Abstract
Gene therapy has received much attention in the field of drug delivery. Synthetic, nonviral gene delivery systems have gained increasing attention as vectors for gene therapy mainly due to a favorable immunogenicity profile and ease of manufacturing as compared to viral vectors. The great majority of these formulations are based on polycationic structures, due to their ability to interact with negatively charged nucleic acids to spontaneously form nanoparticles. In recent years, several polycationic systems have demonstrated high transfection in vitro. However, progress toward clinical applications has been slow, mainly because the cationic nature of these systems leads to intolerable toxicity levels, inappropriate biodistribution and unsatisfactory efficiency in vivo, particularly after systemic administration. Decationized polyplexes are a new class of gene delivery systems that have been developed as an alternative for conventional polycation-based systems. The major innovation introduced by decationized polyplexes is that these systems are based on neutral polymers, without any detrimental effect on the physicochemical stability or encapsulation ability, due to the transient presence of cationic charge and disulfide cross-links between the polymer chains by which the nucleic acids are physically entrapped in the particles. This editorial summarizes the most important features of decationized polyplexes and discusses potential implications for the development of new safe and efficient gene delivery systems.
1. Introduction
Gene therapy is a therapeutic modality that has been developed and evaluated to prevent or treat a broad range of conditions such as genetic disorders, degenerative diseases, infections and cancer Citation[1]. In order to transfect target cells, nucleic acids require safe and efficient gene delivery vectors. The natural ability of viruses to introduce foreign DNA (or RNA) into host cells makes them highly efficient gene delivery systems. Consequently, engineered viruses are the most commonly used delivery vehicles in clinical trials Citation[1]. However, the therapeutic use of viruses is associated with several risks, such as immune reactions or insertional mutagenesis, which requires alternative technologies to emerge Citation[1].
Nonviral vectors, particularly cationic polymers, have been investigated for more than two decades for gene therapy applications due to their low or absent immunogenicity and ease of pharmaceutical preparation when compared to viral vectors Citation[2]. However, polymeric gene delivery systems have failed to become a reality in clinical settings not only because their insufficient in vivo efficiency but also because they are associated with serious toxicity issues. Cationic polymers form nanoparticles with nucleic acids, such as plasmid DNA (pDNA) or small interfering RNA (siRNA), named polyplexes, via electrostatic interactions, but stable particles are only formed when an excess of cationic polymer is present in the formulation. This excess of cationic polymers results in an overall positive charge of the polyplexes, which has a substantial impact on the in vivo stability and toxicity. It was found that, for example, polyplexes based on polyethylenimine (PEI) (the most commonly used polymer in gene delivery) upon systemic administration, immediately interact with negatively charged blood components (e.g., proteins, erythrocytes) leading to the formation of aggregates, which caused lung embolisms and animal death. Polycations are also associated with blood clot formation Citation[3], complement activation, immunogenicity and liver necrosis, as well as uncontrollable biodistribution and transfection Citation[4,5]. The acute toxicity observed was found to be strongly related to the presence of excess free cationic polymers in the polyplex samples. In order to reduce the acute toxicity in vivo of PEI formulations, Boeckle et al. Citation[6] have developed a technique to purify PEI polyplexes prepared at high N/P ratio by size exclusion chromatography. The purified polyplex formulations possessed a decreased systemic toxicity; however, it was shown that the charge of the particles remained highly positive (> 20 mV). Consequently, toxicity of these formulations was still highly dependent on the polyplex concentration. A more preeminent and straightforward approach to reduce acute toxicity in vivo consists of shielding polyplexes with hydrophilic polymers like PEG. This modification can prevent systemic toxicity by reducing interactions with blood components and formation of aggregates, resulting in improved circulation kinetics and target tissue accumulation Citation[7,8]. However, PEG shielding is only partial since PEGylated polyplexes are still rapidly cleared compared to other well-known delivery systems (e.g., PEGylated liposomes), resulting in short blood circulation half-lives Citation[9,10]. Alternatives to this approach consist of masking the net positive charge of the pre-formed complexes, by modification of with polyanions Citation[11,12]. This results in the formation of negatively charged ternary complexes, which have shown decreased toxicity, improved in vivo stability and therapeutic effects in vivo Citation[11]. Another interesting approach concerns polymer/calcium phosphate polymer (CaP) hybrid nanoparticles. Pittella et al. have used such approach for siRNA delivery purposes, where siRNA/CaP particles were stabilized by a PEG-b-polyanion copolymer. The polyanions possessed charged convertional properties; the anionic functional groups were converted to cationic groups in the endosomal acidic conditions providing endosomal escape functionalities to the system. Such nanoparticles were able to target tumors and effectively reduce tumor growth by antiangiogenic therapy Citation[13]. Both strategies have shown promising results in vivo; however, such approaches do not avoid the presence of cationic polymers intracellularly.
