Abstract
We investigated the seasonal snow cover at six islands of Franz Josef Land in July 1995. Samples were chemically analyzed for major ions, pH, conductivity, fluoride, acetate, formate, and methane sulfonate. The chemical composition of the snow cover was dominated by sodium and chloride, which contributed up to 70% of the ion balance. Mean nitrate concentration was about 2 &mu= L−1, and non-sea-salt sulfate ranged from 5 to 19 &mu= L−1. Ion concentrations revealed a high local and seasonal variability, with peak concentrations attributed to spring and summer snow. We also found a high local variability for the water equivalent of the snow cover, with values between 14 and 118 cm, reflecting the strong influence of wind drift and redistribution of snow. The fractionation of ions during snowmelt was followed by sampling the snow cover at Salisbury Island at three stages of the snowmelt.
Introduction
For the last 25 to 30 yr numerous studies have demonstrated that the northern polar regions are far from pristine. Chemical analysis of Greenland ice cores, for instance, revealed a significant increase of sulfate and nitrate concentrations from preindustrial levels to the 1980s (CitationNeftel et al., 1985; CitationFinkel et al., 1986; CitationMayewski et al., 1990), but elevated concentrations of heavy metals, persistent organic pollutants, polycyclic aromatic hydrocarbons, or black carbon are reported from arctic aerosol, snow, and ice as well (e.g., CitationBoutron et al., 1995; CitationPeters et al., 1995; CitationBarrie, 1996; CitationMacdonald et al., 2000; CitationBerg et al., 2001). The occurrence of arctic haze in late winter and early spring is predominantly related to fine particles of winter aerosol, which are mainly composed of anthropogenic sulfate, i.e., sulfuric acid and ammonium bisulfate (CitationPacyna, 1995; CitationShaw, 1995; CitationBarrie, 1996). Measured sulfate concentrations in the aerosol of the Norwegian Arctic reached maximum values at the beginning of the 1980s (CitationPacyna, 1995) and showed a decrease between 1980 and 1992, thus corresponding to reported SOx emissions in Eurasia (CitationBarrie, 1996). No major trend, however, has been observed in the Canadian Arctic at Alert and in Alaska at Barrow. The increased pollution of the Arctic is attributed to the long-range transport of anthropogenic trace constituents from middle to high latitudes, so local anthropogenic sources in the polar regions may be neglected. Most of the poleward transport of pollutants occurs during the cold season blocking conditions, which enhance the meridional exchange of air (e.g., CitationPacyna, 1995; CitationShaw, 1995; CitationIversen, 1996). In winter, in particular, the circulation of the lower arctic troposphere is determined by high-pressure systems over the continents and low-pressure systems over the northern oceans. Main pathways for the air transport into the Arctic are thus over the Atlantic and Pacific Oceans and over Eurasia, the former being most frequent. Several investigations, however, stress the importance of anthropogenic emissions in Eurasia, which contribute the major part of pollutant input to the Arctic (e.g., CitationRahn, 1981). Most studies on the past and current status of arctic pollution have been carried out in the Canadian Arctic, Alaska, Greenland, and Spitzbergen. Comparably little information, in particular concerning the deposition of atmospheric trace substances, is available so far in literature from the Russian part of the Arctic (e.g., CitationSmirnov et al., 1998; CitationVinogradova, 2000).
In view of the numerous results demonstrating the influence of anthropogenic emissions on the chemical composition of both arctic atmosphere and precipitation, we wanted to investigate the chemical composition of the seasonal snow cover at Franz Josef Land. One major objective was to determine the main contributors to the ion content of the snowpack, i.e., whether natural sources or anthropogenic emissions dominate the chemical composition of snow at the Russian archipelago. The study was performed at six islands of Franz Josef Land in July 1995. The local and seasonal variability and the major factors determining the ion content of the snow cover are discussed.
Methods
STUDY AREA
Franz Josef Land, about 900 km from the North Pole, is a highly dissected archipelago in the Russian Arctic ()—70% of the almost 200 islands contribute only 0.4% of the total area. Glaciers cover 85% of the land area. The highest elevation is 670 m a.s.l. (CitationGlazovskij, 1995; CitationMacheret et al., 1999). Mean annual temperature amounts to −12 to −13°C (CitationDowdeswell, 1995), and temperature is highest in July. With a mean value of 0 to 2°C, July temperatures range 3 to 5°C below the corresponding temperatures at the west coast of Spitzbergen or in the Canadian Arctic (CitationHisdal, 1995). Annual precipitation is low, with a mean amount of 100–150 mm yr−1 close to sea level (CitationHisdal, 1995), but may reach several hundred millimeters at higher elevations.
SAMPLING AND ANALYSIS
In July 1995 we sampled the seasonal snow cover at six islands of the archipelago (): Rudolf, Eva-Liv, Wiener Neustadt, Hall, Salisbury, and Wilczek Land. All sampling sites were situated at flat, wide domes at heights between 160 and 500 m a.s.l. Snow pits were dug down to the first major ice layer, which was assumed to represent the summer horizon of the previous year, as meltwater had not penetrated the pack. This ice layer clearly differed in hardness and thickness from the ice lenses above. It consisted of extremely hard and generally clear ice of unknown (but more than 10 to 15 cm) vertical extension, whereas the ice lenses in the overlying snow cover did not exceed 1-cm thickness.
