Abstract
Nitrous oxide (N2O) emissions were measured and nitrogen (N) budgets were estimated for 2 years in the fertilizer, manure, control and bare plots established in a reed canary grass (Phalaris arundinacea L.) grassland in Southern Hokkaido, Japan. In the manure plot, beef cattle manure with bark was applied at a rate of 43–44 Mg fresh matter (236–310 kg N) ha−1 year−1, and a supplement of chemical fertilizer was also added to equalize the application rate of mineral N to that in the fertilizer plots (164–184 kg N ha−1 year−1). Grass was harvested twice per year. The total mineral N supply was estimated as the sum of the N deposition, chemical fertilizer application and gross mineralization of manure (GMm), soil (GMs), and root-litter (GMl). GMm, GMs and GMl were estimated by dividing the carbon dioxide production derived from the decomposition of soil organic matter, root-litter and manure by each C : N ratio (11.1 for soil, 15.5 for root-litter and 23.5 for manure). The N uptake in aboveground biomass for each growing season was equivalent to or greater than the external mineral N supply, which is composed of N deposition, chemical fertilizer application and GMm. However, there was a positive correlation between the N uptake in aboveground biomass and the total mineral N supply. It was assumed that 58% of the total mineral N supply was taken up by the grass. The N supply rates from soil and root-litter were estimated to be 331–384 kg N ha−1 year−1 and 94–165 kg N ha−1 year−1, respectively. These results indicated that the GMs and GMl also were significant inputs in the grassland N budget. The cumulative N2O flux for each season showed a significant positive correlation with mineral N surplus, which was calculated as the difference between the total mineral N supply and N uptake in aboveground biomass. The emission factor of N2O to mineral N surplus was estimated to be 1.2%. Furthermore, multiple regression analysis suggested that the N2O emission factor increased with an increase in precipitation. Consequently, soil and root-litter as well as chemical fertilizer and manure were found to be major sources of mineral N supply in the grassland, and an optimum balance between mineral N supply and N uptake is required for reducing N2O emission.
Introduction
Grassland is one of the world’s most abundant land cover types accounting for approximately 40.5% of the earth’s terrestrial land area, excluding the areas of permanent ice cover (CitationAdams et al. 1990; CitationWhite et al. 2000). Grassland is an important ecosystem to support the production of herbivorous livestock (CitationSoussana et al. 2007). Application of chemical fertilizer and animal manure to grasslands has increased grass production, especially in developed countries where grassland-based livestock production is important (CitationBouwman et al. 2002). However, Nitrogen (N) application to grasslands also poses a risk of N loss to the environment in the form of nitrous oxide (N2O) emission and nitrate leaching (CitationBouwman 1996; CitationJordan et al. 1997).
Nitrous oxide is a potent greenhouse gas, which has a global warming potential of 298 times that of carbon dioxide (CO2) over a 100-year time horizon (CitationIPCC 2007). Furthermore, N2O destroys the stratospheric ozone layer (CitationCrutzen 1974). N2O concentration in the atmosphere has increased from about 270 ppbv in the preindustrial era to 319 ppbv in 2005 (CitationIPCC 2007). Global N2O sources have been estimated to be 17.7 Tg N year−1 (CitationIPCC, 2007). N2O emission from fertilized agricultural fields amounted to 2.8 Tg N year−1, which is the biggest anthropogenic N2O source (CitationIPCC 2007). Isotopic measurements made on polar firn air also suggested that the anthropogenic N2O sources have been dominated by agricultural emissions (CitationRahn and Wahlen 2000; CitationRockmann et al. 2003).
Nitrous oxide emissions from soils result principally from microbial nitrification and denitrification (CitationBouwman 1990). These processes require mineral N (NH4 + and NO3 −) as a substrate, and are controlled by soil moisture content, temperature, pH and organic carbon (CitationBouwman 1990; CitationMaag and Vinther 1996; CitationMosier 1998; CitationYamulki et al. 1997). In managed grassland, the sources of mineral N are considered to be chemical fertilizer, manure, soil organic matter, plant litter, N fixation and atmospheric deposition (CitationDam Kofoed 1981; CitationDubeux et al. 2007). Therefore, estimating the supply rate of mineral N from each source and clarifying the factors controlling N2O emissions can be helpful in establishing environmental-friendly N management.
In the grasslands of Southern Hokkaido, Japan, which were used for this study, carbon budgets have been measured using an ecological technique (CitationShimizu et al. 2009). We found that the CO2 fluxes from soil surface including roots in the fertilizer, manure and control plots were higher in spring (beginning of March to end of June) than in other seasons (beginning of July to end of February) at the same soil temperature, while the relationship between CO2 flux and soil temperature in the root-excluded bare plot was well explained by a single exponential regression throughout the year. This suggests that there might be an increase in root respiration or heterotrophic respiration of litter produced by fine roots. If the increment in spring was caused by root respiration, the root respiration would not have been counted in the carbon budget. If it was caused by heterotrophic respiration of fine root litter, the heterotrophic respiration was offset by the fine root production because of an unclear yearly change, and had no effect on the estimation of carbon budget. Therefore, we concluded that either root respiration or heterotrophic respiration had no effect on the carbon budget in our estimations. However, the distinction between the heterotrophic respiration and root respiration has a great influence on N budgets. Heterotrophic respiration accompanies N mineralization (CitationSchimel 1986); while an increase in root respiration does not enhance the N mineralization. Also, the processes of nitrification and denitrification after N mineralization would have a major influence on N2O emission (CitationBouwman 1990), and the determination of the source of CO2 emission is an important factor when clarifying the source of N2O emission. Actually, a positive relationship between CO2 and N2O emissions was reported by several field studies (CitationChu et al. 2007; CitationToma and Hatano 2007) and a laboratory incubation (CitationHashidoko et al. 2007).
The objectives of the present study were to identify the sources of mineral N, their supply rate in managed grasslands and to clarify the factors controlling N2O emission from the grassland.
Materials and Methods
Study site
This study was conducted for 2 years from mid April 2005 to early May 2007 in managed grasslands located on the Shizunai Experimental Livestock Farm, Field Science Center for the Northern Biosphere of Hokkaido University in Southern Hokkaido, Japan (42°26′N, 142°29′E). The site is characterized by a humid continental climate with cold winters and cool summers but without apparent wet or dry seasons. The mean annual precipitation is 1365 mm and the mean annual temperature is 7.9°C, with the mean monthly temperature ranging from 20.7°C in August to −3.9°C in January. The site is covered with snow from the end of December to the beginning of March.