Avoidance of cationic polymers in a delivery system of particular importance since polycations can induce cellular toxicity at many levels. Polycations can disrupt cellular homeostasis by compromising the cell membrane integrity, interacting with cellular polyanions, changing the cell expression profile or by activation of oncogenes and by induction of cell apoptosis Citation[14,15]. The toxicity of polyplexes can be decreased to some extent by using biodegradable polymers Citation[16] or by decreasing the polymer charge density Citation[11]. Nevertheless, neutral polymer-based systems are a solid alternative to conventional that require polycationic structures. The current editorial focuses on decationized polyplexes and their importance to substantially improve the in vivo safety profile of polymeric gene delivery systems both at systemic and cellular levels. These systems were developed and evaluated in our department and summarized in the next sections.
2. Decationized polyplexes structural design
Decationized polyplexes are exclusively based on neutral polymers, and structurally constituted of a PEG corona and a disulfide cross-linked poly(hydroxypropyl methacrylamide) (pHPMA) core to encapsulate and retain the nucleic acids such as pDNA. The preparation of decationized polyplexes occurs essentially in three steps (). Firstly, pDNA is complexed with the block copolymer of the cationic precursor p(HPMA-DMAE-co-PDTEMA)-b-PEG (pHDP–PEG) (which can contain a desired targeting ligand) exploiting electrostatic interactions. In the second step, the polyplexes are stabilized by interchain disulfide cross-linking of the pyridyl disulfide groups present in the core of the polyplexes using a multifunctional thiol (such as dithiothreitol), yielding cationic pHDP–PEG polyplexes. In the last step, decationized polyplexes are obtained by removing the now unnecessary dimethylaminoethanol (DMAE) cationic side groups by hydrolysis, yielding p(HPMA-co-PDTEMA)-b-PEG (pHP–PEG) decationized polyplexes. With this protocol, we obtain neutral polymer-based polyplexes in which pDNA is loaded in the core exclusively by physical entrapment due to the presence of disulfide cross-links. This property is particularly innovative when compared to previously developed disulfide-containing polyplexes, which require electrostatic interactions for pDNA entrapment. The stabilization of with disulfide cross-links is particularly elegant strategy because ensures the stability of the polyplexes in the circulation avoiding unwanted polyplex disassembly in the bloodstream. On the other hand, disulfide bonds are rapidly reduced in the intracellular environment, resulting in polyplexes destabilization and release of the nucleic acid loading Citation[17,18]. Accordingly, by stabilization with interchain disulfide cross-linking, decationized polyplexes possess a triggered gene release mechanism upon cell entry. Furthermore, the particles are covered with PEG chains, enabling the introduction targeting ligands, which can be employed to facilitate cellular uptake.
Figure 1. Schematic representation of the general approach for the preparation of (targeted) decationized polyplexes, via a three-step process: 1) Charge-driven condensation with nucleic acids using the pHDP–PEG polycation precursor; 2) stabilization of polyplexes by interchain disulfide cross-linking; 3) removal of the cationic DMAE groups under mild conditions resulting in decationized pHP–PEG polyplexes. Targeting ligand (e.g., folic acid) is used to improve the degree and specificity of uptake.