Snow samples were taken along vertical profiles at increments of generally 10 to 20 cm. Snow was cut off with a tube of stainless steel and placed in airtight polyethylene bottles. Further details on the sampling procedure and precautions against sample contamination are described in CitationNickus et al. (1997). Concomitant with the chemical snow sampling, vertical profiles of snow density, stratigraphy, and temperature were recorded at each site.
Samples were thawed at the camp at Franz Josef Land, and after we measured pH and conductivity, they were stabilized with chloroform (0.2%, v/v) (CitationTartari et al., 1995). Fractions of 100 ml each were placed in polyethylene bottles for analysis of inorganic ions and in glass bottles for determination of organic acids.
Both inorganic and organic ions were analyzed with ion exchange chromatography using a Dionex device (DX-100 combined with DX-500) with AS12A and CS12 analytical columns. Method detection limits, defined as the minimum concentration of a substance that can be measured with 99% confidence (CitationLongbottom and Lichtenberg, 1982), amounted to about 1 μg L−1 for most ions (CitationNickus and Kuhn, 1994). Higher values were found only for sodium, with almost 4 μg L−1, and for acetate with almost 5 μg L−1. The relative standard deviation remained below 3% for ion concentrations <10 μg L−1 and was up to 10% for sodium and acetate concentrations less than 10 μg L−1.
Results
PHYSICAL PARAMETERS OF THE SNOW COVER
The snow depths at the sampling sites () revealed a high regional variability, with values ranging between 40 and 230 cm. However, no correlation could be found between snow accumulation and altitude, aspect, or latitude of the sites. The amount of deposited snow seemed rather to be strongly influenced by wind drift and redistribution of snow. Sounding with an avalanche probe 50 to 100 m around the pits revealed that the small-scale variability of the snow depth in the vicinity of the selected sites was low, generally deviating less than 10% from the respective depth of the pits, and was not influenced by elements of the surface structure, such as sastrugi. Mean snow density varied between 300 and 500 kg/m3, and the respective water equivalent at the sampling sites ranged from 14 to 118 cm ().
A dry snowpack was found only at Rudolf Island, the first-sampled and highest-elevated site, with negative snow temperatures throughout the entire snowpack. At all other sites the uppermost 10 to 40 cm had temperatures around 0°C, and values decreased to about −5°C at the base of the seasonal snowpack. During the sampling period, 4 July to 21 July, the snow cover revealed an ongoing wetting, which may be attributed largely to the generally positive mean air temperatures in July (). Due to this wetting within the sampling period, a variable number of thin ice lenses was generally found in the upper half of the snow cover, except for Rudolf Island, with a completely dry snowpack and no ice, and the site at Wilczek Island. There, the snowpack was sampled at the end of the sampling period, and ice lenses and layers of frozen rounded polycrystals formed by the refreezing of the percolating meltwater were found down to a depth of 190 cm, total depth being 230 cm. The occurrence of melt-freeze cycles at Wilczek Island certainly altered the vertical distribution of ion concentrations in the snowpack, and we could not exclude preferential elution of distinct ions (in particular sulfate—see Discussion: Fractionation of Ions). The release of trace substances from the snowpack could be followed nicely at Salisbury Island, where the same site was sampled three times within 2 wk.
ION CONCENTRATIONS AND DEPOSITED LOADS
Results of the snow sampling at six islands are summarized in and . The two dominating ions in the snowpack at Franz Josef Land were chloride and sodium, with mean concentrations between 14 and 38 &mu= L−1. Sulfate ranged from 4 to 13 &mu= L−1. The input of nitrogen was generally low, with nitrate and ammonium concentrations <4 &mu= L−1. Mean calcium concentrations ranged around 3 &mu= L−1, potassium was ≤1 &mu= L−1, and magnesium varied between 3 and 7 &mu= L−1. Two orders of magnitude below that lay the mean fluoride concentrations. Of the organic compounds, acetic, formic, and methane sulfonic acid were determined. Mean concentrations of acetate ranged between 1 and 3 &mu= L−1, and they were up to 1.7 &mu= L−1 for formate and up to 0.3 &mu= L−1 for methane sulfonic acid. Mean free acidity of the snow cover lay between 3 and 9 &mu= L−1.
The regional variability of both water equivalent and mean ion concentrations combined to give marked differences of the deposited ion loads. Like the water equivalent, the accumulated loads of most ions varied by a factor of 9 to 10 between the sampling sites. Only the deposited amounts of nitrate and sulfate were more evenly distributed: the respective values differed by a factor of 3 to 5.
Discussion
MAJOR SOURCES CONTRIBUTING TO THE ION CONTENT
A varimax normalized Principal Component Analysis (PCA) was performed in order to evaluate the major sources which contribute to the ion content of the snow cover. Mean ion concentrations already revealed the importance of the sea-salt aerosol (); therefore, the concentration of the non-sea-salt sulfate (nss-SO4 2−) was used instead of the total sulfate for both the correlation matrix () and the PCA.
Non-sea-salt sulfate was calculated using sodium as the reference species for seawater and a SO4
2− to Na+ ratio of 0.121 (CitationRiley and Skirrow, 1965), with concentrations given in &mu= L−1.
