and 9 mg N m−2, respectively), NH4 +-N supply was negligible, whereas NO3 −-N supply was comparable with the NO3 −-N content in the O-layer. This indicates that NO3 −-N supply by irrigation might stimulate N2O emission via an increase in denitrification in the irrigated plot. CitationLiu et al. (2008) could not detect variation in the N2O flux from the surface of the O-layer after irrigation owing to the lack of substrate for denitrification process on grassland in Inner Mongolia. The weak relationship between N2O flux from the surface of the O-layer and both soil temperature and moisture in the present study might be caused by a deficiency of N. CitationDu et al. (2006) also observed no significant linear relationship between both soil temperature and moisture and diurnal N2O flux from the surface of the O-layer in grassland in Inner Mongolia. However, these researchers mentioned that it was the distribution of effective precipitation, rather than the precipitation intensity, which influenced seasonal and inter-annual variations in N2O flux. Even in the ecosystem investigated in the present study, observations over a longer period are needed to examine the effects of precipitation on N2O flux from the surface of the O-layer.
Conclusions
In the investigated Taiga forest near Yakutsk, which is characterized by low soil temperature and dry weather, heavy precipitation events would increase both soil temperature and moisture. The increased soil temperature and moisture would accelerate microbial activities, which play an important role in controlling the GWP in the soil, resulting in decomposition of soil organic matter in both the O-layer and the mineral soil. As a result, the release of inorganic N into the soil together with an increase in N2O emission, which has a huge impact on the GWP of the soil, would be expected. Continuous study on the effect of changes in precipitation patterns on the decomposition of soil organic matter accompanied by N mineralization is crucial in predicting the future GWP of soil in this region. In addition, an increase in the gross CH4 production rate might decrease the potential of the soil to act as a CH4 sink. Further study is required to investigate changes in CH4 production in the O-layer caused by heavy rain events.
Acknowledgments
We are deeply grateful to the staff members of the Institute for Biological Problems of Cryolithozone, Siberian Branch, Russian Academy of Science, for their support during the field research. This study was funded by the RR2002 Program of the Japanese Ministry of Education, Culture, Sports, Science and Technology.
References
- Anderson , SP , Hinton , A and Weller , RA . 1998 . Moored observations of precipitation temperature . Journal of Atmospheric and Oceanic Technology , 15 : 979 – 986 .
- Atkin , OK , Edwards , EJ and Loveys , BR . 2000 . Response of root respiration to changes in temperature and its relevance to global warming . New Phytol. , 147 : 141 – 154 .
- Bond-Lamberty , B , Wang , CK and Gower , ST . 2004 . A global relationship between the heterotrophic and autotrophic components of soil respiration? . Global Change Biol. , 10 : 1756 – 1766 .
- Boone , RD , Nadelhoffer , KJ , Canary , JD and Kaye , JP . 1998 . Roots exert a strong influence on the temperature sensitivity of soil respiration . Nature , 396 : 570 – 572 .
- Borken , W , Davidson , EA , Savage , K , Gaudinski , J and Trumbore , SE . 2003 . Drying and wetting effects on carbon dioxide release from organic horizons . Soil Sci. Soc. Am. J. , 67 : 1888 – 1896 .
- Bouma , TJ and Bryla , DR . 2000 . On the assessment of root and soil respiration for soils of different textures: interactions with soil moisture contents and soil CO2concentrations . Plant Soil , 227 : 215 – 221 .
- Butterbach-Bahl , K and Papen , H . 2002 . Four years continuous record of CH4-exchange between the atmosphere and untreated and limed soil of a N-saturated spruce and beech forest ecosystem in Germany . Plant Soil , 240 : 77 – 90 .
- Curry , CL . 2007 . Modeling the soil consumption of atmospheric methane at the global scale . Global Biogeochem. Cycles , 21 : GB4012
- Dalal , RC and Allen , DE . 2008 . Greenhouse gas fluxes from natural ecosystems . Aust. J. Bot. , 56 : 369 – 407 .
- Davidson , EA , Belk , E and Boone , RD . 1998 . Soil water content and temperature as independent or confounded factors controlling soil respiration in a temperate mixed hardwood forest . Global Change Biol. , 4 : 217 – 227 .
- Davidson , EA and Trumbore , SE . 1995 . Gas Diffusivity and Production of CO2in Deep Soils of the Eastern Amazon . Tellus B Chem. Phys. Meteorol. , 47 : 550 – 565 .
- Dong , Y , Scharffe , D , Lobert , JM , Crutzen , PJ and Sanhueza , E . 1998 . Fluxes of CO2, CH4and N2O from a temperate forest soil: the effects of leaves and humus layers . Tellus B Chem. Phys. Meteorol. , 50 : 243 – 252 .
- Du , R , Lu , DR and Wang , GC . 2006 . Diurnal, seasonal, and inter-annual variations of N2O fluxes from native semi-arid grassland soils of inner Mongolia . Soil Biol. Biochem. , 38 : 3474 – 3482 .
