Abstract
Out of all the neurodegenerative diseases, cell-based therapy has been most intensively tested in Parkinson's disease (PD) patients. Recently, technical advancements in stem cell research have opened the possibility of using stem cell-induced dopaminergic neurons in the clinical setting. However, many issues are yet to be overcome in order to achieve effective and safe therapy before clinical trials can be possible. Here, we discuss how neuroimaging techniques can be used to accelerate studies of stem cell therapy in PD. Neuroimaging techniques allow us to measure various biological markers repeatedly and quantitatively, which may serve as a powerful tool for optimizing the use of stem cells in preclinical and clinical trials.
1. Introduction
It has been over 30 years since neural cell transplantation was first tried as a potential therapy in Parkinson's disease (PD) using fetal midbrain tissues (for review, see Citation[1]). Although double-blind clinical trials did not show significant efficacy of the fetal tissue transplantation, several open-label clinical trials and recent animal studies have shown promising results. However, the limited availability and ethical considerations relating to the use of fetal tissue pose limitations for practical use. Recent technical advances in stem cell research, such as induced pluripotent stem cells (iPS) and mesenchymal stem cells (MSCs), are expected to solve the issue of limited availability of cell sources and to expand potential therapeutic use of stem cells Citation[1,2]. Therefore, it is essential that protocols for cell cultures and cell differentiation are optimized before clinical trials can proceed, in which the cellular efficacy and safety in the host brain must also be evaluated for a long period after transplantation. Here we discuss the importance of cell optimizations based on post-graft information obtained by monitoring with neuroimaging techniques, such as positron emission tomography (PET) and magnetic resonance imaging (MRI). While a number of neuroimaging techniques can be used to understand the pathophysiology of PD (), we will particularly focus on those which may be useful for evaluating and optimizing cell therapy in PD.
Table 1. Neuroimaging instruments, types of imaging, biomarkers and biological & clinical correlates in PD.
2. Why is neuroimaging useful for evaluating cell therapy?
Neuroimaging is useful for the evaluation of cell therapy for several reasons. First, neuroimaging techniques allow us to non-invasively evaluate and monitor the integrity of grafts by examining features such as dopamine synthesizing capacity, non-dopaminergic neural function, survival, tumorigenicity and immune (or inflammatory) response in the grafted tissue. Second, neuroimaging techniques provide quantitative information, which allows us to correlate pre-graft cellular phenotype with post-graft integrity. In particular, information on the survival of grafted cells, as well as on the host tissue environment, may be needed for optimizing cellular differentiation and grafting methods.
The spatial resolution of PET and MRI images is around the order of a few millimeters, which is too large to clearly visualize cellular events occurring at the micrometer level. When compared with other cell imaging modalities such as microscopy, PET and MRI imaging have lower spatial resolutions (). However, these neuroimaging techniques have incomparable advantages such as non-invasiveness and quantitative ability. By contrast, it is not possible to perform monitoring and live imaging of cells using microscopic imaging, and it is difficult to utilize it for quantitating biological function. Since neurodegenerative diseases often proceed over years or decades, the ability to obtain longitudinal and quantitative measurements is of particular importance to efficiently evaluate novel therapeutics Citation[3].
Table 2. Comparison of neuroimaging modalities.
3. Optimizing stem cell therapy using neuroimaging
3.1 Dopaminergic function
The current trials for cell therapy in PD are based on the premise of cell-based dopamine replacement. Therefore, stem cell research has focused on preparing neural cells that produce dopamine resembling dopaminergic neurons. In particular, in PD, A9-type dopaminergic neurons, located in substantia nigra pars compacta, are preferentially vulnerable as compared with other types of dopaminergic neurons such as A8 (located in ventral tegmental area) and A10 (located in retrorubral field) Citation[4]. Recent studies on fetal tissue transplantation suggest that grafts with abundant A9 dopaminergic neurons promote the restoration of synaptic connections and motor function, as well as suppress the formation of side effects, such as dyskinesia Citation[5]. Therefore, current stem cell research effort is largely focused on inducing well-differentiated dopaminergic neurons from embryonic stem (ES) cells Citation[6], as well as from iPS cells Citation[7] and MSCs Citation[8]. Kriks et al. Citation[6] showed that floor-plate strategy using ES cells induces dopaminergic neurons that express tyrosine hydroxylase (TH), dopamine transporter (DAT) and G-protein-regulated inward-rectifier potassium channel 2 (GIRK2). A similar phenotype was recently found in dopaminergic neurons obtained from bone marrow MSCs () Citation[8]. Furthermore, PET scans using a ligand for DAT, 11C-CFT, showed high binding of DAT ligand in the striatum grafted with the MSC-derived dopaminergic neurons (). DAT is more highly expressed by A9-type DA neurons as compared with other types of DA neurons Citation[9], and expression of DAT in the stem cell-derived cells suggests that DA neurons are fully matured Citation[10]. Kinetic analysis of PET data allows us to evaluate the ‘binding potential (BP)' of these ligands, which indicates the density of available DATs (if we assume that the affinity of DAT is not altered).