The soil is derived from Tarumae (b) volcanic ash, and is classified as Thaptic Melanudands (CitationSoil Survey Staff 2006; Mollic Andosol (CitationIUSS Working Group WRB 2006)). A layer of 3-cm thick root-mat was found on the top, and a 21-cm thick Ap-layer was found under the root-mat in a survey conducted in August 2004. The C and N contents in the Ap-layer were 3.7% and 0.33%, respectively, and the C : N ratio was 11.1.
The grassland was established more than 30 years ago and has been continuously used as grassland. The average of chemical fertilizer application rates from 1984 to 2004 was 133 ± 36 kg N ha−1 year−1. The dominant species of the grassland were reed canary grass (Phalaris arundinacea L.) and meadow foxtail (Alopecurus pratensis L.), and the percentage of legume was <2.9% (). Harvesting was carried out twice a year (21 June and 11 August in 2005 and 27 June and 23 August in 2006) in accordance with the local practice.
Study period
We defined the crop growing season as the period of time with 7-day moving average of daily air temperature above 5°C and the non-growing (NG) season as the rest. The growing season was 215 days in 2005 (From 10 April 2005 to 10 November 2005) and 218 days in 2006 (From 15 April 2006 to 18 November 2006), and the NG season was 155 days in 2005 and 151 days in 2006. The growing season was divided into three parts; from the beginning of the growing season to the first crop harvest (G1), from the first harvest to the second harvest (G2), and from the second harvest to the end of the growing season (G3). The divided growing season was 72 days in 2005 and 73 days for G1, 51 days in 2005 and 57 days for G2, and 92 days in 2005 and 88 days in 2006 for G3. The non-growing season was 155 days in 2005 and 151 days in 2006.
Table 1 The botanical composition of grassland (dry matter weight percentage) on the study site
Treatment
Four experimental plots were set up on the study site, one for treatment with chemical fertilizer (fertilizer plot), another with both beef cattle manure and chemical fertilizer (manure plot), a third with no fertilizer or manure (control plot) and the other with no plants (bare plot). Neither manure nor fertilizer was applied to the bare plot. The treatments were initiated in the spring of 2005. Eighteen subplots (5 × 4 m) were established for the fertilizer, manure and control plots with six replications, and six subplots (1 × 1 m) for the bare plot (). In the bare plot, the plants aboveground and roots were removed by hand, with a shovel and a root-proofing permeable sheet (BKS9812, TOYOBO, Osaka, Japan) was vertically inserted to a depth of 20 cm below the ground surface to inhibit regrowth of roots. The plants grown on the bare plot were removed every couple of weeks.
shows the information on the date of application and the application rates of fertilizer and manure. The fertilizer application rates in the fertilizer plot were at the recommended level for this site on the basis of soil diagnosis, and were 164 kg N ha−1 year−1 in 2005 and 184 kg N ha−1 year−1 in 2006 as ammonium sulfate and ammonium phosphate (). The manure application rates were based on adequate amounts of potassium application to the fields. Beef cattle manure with bedding litter (bark) was applied to the manure plot and the application rates were 44 Mg FM ha−1 (236 kg T-N ha−1 and 5.8 Mg T-C ha−1) in May 2005 and 43 Mg FM ha−1 (310 kg T-N ha−1 and 6.0 Mg T-C ha−1) in May 2006 (). In the manure plot, the nutrient supply rates from manure were estimated by multiplying the application rate by the mineralization rate (), and the differences between the supply rates in manure and the application rates in the fertilizer plot were supplied by chemical fertilizer. The N mineralization rates were estimated based on Uchida’s model (CitationShiga et al. 1985), which was developed in Japan and were 13.2 and 7.0%, respectively, in the first and second years after application (). The mineralization rates of phosphorus and potassium from the manure were estimated based on the handbook of animal waste management and utilization in Hokkaido 2004 (CitationHokkaido Prefectural Experiment Stations and Hokkaido Animal Research Center 2004). The phosphorus mineralization rate was 20% in the first year and 10% in the second year (). The potassium mineralization rate was 70% in the first year and 10% in the second year ().
Table 2 The applied date and the application rates of chemical fertilizer and manure
Table 3 Mineralization rates from the cattle manure
Nitrogen deposition
A rainfall collector (0.9 m length, 0.1 m width) with a tank (20 L) was set up, and the water sample was collected once or twice each month from April to November (CitationMorishita et al. 2004). A bucket (0.3 m diameter, 0.4 m depth) was set up to collect snowfall, and the water sample was collected once a month. Thymol (C10H14O) was used as a biocide in the tank or bucket to prevent biological alteration and utilization of the N species (CitationGillett and Ayers 1991). All of the samples were filtered through a membrane filter (0.2 μm) and kept at 4°C before analysis. NH4 +-N content was determined by the indophenol-blue method (UV mini 1240; Shimadzu, Kyoto, Japan), and NO3 −-N content was analyzed by ion chromatography (Dionex QIC Analyzer; Dionex Japan, Osaka, Japan). The amount of N deposition was calculated by multiplying the amount of water by NH4 +-N and NO3 −-N concentrations.
Gross N mineralization
The soil organic matter, root-litter and manure are supposed to release mineral N during decomposition in the managed grassland, and gross N mineralization (GM) was estimated using the following equation.
The RH from soil organic matter, root-litter and manure was previously estimated using the static closed chamber method on this study site (CitationShimizu et al. 2009). These measurements were carried out at the same time with the measurement of N2O and nitric oxide (NO) fluxes as described below. RH from the soil organic matter (RHs) was estimated using the exponential regression model for the bare plot driven by the soil temperature at each plot. The RH from manure (RHm) was estimated as the difference in soil respiration, which is a CO2 flux from the soil surface composed of RH and root respiration, between manure and fertilizer plots. The RH from root-litter (RHl) was assumed as the following. Soil respiration in the fertilizer, manure and control plots was higher in spring (beginning of March–end of June) than in the other season (beginning of July–end of February) at the same soil temperature (CitationShimizu et al. 2009). We hypothesized that the increment of a CO2 flux in the spring was RHl. To estimate the increment of a CO2 flux in spring, we calculated the cumulative CO2 flux in spring using the exponential regression equation of the two seasons driven by the soil temperature in spring, and estimated the difference between the cumulative CO2 fluxes (CitationShimizu et al. 2009).