- Easterling , DR , Evans , JL Groisman , PY . 2000 . Observed variability and trends in extreme climate events: a brief review . Bull. Am. Meteorol. Soc. , 81 : 417 – 425 .
- Eugster , W , Rouse , WR Pielke , RA . 2000 . Land-atmosphere energy exchange in Arctic tundra and boreal forest: available data and feedbacks to climate . Global Change Biol. , 6 : 84 – 115 .
- Fest , BJ , Livesley , SJ , Drosler , M , van Gorsel , E and Arndt , SK . 2009 . Soil-atmosphere greenhouse gas exchange in a cool, temperate Eucalyptus delegatensis forest in south-eastern Australia . Agricultural and Forest Meteorology , 149 : 393 – 406 .
- Flessa , H , Rodionov , A Guggenberger , G . 2008 . Landscape controls of CH4fluxes in a catchment of the forest tundra ecotone in northern Siberia . Global Change Biol. , 14 : 2040 – 2056 .
- Goodale , CL , Apps , MJ Birdsey , RA . 2002 . Forest carbon sinks in the Northern Hemisphere . Ecol. Appl. , 12 : 891 – 899 .
- Hatano , R , Sawamoto , T Shibuya , M . 2001 . “ Characteristics of nitrogen cycling in Permafrost regional ecosystems in Yakutsk, Russia ” . In Ninth Symposium on the Joint Siberian Permafrost Studies between Japan and Russia in 2000 , Edited by: Fukuda , M and Kobayashi , Y . 99 – 103 . Japan : Sapporo .
- Howard , DM and Howard , PJA . 1993 . Relationships between CO2evolution, moisture-content and temperature for a range of soil types . Soil Biol. Biochem. , 25 : 1537 – 1546 .
- van Huissteden , J , Maximov , TC , Kononov , AV and Dolman , AJ . 2008 . Summer soil CH4emission and uptake in taiga forest near Yakutsk, Eastern Siberia . Agricultural and Forest Meteorology , 148 : 2006 – 2012 .
- IPCC . 2007 . Climate change 2007: The Physical Science Basis. Contribution of Working Group I to the Fourth Assessment Report of the Intergovernmental Panel on Climate Change , Cambridge, , USA : Cambridge University Press . United Kingdom and New York, NY
- Japan Meteorological Agency . 2004 . Monthly Report of the Japan Meteorological Agency , Tokyo : Japan Meteorological Agency .
- Kusa , K , Sawamoto , T , Hu , R and Hatano , R . 2008 . Comparison between closed-chamber and gas concentration gradient methods for measurement of CO2and N2O fluxes in two upland field soils . Soil Sci. Plant Nutr. , 54 : 777 – 785 .
- Liu , CY , Holst , J Bruggemann , N . 2008 . Effects of Irrigation on Nitrous Oxide, Methane and Carbon Dioxide Fluxes in an Inner Mongolian Steppe . Advances in Atmospheric Sciences , 25 : 748 – 756 .
- Lopez , MLC , Saito , H Kobayashi , Y . 2007 . Interannual environmental-soil thawing rate variation and its control on transpiration from Larix cajanderi, Central Yakutia, Eastern Siberia . J. Hydrol. , 338 : 251 – 260 .
- Millard , P , Midwood , AJ , Hunt , JE , Whitehead , D and Boutton , TW . 2008 . Partitioning soil surface CO2efflux into autotrophic and heterotrophic components, using natural gradients in soil delta C-13 in an undisturbed savannah soil . Soil Biol. Biochem. , 40 : 1575 – 1582 .
- Morishita , T , Hatano , R and Desyatkin , RV . 2007 . N2O Flux in Alas Ecosystems Formed by Forest Disturbance Near Yakutsk, Eastern Siberia, Russia . Eurasian Journal of Forest Research , 10 : 79 – 84 .
- Morishita , T , Hatano , R , Takahashi , K and Kondrashov , LG . 2005 . Effect of deforestation on CH4uptake in Khabarovsk, Far East, Russia . Phyton , 45 : 267 – 274 .
- Morishita , T , Matsuura , Y , Zyryanova , OA and Abaimov , AP . 2006 . “ CO2, CH4, and N2O fluxes from a larch forest soil in Central Siberia ” . In Symptom of Environmental Change in Siberian Permafrost Region , Edited by: Hatano , R and Guggenberger , G. 1 – 9 . Sapporo, , Japan : Hokkaido University Press .
- Nakano , T , Inoue , G and Fukuda , M . 2004 . Methane consumption and soil respiration by a birch forest soil in West Siberia . Tellus B Chem. Phys. Meteorol. , 56 : 223 – 229 .
- Oberbauer , SF , Gillespie , CT Cheng , W . 1992 . Environmental-Effects on CO2Efflux from Riparian Tundra in the Northern Foothills of the Brooks Range, Alaska, USA . Oecologia , 92 : 568 – 577 .
- Ogura , Y . 1999 . General Meteorology (in Japanese) , Tokyo : University of Tokyo Press .
- Ohta , T , Hiyama , T Tanaka , H . 2001 . Seasonal variation in the energy and water exchanges above and below a larch forest in eastern Siberia . Hydrol. Process. , 15 : 1459 – 1476 .