Figure 1. Dopamine neurons induced from autologous bone marrow mesenchymal stem cells (MSCs) in parkinsonian monkeys and post-graft PET imaging. A) Bone marrow MSCs in primate animals. B) Neuron-like cells induced from MSCs. Immunocytochemistry showed that these neuron-like cells were positive for markers of neurons, Tuj-1 (C) and MAP-2 (D), catecholamine neurons, TH (E), dopaminergic neurons, DAT (F). G) After being autologously grafted into the striatum of MPTP-treated hemi-parkinsonian animals, PET imaging showed high binding potential (BP) of the DAT ligand, 11C-CFT in the dorso-lateral part of the putamen (yellow arrow). White arrow shows high BP in the normal side of the putamen. H) Kinetic analysis of BP data obtained repeatedly till 7 months after transplantation. The kinetic analysis indicated that a major part of the grafted cells (∼ 90%) lost the expression of DAT (or degenerated) with a half-life of 10 days, while the rest of the grafted cells retained the DAT expression at the 7-month post-graft.
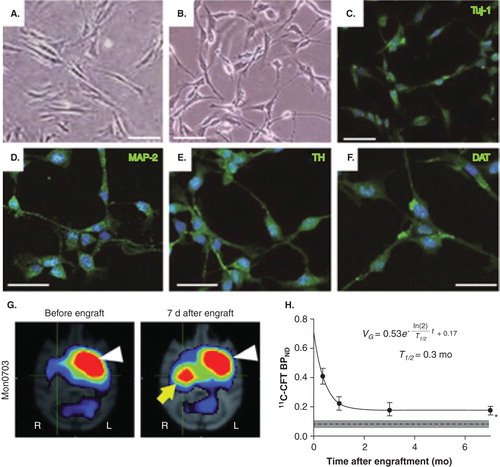
The gold standard for imaging the effects of cell therapy in PD has been the use of 18F-DOPA (dihydroxyphenylalanine) PET, which visualizes the enzymatic activity of aromatic amino acid decarboxylase (AADC) for dopamine synthesis, enabling us to evaluate the ability of the grafted tissues to produce dopamine in the PD brain. Kinetic analysis of 18F-DOPA PET data allows us to quantify the metabolic rate of 18F-DOPA in the tissue and to compare the values between subjects and conditions. It is useful for quantifying dopaminergic function in PD or PD-related diseases, as well as for evaluating fetal midbrain transplantation in PD; however, it has not been used for evaluating stem cell transplantations until very recently. Several studies that evaluate stem cell transplantation in preclinical animal models have shown usefulness of 18F-DOPA Citation[11], as well as 11C-DOPA Citation[12]. One of the concerns for using a ligand for DOPA is that since 18F-DOPA is an amino acid analog and is known to be accumulated in inflamed tissue and in the tumor, it may be difficult to distinguish whether the 18F-DOPA accumulation is due to higher dopaminergic function of the grafted cells or to increased tumorigenicity/inflammation Citation[13]. A potential solution to this issue may be to combine it with PET scans of 18F-OMFD, one of the major metabolites of 18F-DOPA. Inflammatory and/or tumorigenic cells can accumulate 18F-OMFD because they are metabolically active and can have increased retention of amino acids. 18F-OMFD easily gets across the blood–brain barrier; therefore it enters into the grafted cells, as well as brain tissues. However, inflammatory/tumorigenic cells may not have increased activity of AADC, which converts 18F-DA to 18F-OMFD and is normally expressed in the catecholaminergic, dopaminergic and serotonergic neurons. Therefore, combined analysis using 18F-DOPA and 18F-OMFD may potentially discriminate the specific and non-specific 18F-DOPA metabolism in the brain and graft tissues. This idea of combined analysis was initially proposed in 1996 Citation[14], but not been applied to the assessment of cell therapy. As described below, combining 18F-DOPA with other PET tracers for inflammation/cell overgrowth, for example, 11 C-PK11195 or 18F-fluorodeoxyglucose (FDG), may also help to discriminate expression of dopaminergic function from tumorigenicity/inflammation.