We used a C : N ratio of soil in the Ap-layer (11.1) to estimate GMs. Roots of <2 mm in diameter were obtained four times in 2006 (mid April, late June, mid August and late October), and the C : N ratio was analyzed using an N/C analyzer (SUMIGRAPH NC-1000; Sumika Chemical Analysis Service, Ltd., Osaka, Japan). The average value was 15.5, and we used it for the C : N ratio of root-litter to estimate the GMl. The C : N ratio of manure was 25 for 2005, and was assumed to be 22 for 2006, which was the average of 2 years, because manure applied in 2005 have remained in 2006.
N uptake in aboveground biomass
The aboveground biomass, which includes live and dead parts, was measured four times a year; in mid April, late June (before the first crop harvest), mid August (before the second crop harvest) and in late October. The aboveground biomass at the time of the harvest was estimated to be the sum of the harvests and the residue. The harvest was measured by clipping at 5 cm above the ground using the 100 × 100 cm quadrates, and the harvest residue and the aboveground biomass in April and October were measured by clipping using the 50 × 50 cm quadrates, with six replications conducted in 2005 and eight replications conducted in 2006. All the samples were oven-dried at 70°C for 72 h and weighed. Each dried sample was analyzed for the total N content with an N/C analyzer (SUMIGRAPH NC-1000; Sumika Chemical Analysis Service, Ltd., Osaka, Japan).
The N uptake in aboveground biomass was estimated as an increment in biomass N during each season. Apparent N recovery (ANR) (kg N (kg N)−1) was estimated using the following equation (CitationKaffka and Kanneganti 1996):
N2O and NO fluxes
Nitrous oxide and NO fluxes from the soil to the atmosphere were measured by the static closed chamber method on the fertilizer, manure, control and bare plots (CitationShimizu et al. 2009). The flux measurements were conducted in two 28-day intervals during the crop growing season and 10 30-day intervals during the non-growing season and between 08.00 and 11.00 hours each measuring day to minimize the effect of diurnal temperature variation. The stainless steel chambers were 40 cm in diameter and 30 cm high in the fertilizer and manure plots, and 20 cm in diameter and 25 cm high in the control and bare plots. The chambers were placed directly into the soil to a depth of about 3 cm, 12 h before the measurement of each subplot, and contained no aboveground biomass in the fertilizer, manure and control plots. Before closing the chamber, a 250-mL gas sample from the headspace of each chamber was extracted into a Tedlar bag for NO analysis, and a 20-mL gas sample was injected into an evacuated vial (10 mL) for N2O analysis. This measurement was regarded as time 0 min. After 20 min or 30 min under a closed-chamber condition, 250 mL of the headspace gas sample was extracted from each chamber into a bag, and 20 mL was injected into a vial. From these bag samples, NO gas concentrations were determined in a laboratory within 16 h using a chemiluminescence nitrogen oxides analyzer (Model 265P; Kimoto Electric, Osaka, Japan). N2O gas concentrations were determined in a laboratory within one month using a gas chromatograph (Model GC-14B; Shimadzu, Kyoto, Japan) fitted with an electron capture detector.
Environmental variables
Daily precipitation and daily maximum depths of snow were obtained at the closest AMeDAS (Automated Meteorological Data Acquisition System) station by the Japan Meteorological Agency. Air temperature and soil temperature at a 5-cm depth were measured at the same time with the flux measurements using a thermistor thermometer (CT220; CUSTOM, Tokyo, Japan), and soil moisture content at a 0–6 cm depth was measured using the Frequency Domain Reflectometry (FDR) method (DIK-311A; Daiki, Saitama, Japan). Soil core samples (14 cm diameter, 13 cm height) were collected in April 2007, and calibration curves were made to calculate water-filled pore space (WFPS) from the FDR device reading (m3 m−3) and percent total porosity (CitationLinn and Doran 1984). The percent total porosity was measured using a 100-mL soil core collected in April 2007 and was regarded as constant throughout the study period because of no tillage.
Statistical analyses
Statistical analyses were carried out with Prism ver. 4 (GraphPad Software Inc., San Diego, CA, USA) and Excel Statistics version 5.0 (Esumi; Tokyo, Japan). The differences in soil temperature, WFPS and N2O flux among the plots were analyzed with a repeated-measures one-way analysis of variance (anova) and Bonferroni test. The differences in GMs, GMl, GMm, total mineral N supply, N uptake in aboveground biomass and cumulative N2O flux among the seasons were analyzed with a repeated-measures anova and Bonferroni test. The differences in the annual values between the years and the plots were analyzed with a two-way factorial anova and Bonferroni test.
Uncertainties in the N uptake in aboveground biomass and the cumulative N2O flux were calculated using the following equation:
The two-sided 95% confidence interval was calculated by using the following equation:
Results
Environmental variables
Daily precipitation and daily maximum depths of snow are shown in . Annual precipitations were 1152 mm and 1047 mm from mid April 2005 to the beginning of April 2006 and from mid April 2006 to the beginning of April 2007, respectively. These values are smaller than the 12-year average (1365 ± 215 mm), but drought did not occur.
Figure 2 Seasonal variations in meteorological variables and the N2O and NO flux; Daily precipitation and daily maximum snow depth (a), soil temperature at a 5-cm depth (b), water-filled pore space (WFPS) (c), N2O flux (d) and NO flux (e). Data of soil temperature, WFPS, N2O flux and NO flux represent means ± standard deviation (SD) (n = 6). The arrows indicate the timing of fertilizer or manure application.
