Abstract
Physical, chemical and thermal properties of five pedons in the Uinta Mountains of northeastern Utah were studied to determine how soils and microclimates vary across the ecotone between subalpine forest and dry meadow. All five profiles have developed in the same parent materials, from base upward: basal till, glaciofluvial sediment, and coarse supraglacial debris with infilled eolian silt. Temperatures were measured at the soil surface and at depths of 10 and 50 cm for 420 days. Meadow soils (Typic Humicryepts) are warmer, have Bw horizons, and contain more Ca, organic carbon, and clay in the A horizon. Forest soils (Inceptic Haplocryalfs) have Bt horizons, higher B/A horizon clay ratios, are more acidic, and contain more exchangeable Mg and total exchangeable cations than meadow soils. Although subzero temperatures were never recorded at the surface of the forest soil in the summers of 1998 and 1999, those at the surface of the meadow soil fell below 0 °C on ∼35% of the nights. Mean 4:00 a.m. temperatures in the meadow during the summer are significantly colder than those in the forest (2.2 °C vs. 5.2 °C). The meadow formed following an initial forest clearing event, possibly fire or an insect infestation. Since that time, forest encroachment has been slow because seedling establishment in the meadow is inhibited by a combination of frequent summer freezing events, moisture stresses resulting from textural discontinuities between soil parent materials, and competition between tree seedlings and meadow vegetation.
Introduction
Subalpine meadows occupy openings within otherwise closed coniferous forest at high elevations in the western U.S.A., and represent important elements of habitat diversity. The origin of these features has long intrigued ecologists because they exist despite no obvious impediments to tree encroachment. Many studies have investigated possible biological and physical factors controlling the location of the ecotone between subalpine forest and open meadow environments and its long-term persistence. Some have reported stability of this ecotone over millennial time scales. For example, CitationDoering and Reider (1992) utilized soil morphology and mapping to conclude that the boundary of a subalpine meadow in Wyoming has been stable for at least the past 2000 years. Many other studies, however, have documented migration of forest into subalpine meadows in mountainous areas (e.g. CitationPatten, 1969; CitationDunwiddie, 1977; CitationVale, 1978; CitationFisher et al., 1987; CitationJakubos and Romme, 1993; CitationMillar et al., 2004; CitationRoush et al., 2007). Meadow invasion by trees has been related to climatic amelioration following the termination of the Little Ice Age in the late 1800s (CitationFonda and Bliss, 1969; CitationFranklin et al., 1971; CitationMunroe, 2003a; CitationMillar et al., 2004), fire suppression (CitationGruell, 1983; CitationFisher et al., 1987), and changes in livestock grazing practices (CitationDunwiddie, 1977; CitationVale, 1981). Microclimatic effects, including moderation of temperature extremes adjacent to the meadow edge, have also been implicated (CitationPatten, 1969; CitationFranklin et al., 1971).
Subalpine meadows are common landscape features in the Uinta Mountains of northeastern Utah. Despite their ubiquity, however, meadows have received little study beyond basic vegetation cataloging by ecologists with the U.S. Forest Service. In the one published report, CitationOstler et al. (1982) investigated the effects of snow depth on meadow vegetation. They determined that graminoid production increased with earlier dates of snowmelt, while forb production was essentially stable. These results suggest that future climatic changes that alter the average duration of annual snow cover could impact the composition of meadow vegetation communities, and could conceivably affect the overall persistence of some meadow environments.
This study evaluated the relative importance of potentially limiting pedologic and microclimatic factors across the forest-meadow ecotone surrounding a representative subalpine meadow in the Uinta Mountains. Field and lab analyses of soil profiles, and quantitative assessment of soil thermal environments, were combined in order to: (1) identify the geologic origin of soil parent materials in the study area; (2) compare the chemical and physical properties of soils across the boundary between subalpine forest and dry meadow environments; (3) compare surface and subsurface temperature conditions across this boundary; and (4) evaluate the role of these variables in controlling the position of the forest-meadow ecotone at this site.