Typing of grafted cells with PET ligands for serotonergic neurotransmission may also help to optimize cell therapy. Contamination of the grafted cells with serotonergic cells has been associated with the formation of the adverse symptom dyskinesia, a kind of abnormal involuntary movement. PD patients who developed disabling dyskinesia after showing remarkable improvement of their motor symptoms with fetal midbrain tissue grafts were studied clinically and with PET ligands for serotonin, 11C-DASB Citation[15]. The results indicated excessive striatal serotonin innervation in all grafted sites. Therefore, serotonergic cells may need to be kept to a minimum or removed from dopaminergic neuron populations by cell sorting in future stem cell transplantation trials.
3.2 Cell survival and overgrowth
Stem cell therapy should be carefully evaluated for tumorigenicity of the grafts because stem cells have a potential for overgrowth. PET ligands clinically used for tumor detection can be useful for evaluating this. For example, a ligand for glucose metabolism, 18F-FDG, which is clinically used for detection of malignant tumors in the whole body, was also useful for evaluation of tumorigenicity for both MSC-derived Citation[8] and ES-derived neurons Citation[7]. Similarly, a ligand for nucleic acid metabolism, 18F-FLT, which is known to be useful for diagnosis of malignant brain tumors, specifically detected overgrowth of the ES-derived grafts Citation[7]. High-resolution T2-weighted MRI images also provided information on time-dependent changes of the graft volumes, allowing us to evaluate the ‘doubling times', that is, the rates of cellular overgrowth among different cells after grafting Citation[7].
On the other hand, the survival of graft tissues may be a problem in stem cell therapy. For example, MSCs are known to suffer from apoptosis after they are grafted; therefore, quantification of the survival rate of grafts in future studies may provide useful information to find better cell preparations for cell therapy. Repeated PET measurements may allow us to evaluate the long-term survival of the graft and the integrity of graft function. In fact, we have recently succeeded to assess the survival rate of the MSC-derived dopaminergic neurons by evaluating the DAT expression measured by PET and by applying the neuronal survival model () Citation[8]. The half-life period of the grafted cells was found to be around 10 days, which is considered very short and will need to be prolonged for more long-term efficacy in future studies. This evaluation method can thus be potentially used for quantitatively comparing the survival rate across different preparations of grafts.
3.3 Graft–host interaction: negative effects
Grafted tissues may function properly only when they are adapted to the surrounding host tissue. A favorable environment will allow the grafted cells to gain nutrition and to eliminate metabolites via vasculature, and to form cell-to-cell interactions that are hallmarks of functional neurons. However, there has been little information on key interactions between the graft and the host in successful cell therapy. One of the factors that limit proper graft–host interaction may be inflammatory or immune reactions by host glial tissues including astrocytes, microglia and oligodendrocytes. Histological studies of PD patients with clinical improvements after fetal cell transplantation, as well as those with less adverse effects of dyskinesia, showed less microglia, the resident macrophages of the brain, which act as the main form of active immune defense in the brain. Thus, less immune response by host microglia may be one of the factors that determine successful transplantation. A PET ligand, 11C-PK11195, has been used for imaging the translocator protein 18kDA (TSPO), which is expressed in the activated microglia and can potentially be used for assessing grafted brain tissues. A recently developed PET ligand for cyclooxygenase, 11C-ketoprofen-metylester, can also be used for imaging activated microglia Citation[16]. In our preliminary data using these two PET ligands, early microglial activation after grafting the stem cells seems to compromise the later survival of the grafted cells (). Therefore, in vivo microglial imaging may provide information useful to predict the long-term cell viability after stem cells are grafted into host tissue.