Two factors were derived from the PCA with eigenvalues >1 (according to the Kaiser criterion), explaining 91% of the total variance (). Factor 1 was loaded with chloride, sodium, potassium, magnesium, and calcium and is associated with the sea-salt aerosol. Almost 79% of the total variance in the chemical composition of the snowpack can be explained by the contribution of the sea-salt aerosol. Factor 1 may, however, also include some influence of soil-derived dust. The high correlation of Ca2+ with K+ and Mg2+ (r2 = 0.93 and 0.78) () indicates the input of dust containing silicate and dolomite. However, the influence of dust on the chemical composition of the snow cover was too small to show up as a third factor with an eigenvalue >1, so dust accounted for only a random variability of the snow chemistry at Franz Josef Land.
The second factor covered about 12% of the total variance and was loaded with NO3 −, nss-SO4 2−, NH4 +, and H+. It represents the input of acid components, i.e., sulfuric and nitric acid, but also of ammonium sulfate, ammonium bisulfate, and ammonium nitrate ([NH4]2SO4, NH4HSO4, NH4NO3). The free acidity of the snowpack seemed to be associated more with sulfate than with nitrate, and the association was expressed by the better correlation of H+ and nss-SO4 2− than compared to NO3 − (r2 = 0.84 and 0.56). Ammonium was also better correlated with non-sea-salt sulfate than with nitrate (r2 = 0.78 and 0.52), revealing a higher probability of its being deposited as ammonium sulfate than as ammonium nitrate.
Generally, the fraction of non-sea-salt sulfate amounted to about 70% of the total sulfate at Franz Josef Land (). Only at Wilczek Island was the nss-SO4 2−/SO4 2− ratio, at 0.2, clearly below this average. Preferential elution of the sulfate ion (see Discussion: Fractionation of Ions) in respect to Na+, which was used to derive the sea-salt part of the total sulfate concentration (see Equation 1), may account for the low sulfate ratio. In particular, the higher nss-SO4 2− concentrations of the upper snow layers, which were determined at the other sites, had been reduced at Wilczek Island by preferential elution of sulfate prior to sampling.
The Cl−/Na+ ratio was close to the seawater ratio of 0.165 (CitationRiley and Skirrow, 1965) at all sites (). Therefore, we assumed that the sea-salt aerosol was the major source for these ions; neither HCl nor dust-derived sodium seemed to contribute much to the chloride and sodium content of the snow cover. Similarly, the concentration ratio of Mg2+ to Na+ was in good accordance with the corresponding value for seawater: Mg2+/Na+ = 0.227. Only the snow cover at Wilczek Island revealed a lower concentration ratio of 0.16 (). Since this low value could not be explained by an increased Na+ concentration, we suspected that the Mg2+ ion was preferentially eluted compared to Na+ (see Discussion: Fractionation of Ions). Both the Cl−/Na+ and Mg2+/Na+ ratios, with values close to the seawater ratios, support the results obtained by the PCA that sea salt was the dominant source for ions in the snow cover at Franz Josef Land.
LOCAL VARIABILITY OF THE SNOW COVER
The water equivalent of the snow cover at the six investigated islands of Franz Josef Land varied by a factor of 10, with values ranging from 14 to 118 cm water equivalent (see Physical Parameters of the SnowCover). The high variability could not be explained by differing elevation or orography of the distinct sampling sites but only by wind drift and redistribution of snow. This explanation applies, in particular, to the small-scale variability of water equivalent and ion concentrations found for the two sites at Wiener Neustadt ( and ). Both sites were situated on a flat, wide dome, with a horizontal distance between the sites of about 100 m. The snow cover at site 1 revealed ion concentrations that were on an average about 20% lower than those at site 2. Moreover, due to a 13% lower water equivalent at site 2, deposited loads there reached only about 70% of the loads found at site 1.
Wind drift is a common feature in polar regions and clearly affects both the physical characteristics of a snow cover and its chemical composition (CitationPomeroy et al., 1991; CitationPomeroy and Jones, 1996). Once deposited, snow may be eroded again and, snow crystals are abraded, split and/or sublimated while being transported.
The threshold wind speed, i.e., the wind speed at the initiation of snow particle transport, ranges between 6 and 10 m s−1 at 10-m height, being lowest for dry and fresh snow at temperatures near −25°C (CitationPomeroy and Brun, 2001). The transport rate of snow for a 1-m air column clearly increases with wind speed (u) at 10-m height, from less than 1 g s−1 for u = 8 m s−1 to about 1 kg s−1 at a wind speed of 25 m s−1. Due to the nonlinear increase of the transport rate with wind speed, CitationPomeroy and Jones (1996) assumed that most of the annual snow transport may be caused by extreme wind events. In view of these results, both the temporary high wind speed at Franz Josef Land and the low air temperatures—monthly mean values generally remain below −20°C from November through March (meteorological observer at Krenkel station, pers. comm.)—represent conditions favorable for frequent wind drift and redistribution of snow.
Wind redistribution also affects the chemical composition of a snow cover. In particular, sublimation of water vapor, volatilization of chemical components, and scavenging of particles and vapors from the atmosphere by the blowing snow may alter the chemical composition (CitationPomeroy and Jones, 1996). Generally, sulfate and ammonium tend to be conservative ions, the concentration of which increases with the rate of sublimation and hence (exponentially) with wind speed but varies with climate and terrain. Sea-salt aerosols are preferentially scavenged species, while HNO3 belongs to the volatile components at high sublimation rates.
The various effects of wind redistribution on the snowpack of Franz Josef Land could not be assessed quantitatively and should be kept in mind during the discussion of results on snow accumulation and ion concentrations and loads.