Methods
PHYSICAL SETTING
The 18-ha study site, informally named Whiterocks Meadow, is located in the headwaters of the Whiterocks River in the Uinta Mountains of northeastern Utah, at an elevation of ∼3200 m (). Bedrock in the area is a mixture of weakly metamorphosed, red-colored, Precambrian quartzite and shale (CitationHintze, 1980). The Uintas were extensively glaciated during the Pleistocene, and the basin in which Whiterocks Meadow is located was the accumulation area for a valley glacier that covered more than 86 km2 (CitationMunroe and Laabs, 2009). Basal radiocarbon dates from two lakes 6 km west of Whiterocks Meadow indicate that deglaciation was complete by ∼14 ka BP (CitationCorbett and Munroe, 2010). Whiterocks Meadow was selected for this study because of past soils and vegetation research conducted in the vicinity by research staff of the Ashley National Forest. The meadow has not been grazed for several decades.
Figure 1 Land-type map of the upper Whiterocks River watershed. Land types were determined through visual delineation of homogeneous polygons on a large-scale false-color infrared aerial photograph, coupled with field checking. Out of the 3400 ha mapped, 6% is dry meadow, 13% is wet meadow, 56% is continuous forest, 20% is stony upland, 2% is thinly forested stony upland, 2% is open water, and 1% is disturbed (water supply dam). The contour interval in the background is 25 meters, and Whiterocks Meadow is outlined in bold. The inset at upper right shows an enlargement of Whiterocks Meadow overlain on 10-m contours, and the locations of the five soil pits arranged along a transect from coniferous forest (Sites 1 and 2) into the center of the open meadow (Sites 3, 4, and 5). The inset at upper left shows the location of the Whiterocks River headwaters in northeastern Utah.
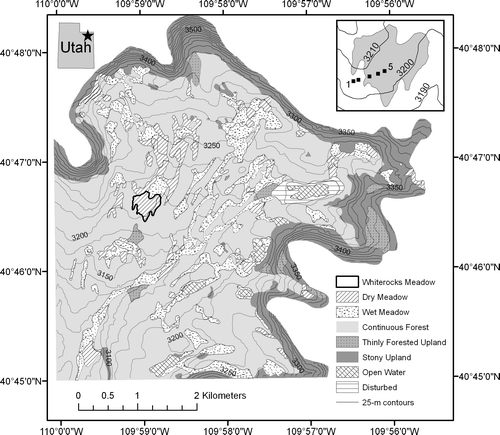
A total of 27 plant species has been reported from Whiterocks Meadow, although Danthonia intermedia (wild oat grass), Deschampsia caespitosa (tufted hairgrass), and Carex scirpodieae (single spike sedge) are dominant (). Based on two counts of 160 points, 56% of the meadow surface is vegetated, 37% is unvegetated organic litter, and 7% is bare rock (). The surrounding forest community is composed of Picea engelmannii (Engelmann spruce) and Pinus contorta (lodgepole pine) with a sparse understory of Vaccinium scoparium (grouse whortleberry).
Table 1 Plant species present in Whiterocks Meadow.*
Mean annual air temperatures at the Chepeta Snowpack Telemetry (SNOTEL) station, approximately 2 km from the meadow at an elevation of 3120 m, averaged −0.25 °C for the period 1990 through 2010. Mean summer (June/July/August) temperatures averaged 9.5 °C (July 11.4 °C) for 1990 to 2010. The 1 April water content of the snowpack during the period 1971 to 2000 ranged from 250 to 700 mm (mean of 360 mm), and the total annual precipitation ranged from 470 to 1040 mm, with a mean of 775 mm (CitationWestern Regional Climate Center, 2010).