Figure 2. Potential predictability of the post-graft inflammatory response for delayed graft cell death. A. T2-weighted MRI brain image of a PD model animal, in which human embryonic stem cells were transplanted bilaterally in the striatum 1 month before MRI scan. B. At the same time point, PET ligands for activated glia, 11C-ketoprofen methyl ester (upper) and 11C-PK11195 (lower) were accumulated in the grafted areas of striatum, suggesting that host tissues have significant immune reactions to the grafted cells. C. Follow-up T2-weighted MRI scan in the same animal (3-month post-transplantation), which showed disappearance of grafts at this later stage. These findings suggest potential usefulness of multi-modal neuroimaging in predicting the long-term graft survival from early stage information of graft–host immune interaction. Unpublished data, courtesy of Prof. Jun Takahashi at Kyoto University.
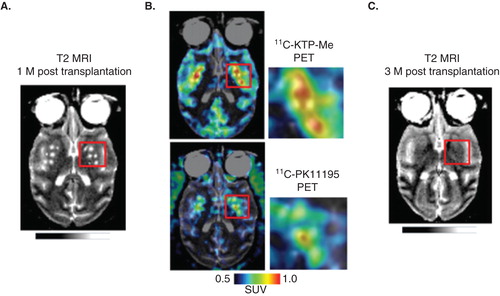
3.4 Graft–host interaction: positive effects
There may be several factors that accelerate the graft–host interaction and potentially lead to successful cell therapy. Neural interaction between the grafted dopaminergic neurons and host neurons may be among these factors. For example, previous studies with fetal midbrain tissue transplantation in PD suggest that re-innervation of the grafted dopaminergic neurons to the host neurons may underlie the mechanisms leading to beneficial effects in those who showed symptomatic improvements Citation[17]. Such an adaption to the host neurons may result from the fact that the grafted neurons were appropriately abundant in A9-type dopaminergic neurons which are needed in the host striatum. However, it is not well studied how this particular cell-type can restore the synaptic connections and release dopamine effectively. With this aim, functionality of dopaminergic synapses can be assessed in vivo by a PET ligand for dopamine D2 receptor, 11C-raclopride, which indirectly measures endogenous dopamine release in the synaptic clefts. It has in fact been used for evaluating function of grafts in PD patients Citation[18] and animal models Citation[12], and could thus be used for optimizing the cell preparations in future studies. A recent technique using resting state functional MRI (fMRI) may also provide information on local intrinsic connectivity, particularly in the striatum. Regional homogeneity of the signals was altered in the striatum (putamen) of PD patients and this was normalized by dopamine replenishment Citation[19]; therefore, this technique could potentially be used to indicate local functional connectivity between host and grafts. Because striatal glutamatergic overactivity is associated with formation of parkinsonian symptoms Citation[20], it may be of interest to study how this local functional connectivity in the striatum is related to the glutamatergic overactivity, and how this phenomenon has clinical significance.
PET has been used in past stem cell studies to quantify dopamine function; however, dopamine dysfunction is not the sole mechanism that explains the motor disturbances in PD. PD patients' motor symptoms only become evident when about 70 – 80% of striatal dopamine and about 50% of nigral dopamine neurons are lost. Thus, plasticity-related neural changes in addition to dopamine deficiency may underlie the mechanism of motor symptoms, and such plastic changes may be reflected in alterations of the large-scale functional network. For example, abnormal network activity involving the motor cortex and subthalamic nucleus is associated with movement dysfunction of PD, as revealed by resting state 18F-FDG PET measurements Citation[21]. Recent fMRI studies in PD patients also suggest abnormality in the large-scale circuitry that involves the subthalamic nucleus and motor cortices Citation[22]. Non-dopaminergic network abnormality is also of clinical significance, since it is a therapeutic target of deep brain stimulation (DBS), which is known to dramatically improve motor function of PD but not by mechanism of facilitated dopamine release. In addition, because the placebo effect that has been significant in PD trials also causes release of striatal dopamine in PD patients Citation[23], the mechanism that explains this therapeutic effect should be studied in detail. Therefore, resting state 18F-FDG PET or fMRI could potentially add further insights for understanding the mechanisms of PD symptomatology and can be used as a biomarker of the abnormal neural network in PD Citation[21].