REGIONAL VARIABILITY OF ION CONCENTRATIONS
Regional differences in the ion content of the arctic seasonal snowpack may be attributed to the atmospheric circulation patterns. In particular, on the western rim of the strong Siberian anticyclone that persists during the cold season, air is transported northward from heavily polluted midlatitude areas in Eurasia, with the arctic front lying south (CitationBarrie, 1986; CitationPacyna, 1995; CitationShaw, 1995; CitationIversen, 1996). Though two other main pathways for air to enter the Arctic exist, over the Atlantic and the Pacific Oceans, air from Europe and Eurasia seems to contribute the major part of arctic pollution. CitationIversen (1989), for instance, estimated anthropogenic sulfur budgets in the Arctic for a distinct year and found that in March, Eurasia contributed 95% and North America 5%, with the North American part increasing to 14% in July. CitationBarrie et al. (1989) gave similar numbers. During the cold season, when air masses move into the Arctic along the western rim of the Siberian anticyclone, and then over North America or the Aleutians and South Greenland (CitationBarrie et al., 1992), Franz Josef Land and Spitzbergen can be assumed to be more heavily affected by polluted air from midlatitudes, in particular Eurasia, than from other parts of the Arctic (CitationSimoes and Zagorodnov, 2001).
The varying exposure to pollutant-laden air masses of different arctic regions was expressed, for instance, by the higher concentrations of nss-SO4 2− found in snow at Franz Josef Land and Spitzbergen compared to Greenland or the Canadian and U.S. Arctic. While average nss-SO4 2− concentrations ranged from 5 to 10 &mu= L−1 in snow of Franz Josef Land and Spitzbergen (CitationSemb et al., 1984; CitationHodgkins and Tranter, 1998; CitationSimoes and Zagorodnov, 2001), in the interior of Alaska or on Agassiz Ice Cap nss-SO4 2− was up to 6 &mu= L−1, and very low values were generally found in Greenland snow, with concentrations less than 2 &mu= L−1 (CitationGoto-Azuma et al., 1997, CitationJaffe and Zukowski, 1993, CitationFinkel et al., 1986; CitationHerron, 1982; CitationBeer et al., 1991; CitationWolff, 1995; CitationLegrand, 1995).
Mean nitrate concentrations found in arctic snow are generally similar to Franz Josef Land values, as for Agassiz Ice Cap at Ellesmere Island, the interior of Alaska (CitationGoto-Azuma et al., 1997, CitationJaffe and Zukowski, 1993), and Greenland (e.g., CitationFinkel et al., 1986; CitationHerron, 1982; CitationBeer et al., 1991; CitationWolff, 1995; CitationLegrand, 1995), whereas on Spitzbergen, nitrate remained below Franz Josef Land concentrations (CitationSemb et al., 1984; CitationHodgkins and Tranter, 1998).
The dominance of sea salt in the chemical composition of the snowpack was also found for glaciers in Svalbard. CitationHodgkins and Tranter (1998), for instance, reported that sea salt accounted for about 98% of the snowpack solute mass at Scott Turnerbreen in central Svalbard. Moreover, mean concentrations of sodium and chloride were up to 10 times higher than those found at Franz Josef Land. In northern Alaska, CitationJaffe and Zukowski (1993) found chloride concentrations that exceeded Franz Josef Land values by a factor of 2 to 3. The influence of the sea-salt aerosol, however, generally decreases both with increasing distance from the sea and with elevation for inland ice sheets, as was demonstrated for Greenland snow by CitationHerron (1982). In Greenland, mean chloride concentrations were about 1 order of magnitude below those on Franz Josef Land, and similar low values were given for the interior of Alaska (CitationJaffe and Zukowski, 1993) and Agassiz Ice Cap, Ellesmere Island (CitationGoto-Azuma et al., 1997).
SEASONAL VARIABILITY OF ION CONCENTRATIONS
Vertical profiles of ion concentrations revealed a pronounced seasonal variability (). Concentrations were generally higher in the upper half of the snowpack, i.e., in spring and summer snow. Although the seasonal variability of ion concentrations was found at all sites, and , and support this statement, one should keep in mind the influence of wind drift on the snow-pack (see Local Variability of the Snow Cover). The chemical stratigraphy found in July was not expected to directly reflect the concentration sequence of at-the-site precipitation. Besides, the elution of ions could alter the vertical concentration profile, as we found at Wilczek Island.
Distinct ions differed in their vertical distribution within the snow cover and could be summarized in two groups: sea-salt- and non-sea-salt-derived ions. The concentration of sea-salt-derived ions such as chloride, sodium, magnesium, or sea-salt sulfate is expected to be high during times when the ocean is open, and sea-salt particles are formed by the bursting of gas bubbles at the water surface, transported by the persisting air motion into the archipelago, and removed there by wet and dry deposition. The vertical concentration profiles of chloride and sea-salt sulfate () revealed the difference of open and ice-covered seasons. This difference was particularly obvious for the snow cover at Eva-Liv and Salisbury Islands, where both chloride and sea-salt sulfate concentrations were 6 to 7 times higher in the upper part of the snowpack than in the lower section of the snow cover, which presumably corresponded to the period when the sea was ice covered. The vertical distribution of sodium, magnesium, and potassium in the snow cover closely followed the concentration profiles of chloride, which was already expressed by the high correlation between these ions ().
