Abstract
The development of nanoparticle platforms with long in vivo circulation half-life has long been one of the major goals in the field of cancer drug delivery. Long-circulating nanoparticles can more effectively localize to the tumor site through either passive or active targeting mechanisms. The current gold standard for bestowing long-circulating attributes involves the use of PEG, which surrounds the particles with a hydration layer and thereby prevents recognition by the mononuclear phagocyte system. Recently, a new strategy for synthesizing biomimetic nanoparticles has been inspired by the body's own long-circulating entities, red blood cells (RBCs). Such a system disguises drug nanocarriers as ‘self’ using membrane materials directly derived from RBCs. This method has been demonstrated to prolong particle systemic circulation half-life beyond that of the corresponding PEGylated systems. The RBC membrane-coated nanoparticles present a major breakthrough in drug delivery technology and show great promise for clinical applications. Herein we highlight the significance and the unique features of this nature-inspired nanoparticle platform and offer opinions on its future prospects.
1. Introduction
The advent of nanoparticle-based drug delivery systems has driven many exciting developments in the field of disease therapeutics Citation[1]. For cancer therapy, nanoparticulate drug formulations hold several advantages over their free-drug counterparts, including improved pharmacokinetics, enhanced tumor accumulations, reduced systemic exposure and side effects, and better patient compliance Citation[2]. An important feature that underlies these advantages is a long systemic circulation half-life, which requires the nanoparticles to evade capture by the macrophages of the mononuclear phagocyte system (MPS). The advent of ‘stealth’ nanoparticles has had a great effect in nanoparticle drug delivery, particularly toward cancer treatment. Many studies have shown that stealthy, long-circulating nanoparticles can better localize to their tumoral targets in vivo by taking advantage of the tumor's leaky vasculature, a phenomenon known as the enhanced permeation and retention (EPR) effect. Moreover, stealthy nanoparticles can selectively target cancer cells through functionalization with tumor-specific targeting ligands.
With the recent advancements in nanotechnology, numerous strategies have been implemented to enable nanoparticle immune evasion. Currently, the most widely adopted immune evasion strategy is PEG functionalization. These hydrophilic polymers hide the nanoparticles behind a hydration layer and reduce their immune clearance Citation[3]. Normally, when foreign entities are circulating within the bloodstream, they are marked for uptake by the MPS through a process known as opsonization. Particles functionalized with PEG or other hydrophilic polymers are shielded with water molecules and appear invisible to opsonins and macrophages. The use of PEG to increase circulation half-life has proven to be an effective one, bestowing upon nanoparticles' half-life on the order of tens of hours as opposed to minutes for non-PEGylated nanoparticles Citation[4]. Despite PEG's successes and its widespread adoption, recent observations of anti-PEG immunological response Citation[5] have triggered the interests of scientists and engineers to investigate long-circulation strategies with inspirations from biology. As the immune system catches up in the game of hide-and-seek, novel biomimetic strategies have been proposed to camouflage drug carriers as self, endogenous entities in order to bypass immune surveillance.
2. Red blood cell (RBC)-based systems as long-circulating drug carriers
Several groups have taken inspiration from nature to develop long-circulating drug carriers Citation[6]. To this end, RBCs, natural carriers of oxygen that normally can circulate in the bloodstream for up to 120 days, represent an ideal system to mimic and exploit. For instance the highly flexible structure of RBCs enables them to pass through narrow capillary networks and ‘sieving organs’ such as the spleen and the liver. This attribute has inspired highly biconcave microparticles as developed by Doshi et al. Citation[7] and Merkel et al. Citation[8], who showed that mechanobiological mimicry of RBCs can increase the particle elasticity and extend their circulation times. Recent advances in molecular and cellular biology also focused on the immune-evasive functionalities of the membrane proteins on RBC surfaces. It has been found that the recognition of homologous RBCs as self is mediated by a variety of proteins residing on the membrane of the cells. For example, CD47 has been identified on RBC surfaces as a self-marker that actively signals macrophages and prevents their uptake Citation[9]. In addition, other membrane proteins including C8-binding protein (C8bp) Citation[10], homologous restriction protein (HRP) Citation[11], decay-accelerating factor (DAF), membrane cofactor protein (MCP), complement receptor 1 (CR1), and CD59 Citation[12] on RBC surfaces fend off the attack by the complement system. A drug delivery system possessing these surface properties of RBCs should therefore be able to, at least in theory, minimize immune response by posing as self and thereby achieve long systemic circulation.