- Ono , R . 2003 . Examination of presuming method of the length of the line contained in a space, and the application in investigation of tea plant rootlet (in Japanese with English summary) . Root Research , 12 : 119 – 123 .
- Osozawa , S and Kubota , T . 1987 . Method or measuring soil gas diffusion coefficient (in Japanese) . Japanese Journal of Soil Science and Plant Nutrition , 58 : 528 – 535 .
- Pihlatie , M , Pumpanen , J Rinne , J . 2007 . Gas concentration driven fluxes of nitrous oxide and carbon dioxide in boreal forest soil . Tellus B Chem. Phys. Meteorol. , 59 : 458 – 469 .
- Potter , CS , Davidson , EA and Verchot , LV . 1996 . Estimation of global biogeochemical controls and seasonality in soil methane consumption . Chemosphere , 32 : 2219 – 2246 .
- Pregitzer , KS , King , JA , Burton , AJ and Brown , SE . 2000 . Responses of tree fine roots to temperature . New Phytol. , 147 : 105 – 115 .
- R Development Core Team . 2009 . R: A Language and Environment for Statistical Computing , Vienna, Austria : R Foundation for Statistical Computing . URL: http://www.R-project.org
- Reid , RC , Prausnitz , JM and Sherwood , TK . 1977 . The Properties of Gases and Liquids , New York : McGraw-Hill .
- Rodionow , A , Flessa , H , Kazansky , O and Guggenberger , G . 2006 . Organic matter composition and potential trace gas production of permafrost soils in the forest tundra in northern Siberia . Geoderma , 135 : 49 – 62 .
- Saari , A , Heiskanen , J and Martikainen , PJ . 1998 . Effect of the organic horizon on methane oxidation and uptake in soil of a boreal Scots pine forest . FEMS Microbiol. Ecol. , 26 : 245 – 255 .
- Saari , A , Martikainen , PJ Ferm , A . 1997 . Methane oxidation in soil profiles of Dutch and Finnish coniferous forests with different soil texture and atmospheric nitrogen deposition . Soil Biol. Biochem. , 29 : 1625 – 1632 .
- Schulze , ED , Schulze , W Kelliher , FM . 1995 . Aboveground Biomass and Nitrogen Nutrition in a Chronosequence of Pristine Dahurian Larix Stands in Eastern Siberia . Canadian Journal of Forest Research-Revue Canadienne De Recherche Forestiere , 25 : 943 – 960 .
- Schwendenmann , L and Veldkamp , E . 2006 . Long-term CO2production from deeply weathered soils of a tropical rain forest: evidence for a potential positive feedback to climate warming . Global Change Biol. , 12 : 1878 – 1893 .
- Sirotenko , OD and Abashina , EV . 2008 . Modern Climate Changes of Biosphere Productivity in Russia and Adjacent Countries . Russian Meteorology and Hydrology , 33 : 267 – 271 .
- Smith , KA , Dobbie , KE Ball , BC . 2000 . Oxidation of atmospheric methane in Northern European soils, comparison with other ecosystems, and uncertainties in the global terrestrial sink . Global Change Biol. , 6 : 791 – 803 .
- Soil Survey Staff . 2010 . Soil taxonomy. A Basic System of Soil Classification for Making and Interpreting Soil Surveys , 11th edn , Washington, DC : US Gov Printing Office .
- Striegl , RG , Mcconnaughey , TA , Thorstenson , DC , Weeks , EP and Woodward , JC . 1992 . Consumption of Atmospheric Methane by Desert Soils . Nature , 357 : 145 – 147 .
- Sugimoto , A , Yanagisawa , N , Naito , D , Fujita , N and Maximov , TC . 2002 . Importance of permafrost as a source of water for plants in east Siberian taiga . Ecol. Res. , 17 : 493 – 503 .
- Takakai , F , Desyatkin , AR , Lopez , CML , Fedorov , AN , Desyatkin , RV and Hatano , R . 2008 . Influence of forest disturbance on CO2, CH4and N2O fluxes from larch forest soil in the permafrost taiga region of eastern Siberia . Soil Sci. Plant Nutr. , 54 : 938 – 949 .
- Takle , ES , Massman , WJ Brandle , JR . 2004 . Influence of high-frequency ambient pressure pumping on carbon dioxide efflux from soil . Agricultural and Forest Meteorology , 124 : 193 – 206 .
- Waldrop , MP and Zak , DR . 2006 . Response of oxidative enzyme activities to nitrogen deposition affects soil concentrations of dissolved organic carbon . Ecosystems , 9 : 921 – 933 .
- Xu , M and Qi , Y . 2001 . Soil-surface CO2efflux and its spatial and temporal variations in a young ponderosa pine plantation in northern California . Global Change Biol. , 7 : 667 – 677 .
- Yavitt , JB , Fahey , TJ and Simmons , JA . 1995 . Methane and Carbon-Dioxide Dynamics in a Northern Hardwood Ecosystem . Soil Sci. Soc. Am. J. , 59 : 796 – 804 .