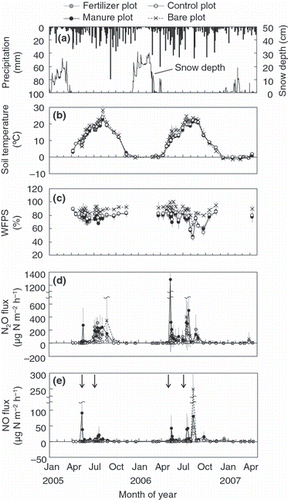
The soil temperature at 5 cm depth increased from April, reaching the maximum temperature from July through August, and then decreasing gradually (). The soil temperature was around 0°C from December to March (). There was no difference in soil temperature between the fertilizer and manure plots, but soil temperature was higher in the control plot than in the fertilizer and manure plots (P < 0.05). Soil temperature in the bare plot was the highest of all plots (P < 0.01).
The range of WFPS in a 0–6 cm depth in 2005 was 67–89% in the fertilizer plot, 67–90% in the manure plot, 72–89% in the control plot and 83–94% in the bare plot (). The WFPS in the fertilizer, manure, control and bare plots decreased to 47%, 48%, 46% and 65%, respectively, in mid August 2006, and then increased with an increase in precipitation. There was no difference in the WFPS between the fertilizer and manure plots; however, it was greater in the control plot than in the fertilizer and manure plots (P < 0.01). Furthermore, the WFPS in the bare plot was found to be the greatest of all the plots (P < 0.01).
N deposition
The annual N deposition was 15 kg N ha−1 year−1 in 2005 and 4 kg N ha−1 year−1 in 2006 ().
Gross N mineralization
There was a significant difference in the GMs among the seasons (P < 0.01). The GMs in the G2 and G3 seasons ranged from 115 to 161 kg N ha−1 period−1, and was greater than those in the other seasons (). There was no significant difference in the GMs between the G2 and G3 seasons. The GMs in the G1 season ranged from 59 to 67 kg N ha−1 period−1, and the GMs in the NG season ranged from 31 to 37 kg N ha−1 period−1 (). The annual GMs in 2005 and 2006 were 367 and 331 kg N ha−1 year−1 for the fertilizer plot, 369 and 336 kg N ha−1 year−1 for the manure plot, 382 and 348 kg N ha−1 year−1 for the control plot and 384 and 356 kg N ha−1 year−1 for the bare plot, respectively (). The annual GMs value was greater in 2005 than in 2006, and was greater in the bare plot than in the fertilizer plot (P < 0.01).
Table 4 N deposition, gross N mineralization of soil (GMs), root-litter (GMl) and manure (GMm), and mineral N pool during each growing season (G1, G2 and G3) and non-growing season (NG) (kg N ha−1 period−1)
The GMl in the G1 season ranged from 68 to 104 kg N ha−1 period−1, and was greater than that in other seasons (P < 0.01) (). In the G1 season, the GMl for each plot was greater than the GMs (). The annual GMl values in 2005 and 2006 were 145 and 126 kg N ha−1 year−1 for the fertilizer plot, 165 and 122 kg N ha−1 year−1 for the manure plot, and 108 and 94 kg N ha−1 year−1 for the control plot, respectively ().
The GMm value was greater in the G2 and G3 seasons than in the NG season (). The annual GMm values in 2005 and 2006 were 88 and 96 kg N ha−1 year−1, respectively ().
N uptake in aboveground biomass
shows the N uptake in aboveground biomass. There was a significant difference among the seasons (P < 0.01), and the N uptake in aboveground biomass in the G1 season was the greatest, followed by those in the G2, G3 and NG seasons. The negative values for the NG season, which were a result of the decrease in aboveground biomass, suggested that the mineral N could have been supplied to soil by the dead aboveground biomass through the decomposition process. The annual values of N uptake in aboveground biomass in 2005 and 2006 were 249 and 219 kg N ha−1 year−1 for the fertilizer plot, 215 and 201 kg N ha−1 year−1 for the manure plot, and 109 and 134 kg N ha−1 year−1 for the control plot (). The annual N uptake in aboveground biomass was greater in the fertilizer and manure plots than in the control plot (P < 0.01), and there were no significant differences among the years.
Table 5 N uptake in aboveground biomass during each growing season (G1, G2 and G3) and non-growing season (NG) (kg N ha−1 period−1)
The ANR in 2005 and 2006 was 85 and 46% for the fertilizer plot, and 29 and 15% for the manure plot, respectively.
N2O flux
Nitrous oxide fluxes in the fertilizer and manure plots increased after the application of fertilizer or manure. These remained at a higher level than in the control plot until the beginning of September (). The N2O flux in the fertilizer plot increased after fertilization in May and July to 37 and 314 μg N m−2 h−1 in 2005, and to 152 and 211 μg N m−2 h−1 in 2006 (). In the manure plot, however, it reached a high peak after the application of manure and fertilizer in May (276 and 1290 μg N m−2 h−1 in 2005 and 2006, respectively) (). The N2O flux in the control plot gradually increased with an increase in soil temperature, and reached the maximum values of 50 μg N m−2 h−1 in August 2005 and 66 μg N m−2 h−1 in July 2006. The N2O flux in the bare plot also increased with an increase in soil temperature, but the maximum value (347 μg N m−2 h−1 in August 2005 and 403 μg N m−2 h−1 in July 2006) was higher than that in the control plot. The N2O fluxes in the fertilizer, manure and bare plots were significantly higher than that in the control plot (P < 0.01), but there were no significant differences among the fertilizer, manure and bare plots.
The relationship between the N2O fluxes and N2O-N/NO-N ratio is shown in . The N2O flux increased with an increase in the N2O-N/NO-N ratio. CitationBouwman (1990) summarized the results reported by CitationAnderson and Levine (1986) and CitationLipschultz et al. (1981), and concluded that the N2O-N/NO-N ratio was <1 during nitrification and >100 during denitrification. Therefore, N2O could have been produced mainly by denitrification in our study site.
The cumulative N2O flux for all seasons is shown in . The cumulative N2O flux was higher in the G2 season than all other seasons except in the manure plot in 2006, but there was a significant difference only between the G2 and NG seasons. In each season, the cumulative N2O flux was compared with the cumulative CO2 flux (), which was reported by CitationShimizu et al. (2009). The cumulative N2O flux in each plot had a positive correlation with the cumulative CO2 flux (Fertilizer plot; Cumulative N2O flux = 0.36 × cumulative CO2 flux – 0.23; R 2 = 0.48; P < 0.05, Manure plot; Cumulative N2O flux = 0.38 × cumulative CO2 flux – 0.17; R 2 = 0.33; P = 0.08, Control plot; Cumulative N2O flux = 0.08 × cumulative CO2 flux – 0.05; R 2 = 0.76; P < 0.01, Bare plot; Cumulative N2O flux = 1.30 × cumulative CO2 flux – 0.39; R 2 = 0.72; P < 0.01) ().