Synthetic approaches to mimic the RBC surface properties have been reported. Tsai et al., for instance, have conjugated CD47 to polystyrene beads and demonstrated promising outcomes in inhibiting macrophage uptake Citation[13]. However, existing nanoparticle functionalization techniques through chemical conjugations have difficulty in replicating the complex protein chemistry of the RBC membranes. Alternative approaches have explored the use of RBCs and their derivatives for the transport of therapeutic compounds. In concept, if drug carriers were to be prepared from autologous RBCs, they would be fully biocompatible with little immunogenicity. Such applications of using RBCs as drug carriers have been reported and shown great promise in terms of their drug loading and circulation half-life properties Citation[6]. In addition, nanoscale RBC membrane-derived vesicles have also been developed by physically extruding RBCs. In doing so, it was possible to make 100 nm drug carriers that were essentially miniaturized RBCs. Daunorubicin was conjugated to these vesicles and the resulting product was shown to have good cytotoxicity in vitro as compared with free daunorubicin. However, later study revealed that these carriers were cleared rapidly from the bloodstream, lasting approximately 30 min in circulation Citation[14]. Such rapid clearance was probably caused by the conjugation of drug molecules that covered the membrane surface. In addition, vesicle aggregation was another issue that plagued the system as large membrane aggregates were found to accumulate in the lung. This finding indicates that these miniaturized RBC vesicles lacked structural integrity and were highly unstable. While intriguing, these RBC membrane-derived vesicles left much room for improvement. A different scheme for drug loading and better structural support would benefit this nanoscale RBC-derived drug carrier concept.
3. RBC membrane-coated polymeric nanoparticles
Recently, Hu et al. have developed a new drug delivery platform that pairs nanoscale RBC membrane-derived vesicles with polymeric nanoparticles made from poly(lactic-co-glycolic acid) (PLGA), an FDA-approved polymer Citation[15]. The resulting nanoparticle has a core–shell structure that consists of a PLGA core surrounded by an RBC membrane-formed shell. In combining the advantages of RBC-based carriers with that of polymeric nanoparticles, this technology has the potential to enable the delivery of slow-releasing drug payloads in vivo with a carrier circulation half-life beyond what can be achieved by PEG. The particles are synthesized in a few simple steps. First, the cores are made using a nanoprecipitation method in which PLGA in organic solvent is added carefully into a miscible anti-solvent. RBC membrane-derived vesicles are then created by extruding 2 μm wide RBC ghosts through a membrane with 100 nm pores. The resulting polymeric cores and RBC vesicles are then extruded together in order to mechanically induce the adsorption and fusion of RBC membranes onto the nanoparticle surfaces. It has been demonstrated that the resulting RBC membrane-coated nanoparticles had excellent stability in phosphate buffered solution (PBS) and in fetal bovine serum (FBS). Additionally, the protein content of the final camouflaged particles was nearly identical to that of the RBC ghosts, indicating that all of the membrane self-markers responsible for long circulation are retained through the preparation process. Upon uptake by a cancer cell in vitro, it was shown that the particle core and the membrane shell were co-localized, an important result that further confirmed the integrity of the particles' core–shell structure. Finally, perhaps the most exciting result was obtained when examining the circulation half-life of these camouflaged nanoparticles. As compared with PEG-coated nanoparticles, which had an elimination half-life of 15.8 h in a mouse model, these novel RBC membrane-coated nanoparticles had an elimination half-life of 39.6 h. These findings indicate that the inclusion of a nanoparticle core into RBC membranes seems to have a stabilizing effect on the RBC membrane-derived vesicles, preventing the issues observed with previous nanoscale RBC-derived carriers that severely affected their circulation lifetime.