The vertical profiles of non-sea-salt-derived ions clearly differed from those of the sea-salt compounds. Nitrate and nss-SO4 2−, for instance, showed a concentration maximum in the upper third of the snowpack and a secondary one right below that ( and ), which may be attributed to summer and spring, respectively. Investigations of the precipitation chemistry at Dye 3, Greenland (CitationFinkel et al., 1986), indicated that anthropogenic nitrate and sulfate inputs peak in late winter and early spring, concomitant with the arctic haze, whereas the preindustrial portions tend to peak in summer or late spring. A similar result was found by CitationBeer et al. (1991) at Dye 3 in Greenland snow, with bimodal peaks of nitrate and sulfate in spring and summer to fall. Peak values of sulfate and nitrate in late winter/early spring and for nitrate again in summer were reported by CitationGoto-Azuma et al. (1997) from Ellesmere Island, Canada.
The seasonality of ion concentrations in snow can be interpreted partly as a consequence of the different atmospheric circulation patterns in winter and summer. During the cold season, trace substances and pollutants mainly from Eurasia are transported into the Arctic, where the stable stratification of the polar air leads to aerosol residence times of several weeks and only low amounts of wet and dry deposition (CitationPacyna, 1995). The major components of the arctic aerosol during winter are sulfates, whereas nitrates are largely missing. The latter finding corresponds well with the generally low nitrate concentrations, which hardly reached 1 &mu= L−1 (), found in the lower parts of the snow cover at Franz Josef Land. However, the low concentration ratio of nitrate to sulfate in the arctic winter aerosol is not directly reflected in snow, likely because of the more efficient scavenging of nitrate by snow crystals than by sulfate (CitationBarrie, 1996). This explained the fact that nss-SO4 2− concentrations measured in the lower parts of the snow cover at Franz Josef Land were similar to or only slightly exceeded the corresponding nitrate concentrations. The south-to-north transport prevailing in winter changes to a weak north-south flow during summer, and transport over the northern Atlantic and Pacific Oceans becomes more frequent. Air masses thus contain anthropogenic and natural components from a multitude of sources, which, combined with a higher deposition rate compared to winter, caused considerably higher ion concentrations in the upper part of the snowpack of Franz Josef Land. Peak values are about 1 order of magnitude above the respective winter concentrations ( and ).
The different transport regimes in winter and summer can be clearly seen from a study by CitationVinogradova (2000), who gave a detailed picture of the areas contributing to the input of anthropogenic pollutants to the Russian Arctic. Calculating 5-d backward trajectories for a 10-yr period, she found that Northern Europe, Kola Peninsula, the Norilsk area, and the Pechora Basin were the main sources for Franz Josef Land in April, whereas in July the importance of these regions as a source area for the archipelago was strongly reduced.
FRACTIONATION OF IONS
The fractionation in ion elution could be followed nicely at Salisbury Island. There the seasonal snow cover was sampled three times at the same site, once right before the onset of snowmelt and twice within the melt phase. Whereas the water equivalent of the snowpack decreased by only 10% between 7 and 21 July, mean concentrations of specific ions revealed a considerable reduction (). Sulfate, in particular, decreased in concentration by a factor of 5 to 6 within the 2-wk sampling period, and the decrease was even more pronounced for nss-SO4 2−, a measurement that was derived using sodium as a reference substance for the sea-salt fraction (). The ratio between the mean magnesium concentrations on 7 July and 21 July amounted to 3.7 and varied between 1.3 and 1.9 for other major ions. Only the mean ammonium concentration remained more or less constant. The decrease due to elution was apparently counterbalanced by the enhanced input during summer ().
In view of the spatial variability of the snow cover ( and ), one might argue that the observed decrease of ion concentrations was incidental and could not be attributed to ion elution. However, eight out of nine ions and the mean electrical conductivity of the snow cover steadily decreased between 7 and 21 July (), which ruled out a possibly artificial trend. Moreover, the decreasing ion content as a consequence of the elution of ions, a well-known and frequently observed phenomenon, was supported by the changing stratigraphy of the snow cover. On 7 July, snow temperature varied between −0.5°C at the top and −2.8 °C at the bottom of the pack, whereas it was close to 0°C throughout the entire snow cover on 12 and 21 July. Crystal forms turned from rounded and faceted particles on 7 July to clustered, rounded polycrystals and slush, both indicative of wet snow.
Concentration ratios of distinct ion pairs and their temporal variability during snowmelt may indicate some sequence of ion elution. Examples of ion ratios are given in , clearly revealing the decrease of the sulfate/nitrate ratio in the melting snowpack. The ratio of mean magnesium to sodium concentration also decreased from the sea-salt value to about 0.1, whereas the chloride/sodium ratio increased slightly. Comparison of mean concentrations of sulfate, nitrate, magnesium, sodium, chloride, and calcium at the three stages of snowmelt suggested the following elution sequence: SO4 2− > Mg2+ > Na+ > NO3 −, Cl−, Ca2+. The preferential elution of the sulfate ion compared to nitrate and chloride observed in the snowpack at Franz Josef Land was in good accordance with many other investigations and has been observed in several field and laboratory experiments (e.g., CitationJohannessen and Henriksen, 1978; CitationBrimblecombe et al., 1985; CitationBales et al., 1989; CitationGoto-Azuma et al., 1993; CitationCragin et al., 1996; CitationEichler et.al, 2000). The mobility of the magnesium ion was also found by, e.g., CitationDavies et al. (1982), CitationBrimblecombe et al. (1987), and CitationEichler et al. (2000). Generally, ion elution sequences are determined by several factors such as snow metamorphism, the extent of exclusion undergone by impurities during crystal growth, and the number of melt-freeze cycles. This combination of factors accounts for the variability in the order of elution found by different authors in their investigations, particularly in field experiments, when random conditions may vary significantly.