3.5 Standardization and optimization of neuroimaging for stem cell therapy
It requires time and perseverance to ultimately achieve effective stem cell therapy. Stem cells will need to be optimized by improving the associated techniques, such as cell differentiation and the characterization of cells with cell markers, transcription codes or cellular electrophysiology. Furthermore, grafting techniques will need to be optimized by improving understanding of factors, such as determining the optimal location of grafts, and method of grafting (needle injection or en bloc grafting). Adaptation to host tissue environment and integrity of the host–graft interaction must be evaluated after the cells are grafted into the host tissue. In addition, long-term follow-up evaluations are needed for each cell therapy trial as conclusions cannot be drawn from short follow-up periods. Thus, neuroimaging techniques should be optimized and standardized to evaluate any type of cell graft, so that one can analyze and interpret the data in a cross-sectional manner, which is needed for setting technical targets. As noted, while 18F-DOPA PET has been consistently used since early open-label and recent double-blind trials of fetal cell therapy in PD, potential accumulation of this tracer in the cell overgrowth requires further evaluation in trials of stem cell therapy in PD. Therefore, for the present, multiple evaluations continue to be required for DAT expression, cell overgrowth, cell–graft interaction, non-dopaminergic neural function, as well as for DOPA synthesis.
4. Limitations of neuroimaging
Although neuroimaging techniques have significant advantages, such as non-invasiveness, ability of longitudinal and quantitative assessments, several factors may limit its utility and the data need careful interpretation. In particular, for applied research of neuroimaging, the researchers need to know the physical properties and limitations of each imaging device, for example, spatial resolution, temporal resolution, sensitivity and biochemical specificity (). The spatial resolution of neuroimaging techniques is relatively lower (∼ mm) than that expected for evaluating the cellular events (∼ m). Therefore, neuroimaging data cannot quantitate function for each cellular unit, but evaluate the net biological events that take place in the larger volume of tissues: thus, careful interpretation is needed to correlate the imaging data with biological events of the graft. In addition, the specific binding of PET ligand is often degraded by formation and uptake of metabolites of the ligand, as was discussed for 18F-DOPA; thus careful assessment of the data and its interpretation is required.
5. Expert opinion
It may not be realistic to expect stem cell therapy to induce complete functional recovery by replacing cells lost through disease, considering the complexity of human brain structure and function Citation[24]. Even for fetal cell therapy in PD, none of the clinical trials ever exceeded the effect size that was achieved by subthalamic DBS Citation[25]. Thus, one of the most important directions of future stem cell therapy in PD is to optimize and maximize the effect of therapy. Neuroimaging techniques may provide quantitative and multi-modal information for the effects of cell therapy in living host tissues in PD. PET with dopaminergic ligands such as 18F-DOPA, 11C-CFT, 11C-PE2I and 11C-raclopride allows us to assess the dopaminergic function of the graft. The tumorigenicity/inflammatory reactions can be visualized at an earlier stage by using 18F-FDG, 18F-FLT, 11C-PK11195 and 11C-ketoprofen methyl ester. In addition, functional networks associated with motor dysfunction can be evaluated by assessing local and large-scale functional networks using resting state fMRI. Future studies with neuroimaging should be directed to expand our knowledge and to understand unsolved issues of symptomatic mechanisms, such as the neural interaction between dopaminergic and non-dopaminergic systems or therapeutic mechanisms such as the cell–graft neural interaction, thereby finding new strategies to maximize the potential of stem cell therapies in PD.
Declaration of interest
This study was supported by a Grant from the Project for Realization of Regenerative Medicine from the Ministry of Education, Culture, Sports, Science and Technology (MEXT), and by the Program for Promotion of Fundamental Studies in Health Sciences of the National Institute of Biomedical Innovation (NIBIO, 05-06, 10-05). None of the authors have any competing interests to declare.