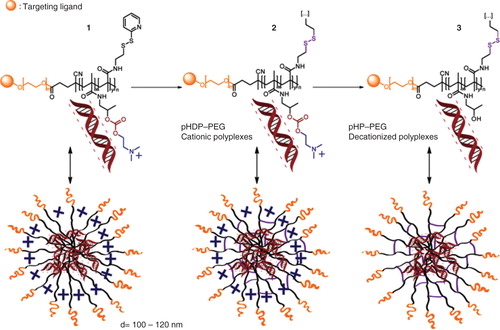
3. Decationized polyplexes properties
Decationized polyplexes have demonstrated important and unique properties when compared to conventional polyplexes. Decationized polyplexes generally have a size of ∼ 120 nm and a slightly negative zeta-potential (−5mV) (vs ∼ 120 nm and +10 mV for the pHDP–PEG cationic polyplexes), excellent colloidal stability and triggered pDNA release in a reductive environment Citation[19]. Another important characteristic of decationized polyplexes is their low degree of nonspecific cell uptake, which is a requirement for targeted therapies by using cell-specific ligands that are coupled on the surface of the polyplexes. Upon the development of folate-targeted decationized polyplexes, in vitro tests have shown that targeted decationized polyplexes showed significantly higher cellular uptake in a folate receptor overexpressing cell line (OVCAR-3), when compared to their nontargeted counterparts. On the other hand, for the cell line (A549) with low folate receptor expression, a similar uptake was observed for both targeted and nontargeted decationized polyplexes. Furthermore, the improved cellular uptake for targeted systems had a direct correlation with improved transfection observed in OVCAR-3 cells Citation[20]. Importantly, the nontargeted cationic system showed the highest cellular uptake, mainly due to nonspecific interaction between the positively charged polyplexes membrane anionic components, which is not favorable for highly specific targeted delivery which requires low uptake by nontarget cells ().
Figure 2. Main decationized polyplex properties. A. Cell-specific uptake of folate-targeted (pHP–PEG–FA) decationized polyplexes by folate receptor overexpressing (FR +) OVCAR-3 (a) cells and low degree of unspecific uptake by A549 cells (FR −) (b). B. Excellent cytocompatibility, observed by (a) high relative HUVEC cell viability upon exposure for 72 h to the decationized pHP–PEG, neutral polymer, and observed by (b) no mortality and low interference on zebrafish embryos upon exposure to decationized pHP–PEG, *significant mortality, ‡significant developmental defects (i.e., decreased pigmentation, delayed hatching ratio and slower heartbeat). C. Improved blood circulation half-life (a) and target tissue (tumor) accumulation determined by noninvasive in vivo imaging 3D CT-FMT (b) or ex vivo two-dimensional fluorescence reflectance imaging quantification (and respective images) (c) of near-infrared fluorophore-labeled polyplexes upon systemic administration in tumor bearing mice, *p < 0.01 (t test).
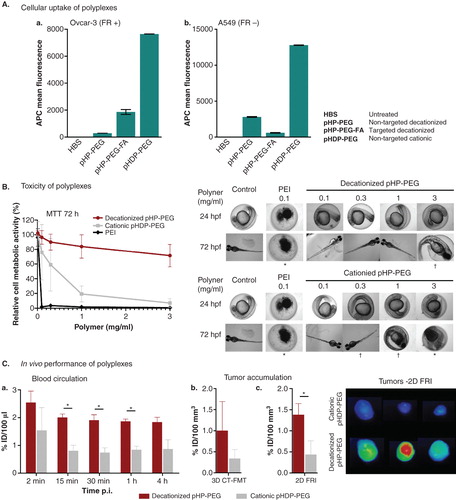
Additionally, as anticipated, decationized polyplexes have shown an excellent safety profile and extensive in vitro tests have shown that decationized polyplexes do not interfere with cell viability nor induce membrane destabilization at any of the doses tested Citation[19,20]. Furthermore, the neutral block copolymer pHP–PEG that forms decationized polyplexes showed excellent cytocompatibility upon incubation with HUVEC cells. Finally, an in vivo zebrafish nanotoxicity assay Citation[21] also demonstrated that this polymer had a remarkable low teratogenicity and mortality profile, in great contrast with its biodegradable cationic counterpart () Citation[22].