The annual N2O fluxes in 2005 and 2006 were 2.8 ± 0.7 and 3.0 ± 0.8 kg N ha−1 year−1 for the fertilizer plot, 3.6 ± 1.3 and 4.9 ± 3.0 kg N ha−1 year−1 for the manure plot, 0.7 ± 0.5 and 0.6 ± 0.3 kg N ha−1 year−1 for the control plot, and 4.2 ± 3.3 and 3.3 ± 2.1 kg N ha−1 year−1 for the bare plot (). There was no significant difference in the annual N2O flux among the years, and the annual N2O flux was significantly higher in the fertilizer, manure and bare plots than in the control plot (Fertilizer plot: P < 0.05, Manure plot: P < 0.01).
Table 6 The cumulative N2O flux during each growing season (G1, G2 and G3) and non-growing season (NG) (kg N ha−1 period−1)
Figure 4 The cumulative N2O flux for each seasons (G1, G2, G3 and non-growing season [NG]) compared with the cumulative CO2 flux. G1 is the period from the beginning of the growing season to the first crop harvest, G2 is the period from the first harvest to the second harvest and G3 is the period from the second harvest to the end of the growing season. Data of the cumulative N2O flux represent means ± (uncertainties/100 × means). The cumulative CO2 flux was referred to Shimizu et al. (2009).
![Figure 4 The cumulative N2O flux for each seasons (G1, G2, G3 and non-growing season [NG]) compared with the cumulative CO2 flux. G1 is the period from the beginning of the growing season to the first crop harvest, G2 is the period from the first harvest to the second harvest and G3 is the period from the second harvest to the end of the growing season. Data of the cumulative N2O flux represent means ± (uncertainties/100 × means). The cumulative CO2 flux was referred to Shimizu et al. (2009).](/cms/asset/4d4e5770-1a37-4f88-9690-d92db9f68763/tssp_a_10382839_o_f0004g.gif)
Discussion
Relationship between mineral N supply and N uptake in aboveground biomass
The sources of mineral N supply for plant growth are considered to be N deposition, chemical and manure fertilizer application, biological N2 fixation, mineralization from soil organic matter (GMs), root-litter (GMl) and manure (GMm). Among these components, N deposition, biological N2 fixation, chemical fertilizer application and GMm are all external mineral N supply. In the present study, the biological N2 fixation was assumed to be negligible for the estimation of total and external mineral N supplies, because of a low percentage of legumes (). The plots of the N uptake in aboveground biomass against the external mineral N supply during each growing season showed that the N uptake in aboveground biomass was equivalent to or greater than the external mineral N supply except for the manure plot in the G2 season (). On the other hand, the plots of the aboveground N uptake against total mineral N supply showed that the total mineral N supply exceeded the N uptake in aboveground biomass, and there was a significant linear correlation between them (
Figure 5 N uptake in aboveground biomass during each growing season (G1, G2, G3) compared with the external mineral N supply (a) and total mineral N supply (b). The external N supply is composed of N deposition, chemical fertilizer application and the gross N mineralization of applied manure. The total mineral N supply is composed of the external N supply, gross N mineralization of soil and gross mineralization of root-litter. G1 is the period from the beginning of the growing season to the first crop harvest, G2 is the period from the first harvest to the second harvest and G3 is the period from the second harvest to the end of the growing season. The data of N uptake in aboveground biomass represent means ± (uncertainties/100 × means). The dashed-line indicates a regression model for all values, N uptake in aboveground biomass = 0.58 × total mineral N supply – 47; R2 = 0.68; P < 0.01.
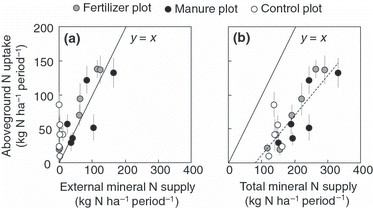
The annual GMs was 2.2–3.6 times larger than the annual GMl. However, the GMl in the G1 season, which accounted for 61–83% of the annual GMl, was 1.1–1.7 times larger than the GMs in the season. This indicates that decomposition of fine roots plays an important role in contributing to the mineral N supply, especially for the first crop. Some reports suggest the significance of fine roots as a source of belowground litter because of their abundance and rapid turnover (CitationGholz et al. 1986; CitationGill and Jackson 2000; CitationGill et al. 2002). CitationSteinaker and Wilson (2005) reported that fine roots accounted for about 90% of the total litter production in natural grassland in Saskatchewan, Canada. CitationParton et al. (2007) and CitationSeastedt et al. (1992) showed that mineral N was proportionally released with decomposition of root litters. CitationFitzhugh et al. (2001) suggested that an increase in the mortality of fine roots due to soil freezing in boreal forests was likely to be an important source of mobile N, based on the observations using mini-rhizotron. They reported that the mortality of fine roots was concentrated in the winter months as concrete frost permeated the rooting zone (CitationGroffman et al. 2001; CitationRuess et al. 1998; CitationTierney et al. 2001). This was the same for our study site too, as the soil is frozen up to about 20 cm in the winter (CitationShimizu et al. 2009), and the mortality of fine roots in the winter could be a main source of belowground litter.
In the present study, GM was estimated by dividing heterotrophic respiration by a C : N ratio (Eq. Equation3) and it was important specifically as the source of mineral N supply. GMl was a major source of mineral N supply especially in the spring. In the previous report (CitationShimizu et al. 2009), although the source of the increment of CO2 flux in the spring was argued as not only root-litter decomposition but also root respiration, the results of this study revealed that root-litter decomposition could possibly be the main source of an increment of CO2 fluxes in the spring.