Summary and Conclusion
We investigated the chemical composition of the seasonal snow cover at six islands of Franz Josef Land in July 1995. The ion content of the snowpack ranged between 50 and 100 &mu= L−1 and was clearly dominated by sea salt, with Na+ and Cl− contributing to about 70% of the ion balance. Mean nitrate concentrations were about 2 &mu= L−1 and corresponded well to values found in Greenland and parts of the Canadian Arctic and Alaska. Mean total sulfate concentrations were up to 13 &mu= L−1, some 30% of which was the estimated sea-salt fraction, when sodium was used as the reference species for seawater. Nss-SO4 2− concentrations reported from different parts of the Arctic were generally exceeded by those at Franz Josef Land, with the exception of Svalbard, where reported concentrations were similar.
Ion concentrations showed a high seasonal and local variability. Local variability was also found for the water equivalent of the snow cover, which ranged between 14 and 118 cm and seemed to be strongly influenced by wind drift and redistribution of snow. Sea-salt- and non-sea-salt-derived ions differed in their seasonal variability. The concentration of sea-salt compounds was generally higher during times when the ocean was ice free. Nitrate and nss-SO4 2−, in contrast, revealed two peaks along their vertical concentration profiles. As in other investigations of arctic snow, the upper peak may be attributed to summer and the lower one to spring.
The fractionation of ions occurring during snowmelt could be followed at Salisbury Island, where one site was sampled three times within 2 wk, before the onset of snowmelt and twice during melting. As had already been shown by previous studies, sulfate was most easily released from the snowpack and experienced the most pronounced concentration decrease. Ion concentration ratios and their temporal variability revealed a certain order of elution: SO4 2− > Mg2+ > Na+ > NO3 −, Cl−, Ca2+. Since several factors affect the release of impurities from the snowpack, the mentioned sequence of ion elution gives some indication of the elution order but may vary with changing boundary conditions.
FIGURE 2. Daily mean and maximum temperature at the coastal station Krenkel, Hayes Island (), for June and July 1995 (pers. comm)
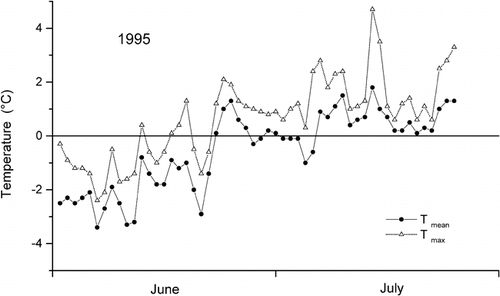
FIGURE 3. Vertical profiles of ion concentrations (nitrate, nss-sulfate, nss-calcium, ammonium) in the snow cover at six islands of Franz Josef Land, July 1995
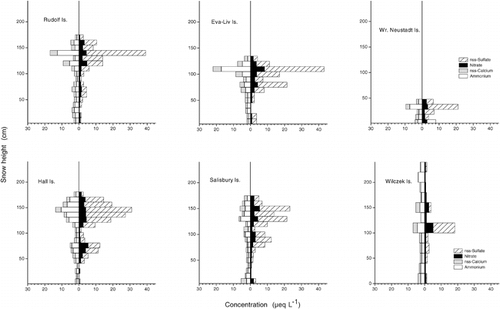
FIGURE 4. Vertical concentration profiles of chloride, ss-sulfate, and nss-sulfate in the snow cover at six islands of Franz Josef Land, July 1995
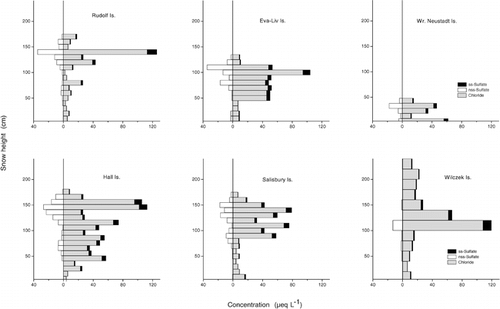
TABLE 1 Weighted mean ion concentrations and mean conductivity of the seasonal snow cover at six islands of Franz Josef Land, July 1995a
TABLE 2 Snow depth, water equivalent, and ionic loads of the seasonal snow cover at six islands of Franz Josef Land, July 1995
TABLE 3 Ion correlation matrix (correlation coefficient r) for 83 snow samples
TABLE 4 Results of the Principal Component Analysis. Factor loadings for nine variables, % of the total variance, and eigenvalues of the two factors
TABLE 5 Mean concentration of non-sea-salt sulfate (nss-SO4 2−) and its part of the total sulfate, and concentration ratio of chloride to sodium and magnesium to sodium at six sampling sites, July 1995
TABLE 6 Weighted mean ion concentrations and ionic loads of the snow cover at Salisbury Island, Franz Josef Land, between 7 July and 21 July 1995
Acknowledgments
This study was financed by Fond zur Förderung der wissenschaftlichen Forschung (FWF grant P-110 10) and the EC project ICE MASS (ENV4-CT 970490). I especially thank G. Markl for his great help during fieldwork and M. Kuhn for discussion.