The in vivo applicability of decationized polyplexes for systemic administration was evaluated by determining the stability of decationized polyplexes in full human plasma and a notably stable size distribution was observed for 48 h, whereas their cationic counterpart showed some degree of aggregation Citation[22]. Polyplexes were also evaluated for their biodistribution and tumor accumulation by noninvasive optical imaging (computed tomography - fluorescence molecular tomography Citation[23]), upon intravenous administration in A431 tumor-bearing mice. When compared to cationic polyplexes, decationized polyplexes exhibited a prolonged blood half-life time and an increased tumor accumulation (). Furthermore, decationized polyplexes were able to induce reporter transgene expression in vivo Citation[22].
4. Conclusions
Decationized polyplexes have shown an initial proof of principle for the fulfilling of important requirements for gene delivery systems. Because of the lack of an excess of cationic polymer, decationized polyplexes have an excellent safety profile. Furthermore, removal of cationic charges after introduction of disulfide cross-links, further limits the unspecific interactions of the polyplexes, as observed by the higher stability in human plasma. The improved stability and simultaneous low degree of unspecific uptake seem to be the driving force of the improved in vivo circulation half-life and target tissue accumulation. These features hold great promise for future systems based on the decationized polyplexes, especially for targeted therapies requiring systemic administration.
5. Expert opinion
Decationized polyplexes provide a unique platform for the preparation of new polymeric gene delivery systems. The innovative approach of decationized polyplexes is particularly important in gene delivery mainly due to the safety profile and low levels of a specific transfection. Due to safety of a neutral polymer-based system, repetitive administrations and high doses are likely not an obstacle for decationized polyplexes, as opposed to most of the conventional polyplexes. This possibility can compensate an apparent lower efficiency of decationized polyplexes. Such advantages are important not only for pDNA delivery, which so far have been primarily the focus of our research, but are also important for the delivery siRNA or mRNA gene therapy modalities as well. The flexibility of these systems allows tuning of the structural design to adapt to different nucleic acids. For example, recently decationized polyplexes have been successfully used for siRNA applications Citation[24], making RNA delivery an important area for future research. Furthermore, the ability of targeted decationized polyplexes to specifically interact with the target cells allows their potential use for a wide range of biomedical and pharmaceutical applications. By choosing the adequate targeting ligand Citation[25] decationized polyplexes can be an interesting tool for vaccination, tissue regeneration, cardiovascular or metabolic disorders.
Even though important findings were obtained, further information on the in vivo performance of decationized polyplexes is required. Understanding the in vivo therapeutic potential of decationized polyplexes, particularly the targeted versions, is crucial for determining the future areas of development of decationized polyplexes. Most likely, in vivo efficiency of polyplexes needs further optimization. First, the circulation half-life can be additionally extended by improvement of stability or by tuning the polymer structure (e.g., PEG density and molecular weight). Second, an important challenge that decationized polyplexes face is to identify the proper functionalities that can be introduced in the particles to overcome some of the cellular barriers for transfection. Particularly, improvements of polyplex endosomal escape and nuclear localization (for pDNA applications) should be implemented to increase transfection efficiency without adversely affecting their safety profile. The challenges are great, but the flexibility of these systems enables adjustments that can yield optimized versions of decationized polyplexes that will without doubt be a serious competitor for viral vectors for systemic gene delivery applications.
Declaration of interest
The authors have no relevant affiliations or financial involvement with any organization or entity with a financial interest in or financial conflict with the subject matter or materials discussed in the manuscript. This includes employment, consultancies, honoraria, stock ownership or options, expert testimony, grants or patents received or pending, or royalties.