Factors controlling N2O emission
A significant positive correlation between the cumulative N2O and CO2 fluxes in the bare plot () indicates that the soil organic matter decomposition contributed to the N2O flux. This relationship was found also in the three other plots of fertilizer, manure and control. The slope of the regression equation for cumulative N2O and CO2 fluxes was largest in the bare plot, because CO2 fluxes in the other plots include root respiration. However, the slope was higher in the fertilizer and manure plots than in the control plot due to an increase in N2O fluxes with the application of chemical fertilizer and manure (CitationBouwman 1996).
shows the relationship between the cumulative N2O flux and the total mineral N supply for each season, which was estimated as the sum of N deposition, the chemical fertilizer application, GMm, GMs and GMl. There was a significant positive correlation between the cumulative N2O flux and the total mineral N supply (Cumulative N2O flux = 0.0059 × (total mineral N supply) − 0.103; R 2 = 0.37; P < 0.01). However, the rate of the cumulative N2O flux to the total mineral N supply was higher in the bare plot than in the other three plots including plant growth (). This is because of the absence of plant N uptake in the bare plot while the N uptake in aboveground biomass accounted for 58% of the total mineral N supply in the other three plots (, Eq. Equation6). When the cumulative N2O flux for each season was plotted against the mineral N surplus, which was estimated as the difference between the total mineral N supply and the aboveground N uptake (), a much better significant positive correlation was found (Cumulative N2O flux = 0.0120 × (mineral N surplus) − 0.537; R 2 = 0.05; P < 0.01). The slope of the regression equation showed that the N2O emission factor against the mineral N surplus was 1.20%, and a negative value of the y-interception showed immobilization of mineral N (CitationSchimel et al. 1985). CitationKaiser and Ruser (2000) reported that the annual N2O emission measured in the arable land soils in Germany had an even stronger correlation with the N balance estimated as the difference between the N fertilization and the crop N uptake than with N fertilization. CitationKatayanagi et al. (2008) also found that N2O emissions conducted on the same field in this study including cornfields had a positive correlation with the N surplus, which was estimated as the difference of all total input (application of fertilizer, manure and slurry, and livestock excreta during grazing) and total output (yield and grazed grass). However, those reports did not take into consideration the contribution of N mineralization to N2O emission. CitationKaiser and Ruser (2000) suggested the contribution of gross N mineralization to N2O emissions from the result, that unfertilized soil produced an N yield of 60 kg N ha−1 year−1 and an N2O emission of 2 kg N ha−1 year−1. In this study, the sum of GMs and GMl in the fertilizer plot in 2005 and 2006 were 512 and 457 kg N ha−1 year−1, respectively. These values are significantly larger than the application rate of chemical fertilizer (164 and 183 kg N ha−1 year−1). CitationMu et al. (2008) reported that the sum of chemical N fertilizer and GMs correlated with the cumulative N2O fluxes better than only the chemical N fertilizer. Thus, not only amounts of N input and crop N uptake, but also the amount of gross N mineralization is indispensable in predicting the amount of N2O emission.
Figure 6 The cumulative N2O flux for each season (G1, G2, G3 and non-growing season [NG]) compared with the total mineral N supply (a) and the mineral N surplus (b). The total mineral N supply is composed of the external N supply (N deposition, chemical fertilizer application and gross N mineralization of applied manure), gross N mineralization of soil and gross mineralization of root-litter. The mineral N surplus was estimated as the difference between the mineral N pool and N uptake in aboveground biomass. G1 is the period from the beginning of the growing season to the first crop harvest, G2 is the period from the first harvest to the second harvest and G3 is the period from the second harvest to the end of the growing season. The data of cumulative N2O flux represent means ± (uncertainties/100 × means). The regression model shown in Fig. 6a is for all values, Cumulative N2O flux = 0.0059 × (total mineral N supply) – 0.103; R2 = 0.37; P < 0.01. The regression model shown in Figure 6b is for all values, Cumulative N2O flux = 0.0120 × (mineral N surplus) – 0.537; R2 = 0.50; P < 0.01.
![Figure 6 The cumulative N2O flux for each season (G1, G2, G3 and non-growing season [NG]) compared with the total mineral N supply (a) and the mineral N surplus (b). The total mineral N supply is composed of the external N supply (N deposition, chemical fertilizer application and gross N mineralization of applied manure), gross N mineralization of soil and gross mineralization of root-litter. The mineral N surplus was estimated as the difference between the mineral N pool and N uptake in aboveground biomass. G1 is the period from the beginning of the growing season to the first crop harvest, G2 is the period from the first harvest to the second harvest and G3 is the period from the second harvest to the end of the growing season. The data of cumulative N2O flux represent means ± (uncertainties/100 × means). The regression model shown in Fig. 6a is for all values, Cumulative N2O flux = 0.0059 × (total mineral N supply) – 0.103; R2 = 0.37; P < 0.01. The regression model shown in Figure 6b is for all values, Cumulative N2O flux = 0.0120 × (mineral N surplus) – 0.537; R2 = 0.50; P < 0.01.](/cms/asset/d7e91a0c-f721-4f81-a89f-5d1bd3665f87/tssp_a_10382839_o_f0006g.gif)
Despite the significant positive relationship between the cumulative N2O flux and mineral N surplus, there was variability in the relationship (). Therefore, we conducted multiple regression analysis to explain the variability. We selected the N2O emission factor against the mineral N surplus for each season as an objective variable, and mean precipitation, mean daily air temperature and mineral surplus N as explanatory variables. The results showed that the emission factor against mineral N surplus was significantly correlated with the mean precipitation (Cumulative N2O/mineral N surplus × 100 = 0.195 × mean precipitation – 0.055; R 2 = 0.21; P < 0.01) (). This suggests that an N2O emission from surplus mineral N increases with an increase in precipitation. In the present study, the predominant source of N2O appeared to be denitrification (), and high precipitation should have enhanced denitrification. CitationMori et al. (2008) also reported that high precipitation enhanced N2O production from denitrification. These results indicated that an optimum N input aiming at reducing the N surplus is required to minimize the environmental risk associated with N2O emission.
Conclusion
Together with chemical fertilizer and manure, mineralization from soil organic matter and root-litter were the major sources for plant growth and N2O emission in the managed grassland. Mineral N surplus, which was estimated as the difference between the total mineral N supply and N uptake in aboveground biomass, was a better indicator of N2O emission than the total mineral N supply. The N2O emission factor against the mineral N surplus was estimated to be 1.20%, and the emission factor in each season increased with an increase in precipitation. These results also indicated that the reduction in mineral N surplus would be able to mitigate N2O emission from the grassland.