Notes
Bibliography
- Brundin P, Barker RA, Parmar M. Neural grafting in Parkinson's disease Problems and possibilities. Prog Brain Res 2010;184:265-94
- Liu S-P, Fu R-H, Huang S-J, et al. Stem cell applications in regenerative medicine for neurological disorders. Cell Transplant 2013;22:631-7
- Hayashi T, Shimazawa M, Watabe H, et al. Kinetics of neurodegeneration based on a risk-related biomarker in animal model of glaucoma. Mol Neurodegener 2013;8:4
- Thompson L, Barraud P, Andersson E, et al. Identification of dopaminergic neurons of nigral and ventral tegmental area subtypes in grafts of fetal ventral mesencephalon based on cell morphology, protein expression, and efferent projections. J Neurosci Off J Soc Neurosci 2005;25:6467-77
- Grealish S, Jönsson ME, Li M, et al. The A9 dopamine neuron component in grafts of ventral mesencephalon is an important determinant for recovery of motor function in a rat model of Parkinson's disease. Brain 2010;133:482-95
- Kriks S, Shim J-W, Piao J, et al. Dopamine neurons derived from human ES cells efficiently engraft in animal models of Parkinson's disease. Nature 2011;480:547-51
- Doi D, Morizane A, Kikuchi T, et al. Prolonged maturation culture favors a reduction in the tumorigenicity and the dopaminergic function of human ESC-derived neural cells in a primate model of Parkinson's disease. Stem Cells 2012;30:935-45
- Hayashi T, Wakao S, Kitada M, et al. Autologous mesenchymal stem cell-derived dopaminergic neurons function in parkinsonian macaques. J Clin Invest 2013;123:272-84
- Fu Y, Yuan Y, Halliday G, et al. A cytoarchitectonic and chemoarchitectonic analysis of the dopamine cell groups in the substantia nigra, ventral tegmental area, and retrorubral field in the mouse. Brain Struct Funct 2012;217:591-612
- Burbach JPH, Smidt MP. Molecular programming of stem cells into mesodiencephalic dopaminergic neurons. Trends Neurosci 2006;29:601-3
- Takagi Y, Takahashi J, Saiki H, et al. Dopaminergic neurons generated from monkey embryonic stem cells function in a Parkinson primate model. J Clin Invest 2005;115:102-9
- Muramatsu S-I, Okuno T, Suzuki Y, et al. Multitracer assessment of dopamine function after transplantation of embryonic stem cell-derived neural stem cells in a primate model of Parkinson's disease. Synapse 2009;63:541-8
- Kikuchi T, Morizane A, Doi D, et al. Survival of Human Induced Pluripotent Stem Cell–Derived Midbrain Dopaminergic Neurons in the Brain of a Primate Model of Parkinson's Disease. J Park Dis 2011;1(4):395-412
- Dhawan V, Ishikawa T, Patlak C, et al. Combined FDOPA and 3OMFD PET studies in Parkinson's disease. J Nucl Med Off Publ Soc Nucl Med 1996;37:209-16
- Politis M, Wu K, Loane C, et al. Serotonergic neurons mediate dyskinesia side effects in Parkinson's patients with neural transplants. Sci Transl Med 2010;2:38ra46
- Shukuri M, Takashima-Hirano M, Tokuda K, et al. In vivo expression of cyclooxygenase-1 in activated microglia and macrophages during neuroinflammation visualized by PET with 11C-ketoprofen methyl ester. J Nucl Med 2011;52:1094-101
- Mendez I, Sanchez-Pernaute R, Cooper O, et al. Cell type analysis of functional fetal dopamine cell suspension transplants in the striatum and substantia nigra of patients with Parkinson's disease. Brain J Neurol 2005;128:1498-510
- Piccini P, Brooks DJ, Björklund A, et al. Dopamine release from nigral transplants visualized in vivo in a Parkinson's patient. Nat Neurosci 1999;2:1137-40
- Wu T, Long X, Zang Y, et al. Regional homogeneity changes in patients with Parkinson's disease. Hum Brain Mapp 2009;30:1502-10
- Picconi B, Centonze D, Rossi S, et al. Therapeutic doses of L-dopa reverse hypersensitivity of corticostriatal D2-dopamine receptors and glutamatergic overactivity in experimental parkinsonism. Brain J Neurol 2004;127:1661-9
- Poston KL, Eidelberg D. Network biomarkers for the diagnosis and treatment of movement disorders. Neurobiol Dis 2009;35:141-7
- Baudrexel S, Witte T, Seifried C, et al. Resting state fMRI reveals increased subthalamic nucleus-motor cortex connectivity in Parkinson's disease. Neuroimage 2011;55(4):1728-38
- de la Fuente-Fernández R, Ruth TJ, Sossi V, et al. Expectation and dopamine release: mechanism of the Placebo Effect in Parkinson's disease. Science 2001;293:1164-6
- Lindvall O, Kokaia Z, Martinez-Serrano A. Stem cell therapy for human neurodegenerative disorders-how to make it work. Nat Med 2004;10(Suppl):S42-50
- Lindvall O, Björklund A. Cell therapeutics in Parkinson's disease. Neurother J Am Soc Exp Neurother 2011;8:539-48