4. Conclusion
One of the major design considerations for nanoparticle-based drug delivery is prolonging the circulation half-life, which can lead to more effective targeting and improved therapeutic efficacies. The current strategy of using synthetic PEG as a stealth moiety has been effective, but increased understanding in biology has encouraged scientists and engineers to explore natural alternatives. RBCs serve as an ideal natural system to exploit, as they possess the molecular tool-kit for immune-evasion and long circulation. Much work has been done to create RBC-derived drug carriers, and, most recently, a new RBC membrane-coated polymeric nanoparticle system has been developed and demonstrated with excellent stability in vitro and prolonged circulation time in vivo. This new platform represents a major breakthrough in nanotechnology and may represent a significant step forward in the field of nanoparticle drug delivery.
5. Expert opinion
The use of RBC membranes to coat polymeric nanoparticles as a means for disguising the particles in vivo represents a promising new technology that has great clinical potential. In order to realize the potential, further studies and developments need to take place. Scalability is one of the key areas that need to be addressed in order to translate any drug delivery system to clinical applications. For the RBC membrane-coated nanoparticle system, the ease of synthesis at the lab-scale is a good indicator of future scalability. Since both the nanoprecipitation techniques to make polymeric nanoparticles and the extrusion techniques to make liposomal vesicles have matured over recent years, the RBC membrane-coated nanoparticles can be manufactured on a large scale by streamlining the production of polymeric cores and RBC membrane-derived vesicles in parallel.
Another exciting feature of the RBC membrane-coated nanoparticle platform is that its core and surface can be tailored individually, offering a high degree of synthetic freedom. In the polymeric core, the platform can encapsulate a wide array of therapeutic payloads and/or imaging agents. On the membrane surface, the lipid bilayer structure allows for the incorporation of different functional groups through the use of phospholipid anchors. Doing so will enable active targeting of the nanoparticles to specific targets in vivo. While the current design uses a polymer as the core material, it can also be envisioned that this RBC membrane coating technique can be generalized to many other types of nanostructures, such as quantum dots and metal oxide nanoparticles. By generalizing this platform, it may one day be possible to develop a wide array of long-circulating nanoparticles spanning numerous modalities, both diagnostic and therapeutic.
Looking to the future, the use of RBC membrane-coated nanoparticles could open up an exciting new field in personalized medicine. As illustrated in , blood can be drawn from a patient and the derived RBC vesicles will then be used to coat therapeutic or diagnostic nanoparticulate cores before injecting them back into the same patient. Containing on average 5 billion RBCs in 1 ml volume, human blood provides an abundance of coating materials for the functionalization of drug carriers. This use of autologous cells would maximize immune tolerance to the encapsulated agent in use. Alternatively, donor blood could also be used in a similar fashion as in blood transfusion; RBCs can be obtained from the blood bank through blood-matching and O-type blood could be used to derive universal coating materials. While there are a number of exciting opportunities going forward, additional studies are warranted and further optimization can be done on the system. The future progression of this technology promises highly efficient drug delivery applicable to many disease types.
Figure 1. Illustration of personalized therapy using the red blood cell (RBC) membrane-coated nanoparticle platform. Blood is drawn from a patient and processed in order to derive nanoscale vesicles from RBC membranes. These vesicles are then used to coat drug-loaded nanoparticles. Finally, the treatment formulation is injected back into the same patient.
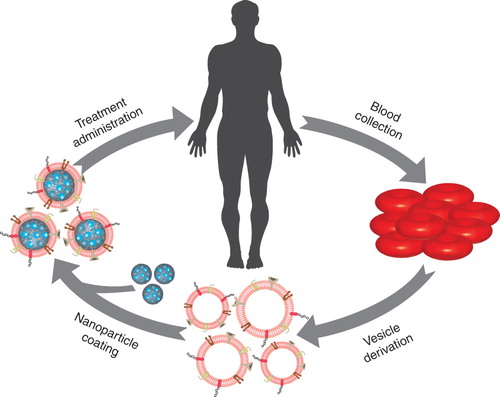
Article highlights.