Figure 7 The N2O emission factor to mineral N surplus for each season (G1, G2, G3 and non-growing season [NG]) compared with mean precipitation. The mineral N surplus was estimated as the difference between the mineral N pool and N uptake in aboveground biomass. G1 is the period from the beginning of the growing season to the first crop harvest, G2 is the period from the first harvest to the second harvest and G3 is the period from the second harvest to the end of the growing season. The data of N2O emission factor to mineral N surplus represent means ± (uncertainties/100 × means). The regression line is for all values, Cumulative N2O/mineral N surplus × 100 = 0.195 × mean precipitation – 0.055; R2 = 0.21; P < 0.01.
![Figure 7 The N2O emission factor to mineral N surplus for each season (G1, G2, G3 and non-growing season [NG]) compared with mean precipitation. The mineral N surplus was estimated as the difference between the mineral N pool and N uptake in aboveground biomass. G1 is the period from the beginning of the growing season to the first crop harvest, G2 is the period from the first harvest to the second harvest and G3 is the period from the second harvest to the end of the growing season. The data of N2O emission factor to mineral N surplus represent means ± (uncertainties/100 × means). The regression line is for all values, Cumulative N2O/mineral N surplus × 100 = 0.195 × mean precipitation – 0.055; R2 = 0.21; P < 0.01.](/cms/asset/4d8b3a9d-bf9f-4698-86a0-3d30116793c8/tssp_a_10382839_o_f0007g.gif)
Acknowledgments
This study was partly supported by a research grant provided by the Project entitled ‘Establishment of good practices to mitigate Greenhouse Gas emissions from Japanese grasslands’ funded by Racing and Livestock Association. We thank the technical staff of Shizunai Livestock Farm for their help in managing the grassland.
References
- Adams , JM , Faure , H , Fauredenard , L , Mcglade , JM and Woodward , FI . 1990 . Increases in terrestrial carbon storage from the last glacial maximum to the present . Nature , 348 : 711 – 714 .
- Anderson , IC and Levine , JS . 1986 . Relative rates of nitric-oxide and nitrous-oxide production by nitrifiers, denitrifiers, and nitrate respirers . Appl. Environ. Microbiol. , 51 : 938 – 945 .
- Bouwman , AF . 1990 . “ Exchange of greenhouse gases between terrestrial ecosystems and the atmosphere ” . In Soils and the Greenhouse Effect , Edited by: Bouwman , AF . 61 – 127 . Chichester, , UK : John Wiley and Sons .
- Bouwman , AF . 1996 . Direct emission of nitrous oxide from agricultural soils . Nutr. Cycl. Agroecosyst. , 46 : 53 – 70 .
- Bouwman , AF , Boumans , LJM and Batjes , NH . 2002 . Modeling global annual N2O and NO emissions from fertilized fields . Global Biogeochem. Cycles , 16 : 1080
- Chu , HY , Hosen , Y and Yagi , K . 2007 . NO, N2O, CH4and fluxes in winter barley field of Japanese Andisol as affected by N fertilizer management . Soil Biol. Biochem. , 39 : 330 – 339 .
- Crutzen , PJ . 1974 . Estimates of possible variations in total ozone due to natural causes and human activities . Ambio , 3 : 201 – 210 .
- Dam Kofoed , A and Brogan , JG . 1981 . “ Crop uptake of nitrogen ” . In Nitrogen Losses and Surface Run-off from Landspreading of Manures , 125 – 160 . The Hague : Nijhoff & Junk .
- Dubeux , JCB , Sollenberger , LE , Mathews , BW , Scholberg , JM and Santos , HQ . 2007 . Nutrient cycling in warm-climate grasslands . Crop Sci. , 47 : 915 – 928 .
- Fitzhugh , RD , Driscoll , CT , Groffman , PM , Tierney , GL , Fahey , TJ and Hardy , JP . 2001 . Effects of soil freezing disturbance on soil solution nitrogen, phosphorus, and carbon chemistry in a northern hardwood ecosystem . Biogeochemistry , 56 : 215 – 232 .
- Gholz , HL , Hendry , LC and Cropper , WP . 1986 . Organic-matter dynamics of fine roots in plantations of slash pine (Pinus-elliottii) in north Florida . Can. J. For. Res. , 16 : 529 – 538 .
- Gill , RA , Burke , IC , Lauenroth , WK and Milchunas , DG . 2002 . Longevity and turnover of roots in the shortgrass steppe: influence of diameter and depth . Plant Ecol. , 159 : 241 – 251 .
- Gill , RA and Jackson , RB . 2000 . Global patterns of root turnover for terrestrial ecosystems . New Phytol. , 147 : 13 – 31 .
- Gillett , RW and Ayers , GP . 1991 . The use of thymol as a biocide in rainwater samples . Atmos. Environ. , 25A : 2677 – 2681 .
- Groffman , PM , Driscoll , CT , Fahey , TJ , Hardy , JP , Fitzhugh , RD and Tierney , GL . 2001 . Colder soils in a warmer world: a snow manipulation study in a northern hardwood forest ecosystem . Biogeochemistry , 56 : 135 – 150 .
- Hashidoko , Y , Takakai , F Toma , Y . 2007 . Emergence and behaviors of acid-tolerant Janthinobacteriumsp. that evolves N2O from deforested tropical peatland . Soil Biol. Biochem. , 34 : 116 – 125 .
- Ohara , M , Takeda , Y and Omura , K , eds. 2004 . “ Hokkaido Prefectural Experiment Stations and Hokkaido Animal Research Center ” . In Handbook of animal waste management and utilization in Hokkaido 2004 , 64 – 67 . Sapporo (in Japanese) : Hokkaido Prefectural Experiment Stations and Hokkaido Animal Research Center .
- IPCC . 2007 . Climate changes 2007: The Physical Science Basis , Cambridge : Cambridge University Press .
- IUSS Working Group WRB . 2006 . World Reference Base for Soil Resources 2006 , Rome : World Soil Resources Reports 103, FAO .
- Jordan , TE , Correll , DL and Weller , DE . 1997 . Effects of agriculture on discharges of nutrients from coastal plain watersheds of Chesapeake Bay . J. Environ. Qual. , 26 : 836 – 848 .