References Cited
- Bales, C. R. , R. E. Davis , and D. A. Stanley . 1989. Ion elution through homogeneous snow. Water Resources Research 25:1869–1877.
- Barr, S. (ed.),. 1995. Franz Josef Land. Polarhandbok No.8. Oslo: Norsk Polarinstitutt. 175 pp.
- Barrie, L. A. 1986. Arctic air pollution: an overview of current knowledge. Atmospheric Environment 20:643–663.
- Barrie, L. A. 1996. Occurrence and trends in the arctic troposphere. In Wolff, E. W., and Bales, R. C. (eds.), Chemical exchange between the atmosphere and polar snow. NATO ASI Series 43:71–92.
- Barrie, L. A. , M. P. Olson , and K. K. Oikawa . 1989. The flux of anthropogenic sulphur into the Arctic from mid-latitudes in 1979/80. Atmospheric Environment 23:2505–2512.
- Barrie, L. A. , D. Gregor , B. Hargrave , R. Lake , R. Muir , B. Shearer , B. Tracey , and T. Bidleman . 1992. Arctic contaminants: sources, occurrence and pathways. The Science of the Total Environment 122:1–74.
- Beer, J. , R. C. Finkel , G. Bonani , H. Gäggeler , U. Görlach , P. Jacob , D. Klockow , C. C. Langway , A. Neftel , H. Oeschger , U. Schotterer , J. Schwander , U. Siegenthaler , M. Suter , D. Wagenbach , and W. Wölfli . 1991. Seasonal variations in the concentration of 10 Be, Cl−, NO3 −, H2O2, 210Pb, 3H, mineral dust and δ18O in Greenland snow. Atmospheric Environment 25A:899–904.
- Berg, T. , J. Bartnicki , J. Munthe , H. Lattila , J. Hrehoruk , and A. Mazur . 2001. Atmospheric mercury species in the European Arctic: measurements and modelling. Atmospheric Environment 35:2569–2582.
- Boutron, C. F. , J. P. Candelone , and S. Hong . 1995. Greenland snow and ice cores: unique archives of large-scale pollution of the troposphere of the Northern Hemisphere by lead and other heavy metals. The Science of the Total Environment 160: /161. 233–241.
- Brimblecombe, P. , M. Tranter , P. W. Abrahams , I. Blackwood , T. D. Davies , and C. C. Vincent . 1985. Relocation and preferential elution of acidic solute through the snowpack of a small remote high-altitude Scottish catchment. Annals of Glaciology 7:144–147.
- Brimblecombe, P. , S. L. Clegg , T. D. Davies , D. Shooter , and M. Tranter . 1987. Observations of the preferential loss of major ions from melting snow and laboratory ice. Water Research 21:1279–1286.
- Cragin, J. H. , A. D. Hewitt , and S. C. Colbeck . 1996. Grain-scale mechanisms influencing the elution of ions from snow. Atmospheric Environment 30:119–127.
- Davies, T. D. , C. E. Vincent , and P. Brimblecombe . 1982. Preferential elution of strong acids from a Norwegian ice cap. Nature 300:161–163.
- Dowdeswell, J. A. 1995. Glaciers in the High Arctic and recent environmental change. Philosophical Transactions of the Royal Society, London, Series A 352:321–334.
- Eichler, A. , M. Schwikowski , and H. W. Gäggeler . 2000. Preferential elution of chemical species in alpine firn. Annual Report 1999. Switzerland: Paul Scherrrer Institut, 44.
- Finkel, R. C. , C. C. Langway Jr. , and H. B. Clausen . 1986. Changes in precipitation chemistry at Dye 3, Greenland. Journal of Geophysical Research 91:D9 9849–9855.
- Glazovskij, A. F. 1995. Geographical position and general characteristics of the Franz Josef Land archipelago. In Barr, S. (ed.), Franz Josef Land. Oslo: Norsk Polarinstitutt, 8–10.
- Goto-Azuma, K. , M. Nakawo , M. Shimizu , N. Azuma , M. Nakayama , and K. Yokoyama . 1993. Temporal changes in chemical stratigraphy of snow cover. Annals of Glaciology 18:85–91.
- Goto-Azuma, K. , R. M. Koerner , M. Nakawo , and A. Kudo . 1997. Snow chemistry of Agassiz Ice Cap, Ellesmere Island, Northwest Territories, Canada. Annals of Glaciology 43:199–206.
- Herron, M. M. 1982. Impurity sources of F−, Cl−, NO3 − and SO4 2− in Greenland and Antarctic snow. Journal of Geophysical Research 87:C4 3052–3060.
- Hisdal, V. 1995. Climate. In Barr, S. (ed.), Franz Josef Land. Oslo: Norsk Polarinstitutt, 11–14.
- Hodgkins, R. and M. Tranter . 1998. Solute in high arctic glacier snow cover and its impact on runoff chemistry. Annals of Glaciology 26:156–160.
- Iversen, T. 1989. Numerical modelling of the long range atmospheric transport of sulphur dioxide and particulate sulphate to the Arctic. Atmospheric Environment 23:2571–2595.