Notes
Bibliography
- Ginn SL, Alexander IE, Edelstein ML, et al. Gene therapy clinical trials worldwide to 2012 – an update. J Gene Med 2013;15(2):65–77
- Mintzer MA, Simanek EE. Nonviral vectors for gene delivery. Chem Rev 2008;109(2):259-302
- Jones CF, Campbell RA, Brooks AE, et al. Cationic PAMAM dendrimers aggressively initiate blood clot formation. ACS Nano 2012;6(11):9900-10
- Chollet P, Favrot MC, Hurbin A, et al. Side-effects of a systemic injection of linear polyethylenimine–DNA complexes. J Gene Med 2002;4(1):84-91
- Ogris M, Brunner S, Schuller S, et al. PEGylated DNA/transferrin-PEI complexes: reduced interaction with blood components, extended circulation in blood and potential for systemic gene delivery. Gene Ther 1999;6(4):595-605
- Boeckle S, von Gersdorff K, van der Piepen S, et al. Purification of polyethylenimine polyplexes highlights the role of free polycations in gene transfer. J Gene Med 2004;6(10):1102-11
- Verbaan FJ, Oussoren C, Snel CJ, et al. Steric stabilization of poly(2-(dimethylamino)ethyl methacrylate)-based polyplexes mediates prolonged circulation and tumor targeting in mice. J Gene Med 2004;6(1):64-75
- Harada-Shiba M, Yamauchi K, Harada A, et al. Polyion complex micelles as vectors in gene therapy – pharmacokinetics and in vivo gene transfer. Gene Ther 2002;9(6):407-14
- de Wolf HK, Snel CJ, Verbaan FJ, et al. Effect of cationic carriers on the pharmacokinetics and tumor localization of nucleic acids after intravenous administration. Int J Pharm 2007;331(2):167-75
- Oupický D, Ogris M, Howard KA, et al. Importance of lateral and steric stabilization of polyelectrolyte gene delivery vectors for extended systemic circulation. Mol Ther 2002;5(4):463-72
- Zhou J, Liu J, Cheng CJ, et al. Biodegradable poly(amine-co-ester) terpolymers for targeted gene delivery. Nat Mater 2012;11(1):82-90
- Schlegel A, Largeau C, Bigey P, et al. Anionic polymers for decreased toxicity and enhanced in vivo delivery of siRNA complexed with cationic liposomes. J Control Release 2011;152(3):393-401
- Pittella F, Miyata K, Maeda Y, et al. Pancreatic cancer therapy by systemic administration of VEGF siRNA contained in calcium phosphate/charge-conversional polymer hybrid nanoparticles. J Control Release 2012;161(3):868-74
- Lonez C, Vandenbranden M, Ruysschaert J-M. Cationic lipids activate intracellular signaling pathways. Adv Drug Deliv Rev 2012;64(15):1749-58
- Parhamifar L, Larsen AK, Hunter AC, et al. Polycation cytotoxicity: a delicate matter for nucleic acid therapy-focus on polyethylenimine. Soft Matter 2010;6(17):4001-9
- Luten J, van Nostrum CF, De Smedt SC, et al. Biodegradable polymers as non-viral carriers for plasmid DNA delivery. J Control Release 2008;126(2):97-110
- Meng F, Hennink WE, Zhong Z. Reduction-sensitive polymers and bioconjugates for biomedical applications. Biomaterials 2009;30(12):2180-98
- Brülisauer L, Gauthier MA, Leroux J-C. Disulfide-containing parenteral delivery systems and their redox-biological fate. J Control Release 2014;195(0):147-54
- Novo L, van Gaal EVB, Mastrobattista E, et al. Decationized crosslinked polyplexes for redox-triggered gene delivery. J Control Release 2013;169(3):246-56
- Novo L, Mastrobattista E, van Nostrum CF, et al. Targeted decationized polyplexes for cell specific gene delivery. Bioconjug Chem 2014;25(4):802-12
- Rizzo LY, Golombek SK, Mertens ME, et al. In vivo nanotoxicity testing using the zebrafish embryo assay. J Mater Chem B 2013;1(32):3918-25
- Novo L, Rizzo LY, Golombek SK, et al. Decationized polyplexes as stable and safe carrier systems for improved biodistribution in systemic gene therapy. J Control Release 2014;195(0):162-75
- Kunjachan S, Gremse F, Theek B, et al. Noninvasive optical imaging of nanomedicine biodistribution. ACS Nano 2013;7(1):252-62
- Novo L, Takeda KM, Petteta T, et al. Targeted Decationized Polyplexes for siRNA Delivery. Mol Pharmaceutics 2014. 10.1021/mp500499x
- Wong SY, Pelet JM, Putnam D. Polymer systems for gene delivery – Past, present, and future. Prog Polym Sci 2007;32(8-9):799-837