- Kaffka , SR and Kanneganti , VR . 1996 . Orchardgrass response to different types, rates and application patterns of dairy manure . Field Crops Res. , 47 : 43 – 52 .
- Kaiser , EA and Ruser , R . 2000 . Nitrous oxide emissions from arable soils in Germany – An evaluation of six long-term field experiments . J. Plant Nutr. Soil Sci. , 163 : 249 – 259 .
- Katayanagi , N , Sawamoto , T , Hayakawa , A and Hatano , R . 2008 . Nitrous oxide and nitric oxide fluxes from cornfield, grassland, pasture and forest in a watershed in Southern Hokkaido, Japan . Soil Sci. Plant Nutr. , 54 : 662 – 680 .
- Linn , DM and Doran , JW . 1984 . Effect of water-filled pore-space on carbon-dioxide and nitrous-oxide production in tilled and nontilled soils . Soil Sci. Soc. Am. J. , 48 : 1267 – 1272 .
- Lipschultz , F , Zafiriou , OC , Wofsy , SC , Mcelroy , MB , Valois , FW and Watson , SW . 1981 . Production of NO and N2O by soil nitrifying bacteria . Nature , 294 : 641 – 643 .
- Luxhoi , J , Bruun , S , Stenberg , B , Breland , TA and Jensen , LS . 2006 . Prediction of gross and net nitrogen mineralization-immobilization turnover from respiration . Soil Sci. Soc. Am. J. , 70 : 1121 – 1128 .
- Maag , M and Vinther , FP . 1996 . Nitrous oxide emission by nitrification and denitrification in different soil types and at different soil moisture contents and temperatures . Appl. Soil Ecol. , 4 : 5 – 14 .
- Mori , A , Hojito , M , Shimizu , M , Matsuura , S , Miyaji , T and Hatano , R . 2008 . N2O and CH4fluxes from a volcanic grassland soil in Nasu, Japan: comparison between manure plus fertilizer plot and fertilizer-only plot . Soil Sci. Plant Nutr. , 54 : 606 – 617 .
- Morishita , T , Hatano , R , Nagata , O , Sakai , K , Koide , T and Nakahara , O . 2004 . Effect of nitrogen deposition on CH4uptake in forest soils in Hokkaido, Japan . Soil Sci. Plant Nutr. , 50 : 1187 – 1194 .
- Mosier , AR . 1998 . Soil processes and global change . Biol. Fertil. Soils , 27 : 221 – 229 .
- Mu , Z , Kimura , SD , Toma , Y and Hatano , R . 2008 . Nitrous oxide fluxes from upland soils in central Hokkaido, Japan . J. Environ. Sci. , 20 : 1312 – 1322 .
- Parton , W , Silver , WL Burke , IC . 2007 . Global-scale similarities in nitrogen release patterns during long-term decomposition . Science , 315 : 361 – 364 .
- Rahn , T and Wahlen , M . 2000 . A reassessment of the global isotopic budget of atmospheric nitrous oxide . Global Biogeochem. Cycles , 14 : 537 – 543 .
- Rockmann , T , Kaiser , J and Brenninkmeijer , CAM . 2003 . The isotopic fingerprint of the pre-industrial and the anthropogenic N2O source . Atmos. Chem. Phys. , 3 : 315 – 323 .
- Ruess , RW , Hendrick , RL and Bryant , JP . 1998 . Regulation of fine root dynamics by mammalian browsers in early successional Alaskan taiga forests . Ecology , 79 : 2706 – 2720 .
- Schimel , DS . 1986 . Carbon and nitrogen turnover in adjacent grassland and cropland ecosystems . Biogeochemistry , 2 : 345 – 357 .
- Schimel , DS , Coleman , DC and Horton , KA . 1985 . Soil organic-matter dynamics in paired rangeland and cropland toposequences in North Dakota . Geoderma , 36 : 201 – 214 .
- Seastedt , TR , Parton , WJ and Ojima , DS . 1992 . Mass-loss and nitrogen dynamics of decaying litter of grasslands – the apparent low nitrogen immobilization potential of root detritus . Can. J. Bot. , 70 : 384 – 391 .
- Shiga , H , Ohyama , N , Maeda , K and Suzuki , M . 1985 . An evaluation of different organic materials based on their decomposition pattern in paddy soils . Res. Bull. Natl. Agric. Res. Cetr. , 5 : 1 – 19 . (In Japanese with English summary.)
- Shimizu , M , Marutani , S , Desyatkin , AR , Jin , T , Hata , H and Hatano , R . 2009 . The effect of manure application on carbon dynamics and budgets in a managed grassland of Southern Hokkaido, Japan . Agric. Ecosyst. Environ. , 130 : 31 – 40 .
- Soil Survey staff . 2006 . Keys to Soil Taxonomy , 10th edn , Washington, DC : USDA-Natural Resources Conservation Service .
- Soussana , JF , Fuhrer , J , Jones , M and van Amstel , A . 2007 . The greenhouse gas balance of grasslands in Europe . Agric. Ecosyst. Environ. , 121 : 1 – 4 .
- Steinaker , DF and Wilson , SD . 2005 . Belowground litter contributions to nitrogen cycling at a northern grassland-forest boundary . Ecology , 86 : 2825 – 2833 .
- Tierney , GL , Fahey , TJ , Groffman , PM , Hardy , JP , Fitzhugh , RD and Driscoll , CT . 2001 . Soil freezing alters fine root dynamics in a northern hardwood forest . Biogeochemistry , 56 : 175 – 190 .
- Toma , Y and Hatano , R . 2007 . Effect of crop residue C:N rate on N2O emissions from Gray Lowland soil in Mikasa, Hokkaido, Japan . Soil Sci. Plant Nutr. , 53 : 198 – 205 .
- White , R , Murray , S and Rohweder , M . 2000 . Pilot Analysis of Global Ecosystems: Grassland Ecosystems , Washington, DC : World Resources Institute .
- Yamulki , S , Harrison , RM , Goulding , KWT and Webster , CP . 1997 . N2O, NO and NO2fluxes from a grassland: effect of soil pH . Soil Biol. Biochem. , 29 : 1199 – 1208 .