One of the major design considerations for nanoparticulate drug delivery systems is circulation half-life. Designing long-circulating nanoparticles by taking design cues from natural entities such as red blood cells (RBCs) is a strategy that has been explored for several decades.
Whereas the PEG functionalization strategy is analogous to hiding nanoparticles behind a hydration layer, RBC-inspired strategies are analogous to posing the nanoparticles as ‘self’.
The RBC membrane-coated polymeric nanoparticles represent a new class of long-circulating nanocarriers that are camouflaged by RBC membrane and can be used to deliver drug payloads with the polymeric core.
While still in its infancy, this new RBC membrane-coated nanoparticle technology has significant clinical potential. It is primed for large-scale fabrication and is highly versatile in terms of further functionalization. Different RBC membrane camouflaged nanostructures can be envisioned.
Declaration of interest
This work is supported by the National Science Foundation Grant CMMI-1031239. RH Fang is supported by the Department of Defense (DoD) through the National Defense Science & Engineering Graduate Fellowship (NDSEG) Program.
Notes
This box summarizes key points contained in the article.
Bibliography
- Farokhzad OC, Langer R. Nanomedicine: developing smarter therapeutic and diagnostic modalities. Adv Drug Deliv Rev 2006;58:1456-9
- Zhang L, Gu FX, Chan JM, Nanoparticles in medicine: therapeutic applications and developments. Clin Pharm Ther 2008;83:761-9
- Veronese FM, Pasut G. PEGylation, successful approach to drug delivery. Drug Discov Today 2005;10:1451-8
- Harris JM, Chess RB. Effect of pegylation on pharmaceuticals. Nat Rev Drug Discov 2003;2:214-21
- Knop K, Hoogenboom R, Fischer D, Schubert US. Poly(ethylene glycol) in drug delivery: pros and cons as well as potential alternatives. Angew Chem Int Ed 2010;49:6288-308
- Muzykantov VR. Drug delivery by red blood cells: vascular carriers designed by mother nature. Expert Opin Drug Deliv 2010;7:403-27
- Doshi N, Zahr AS, Bhaskar S, Red blood cell-mimicking synthetic biomaterial particles. Proc Natl Acad Sci USA 2009;106:21495-9
- Merkel TJ, Jones SW, Herlihy KP, Using mechanobiological mimicry of red blood cells to extend circulation times of hydrogel microparticles. Proc Natl Acad Sci USA 2011;108:586-91
- Oldenborg PA, Zheleznyak A, Fang YF, Role of CD47 as a marker of self on red blood cells. Science 2000;288:2051-4
- Schonermark S, Rauterberg EW, Shin ML, Homologous species restriction in lysis of human-erythrocytes – a membrane-derived protein with C8-binding capacity functions as an inhibitor. J Immun 1986;136:1772-6
- Zalman LS, Wood LM, Mullereberhard HJ. Isolation of a human-erythrocyte membrane-protein capable of inhibiting expression of homologous complement transmembrane channels. Proc Natl Acad Sci USA 1986;83:6975-9
- Kim DD, Miwa T, Kimura Y, Deficiency of decay-accelerating factor and complement receptor 1-related gene/protein y on murine platelets leads to complement-dependent clearance by the macrophage phagocytic receptor CRIg. Blood 2008;112:1109-19
- Tsai RK, Rodriguez PL, Discher DE. Self inhibition of phagocytosis: the affinity of 'marker of self' CD47 for SIRPalpha dictates potency of inhibition but only at low expression levels. Blood Cells Mol Dis 2010;45:67-74
- Desilets J, Lejeune A, Mercer J, Gicquaud C. Nanoerythrosomes, a new derivative of erythrocyte ghost: IV. Fate of reinjected nanoerythrosomes. Anticancer Res 2001;21:1741-7
- Hu CMJ, Zhang L, Aryal S, Erythrocyte membrane-camouflaged polymeric nanoparticles as a biomimetic delivery platform. Proc Natl Acad Sci USA 2011;108:10980-5