- Iversen, T. 1996. Atmospheric transport pathways for the Arctic. In Wolff, E. W., and Bales, R. C. (eds.), Chemical exchange between the atmosphere and polar snow. NATO ASI Series 43:71–92.
- Jaffe, D. A. and M. D. Zukowski . 1993. Nitrate deposition to the Alaskan snow pack. Atmospheric Environment 27A:2935–2941.
- Johannessen, M. and A. Henriksen . 1978. Chemistry of snow melt water: changes in concentration during melting. Water Resources Research 14:615–619.
- Legrand, M. 1995. Sulphur-derived species in polar ice: a review. In Delmas, R.J. (ed.), Ice core studies of global biogeochemical cycles. NATO ASI Series 30:91–119.
- Longbottom, J. E. and J. J. Lichtenberg . (eds.),. EPA Test Methods 1982: Methods for Organic Chemical Analysis of Municipal and Industrial Wastewater. EPA-600/4-82-057. Washington, DC: U.S. Environmental Protection Agency.
- Macdonald, R. W. 28 coauthors,. 2000. Contaminants in the Canadian Arctic: 5 years of progress in understanding sources, occurrence and pathways. The Science of the Total Environment 254:93–234.
- Macheret, Y. Y. , A. F. Glazovsky , J. A. Dowdeswell , and M. R. Gorman . 1999. Ice cap volume change on Franz Josef Land during the last 40 years. Zeitschrift für Gletscherkunde und Glazialgeologie 35:101–116.
- Mayewski, P. W. , B. Lyons , M. J. Spencer , M. Twickler , C. F. Buck , and S. Whitlow . 1990. An ice core record of atmospheric response to anthropogenic sulfate and nitrate. Nature 346:554–556.
- Neftel, A. , J. Beer , H. Oeschger , F. Zurcher , and R. Finkel . 1985. Sulfate and nitrate concentrations in snow from South Greenland, 1895–1978. Nature 314:611–613.
- Nickus, U. and M. Kuhn . 1994. Ion chromatographic determination of anions and cations at ultra-low concentrations in alpine snow. Journal of Chromatography A 671:225–229.
- Nickus, U. , M. Kuhn , U. Baltensperger , R. Delmas , H. Gäggeler , A. Kasper , H. Kromp-Kolb , F. Maupetit , A. Novo , F. Pichlmayer , S. Preunkert , H. Puxbaum , G. Rossi , W. Schöner , M. Schwikowski , P. Seibert , M. Staudinger , V. Trockner , D. Wagenbach , and W. Winiwarter . 1997. SNOSP: Ion deposition in high alpine snow packs. Tellus 49B:56–71.
- Pacyna, J. M. 1995. The origin of arctic air pollutants: lessons learned and future research. The Science of the Total Environment 160: /161. 39–53.
- Peters, A. J. , D. J. Gregor , C. F. Teixeira , N. P. Jones , and C. Spencer . 1995. The recent depositional trend of polycyclic aromatic hydrocarbons and elemental carbon to the Agassiz Ice Cap, Ellesmere Island, Canada. The Science of the Total Environment 160: /161. 167–179.
- Pomeroy, J. W. and E. Brun . 2001. Physical Properties of Snow. In Jones, H. G., Pomeroy, J. W., Walker, D. W., and Hoham, R. W. (eds.), Snow Ecology. Cambridge: Cambridge University Press, 45–126.
- Pomeroy, J. W. and H. G. Jones . 1996. Wind-blown snow: sublimation, transport and changes to polar snow. In Wolff, E. W., and Bales, R. C. (eds.), Chemical exchange between the atmosphere and polar snow. NATO ASI Series G 43:453–489.
- Pomeroy, J. W. , T. D. Davies , and M. Tranter . 1991. The impact of blowing snow on snow chemistry. In Davies, T. D., Tranter, M., and Jones, H. G. (eds.), Seasonal snowpacks: processes of compositional change. NATO ASI Series G 28:71–114.
- Rahn, K. A. 1981. Relative importances of North America and Eurasia as sources of arctic aerosol. Atmospheric Environment 15:1447–1455.
- Riley, J. P. and G. Skirrow . 1965. Chemical Oceanography, Vol. 1. London and New York: Academic Press.
- Semb, A. , R. Braekkan , and E. Joranger . 1984. Major ions in Spitsbergen snow samples. Geophysical Research Letters 11:445–448.
- Shaw, G. E. 1995. The arctic haze phenomenon. Bulletin American Meteorological Society 76:2403–2413.
- Simoes, J. C. and V. S. Zagorodnov . 2001. The record of anthropogenic pollution in snow and ice in Svalbard, Norway. Atmospheric Environment 35:403–413.
- Smirnov, V. V. , V. F. Radinov , A. V. Savchenko , A. A. Pronin , and V. V. Kuusk . 1998. Variability in aerosol and air ion composition in the Arctic spring atmosphere. Atmospheric Research 49:163–176.
- Tartari, G. A. , A. Marchetto , and R. Mosello . 1995. Precision and linearity of inorganic analysis by ion chromatography. Journal of Chromatography A 706:21–29.
- Vinogradova, A. A. 2000. Anthropogenic pollutants in the Russian Arctic atmosphere: sources and sinks in spring and summer. Atmospheric Environment 34:5151–5160.
- Wolff, E. W. 1995. Nitrate in polar ice. In Delmas, R. J. (ed.), Ice core studies of global biogeochemical cycles. NATO ASI Series 30:195–224.