EXPERIMENTAL DESIGN
Five sampling sites were established along a 225-m-long transect centered on 40°46′33″N, 109°59′02″W (UTM Zone 12N, 585738E 4514366N) and extending from the forest, through the forest-meadow boundary, to near the meadow center (, inset). Site 1 was located in mature forest of Picea engelmannii and Pinus contorta. Site 2 was near the edge of the continuous forest canopy, Site 3 was in the open Pinus-Picea parkland transition zone, Site 4 was in the open meadow just beyond the last Pinus seedling, and Site 5 was near the meadow center.
SOIL INVESTIGATIONS
Soil pedons at each of the five study plots were excavated and described to a depth of 100 cm according to standard methods (CitationSoil Survey Staff, 1993). Depth, thickness range, boundary characteristics, Munsell color (at field moisture), structure, texture, root size and abundance, reaction with dilute HCl, and estimated percent gravel, cobbles, and stones were recorded for each horizon. None of the profiles exhibited evidence of burrowing or other disturbance. Samples of each major horizon (n = 26) were taken for laboratory analysis of particle size distribution, and chemical properties including exchangeable Ca, Mg, Na, and K, extractable Al, cation exchange capacity (CEC), exchangeable acidity, total organic carbon (C), pH (by CaCl2 and H2O), percent base saturation (BS), and aluminum saturation. Prior to lab analyses, samples were dried at 25 °C and passed through a 2-mm sieve.
Bulk density for soil horizons was estimated from measured total C through empirical relationships derived for different textural classes (CitationAlexander, 1989, Eq. 2). Quantities of weathering products within individual horizons were calculated from the product of horizon thickness, bulk density, and the specific soil quantity, adjusted for estimated clast content. Horizon values were combined within each profile to yield profile-sums of weathering products. A density of 2.65 gm/cm3 was assumed for the quartzite clasts in the pedon mass calculations.
TEMPERATURE DATALOGGERS
Three Optic Stowaway Temp dataloggers with a temperature range of −5 to 37 °C were installed at each of the five study sites to record soil temperature at depths of 0, 10, and 50 cm. The surface loggers recorded temperature at 16-minute intervals while the subsurface loggers recorded at 96-minute intervals starting on 5 July 1998. The data from all five surface temperature loggers were downloaded on 14 September 1998, and then re-set to record at 60-minute intervals through the winter. They were downloaded again on 29 June and 29 August 1999. The subsurface loggers were exhumed and downloaded on 29 August 1999 after recording continuously since deployment. The 50-cm logger at Site 1 was installed later than the others (29 September 1998) because of a high water table at the site in early summer.
A single Optic Stowaway Temp logger with a −39 to +75 °C range recorded air temperature at 60-minute intervals between 15 July 1998 and 29 August 1999. It was positioned in a shaded location 2 m above the ground between Sites 2 and 3.
Visual comparison of the temperature time-series between loggers was supported by the production of thermoisopleth diagrams (e.g. CitationWundram et al., 2010). Thermal gradients (in degrees/cm) between the loggers at 10 and 50 cm in the same pedon were calculated from the temperature difference between simultaneous measurements and the vertical spacing between the loggers.
AGE CONTROL
Cores of 4 mature upright Pinus contorta and 3 Picea engelmannii trees present in the meadow were taken with an increment borer. Tree-rings were counted with a stage micrometer connected to a computer running Metronics Quick-Chek. In addition, a sample of wood from an in situ stump was submitted for radiocarbon dating. The stump exhibited no signs of having been cut, and it was assumed that the radiocarbon age would reflect the death date of the tree. The resulting radiocarbon age was calibrated using CALIB 6.0 (CitationStuiver et al., 1998; CitationStuiver et al., 2010).
REGIONAL CONTEXT
To evaluate the potential for extrapolating the results of this study throughout the Uinta Mountains, a land type map was generated for the headwaters of the Whiterocks River through a three-stage process. First, contiguous polygons (n = 208) were created by visually delineating homogeneous areas on a large-scale, false-color infrared image. Second, all of these polygons were field checked and classified into one of seven categories: dry meadow, wet meadow, continuous forest, thinly forested upland, stony upland, open water, and disturbed. Digitizing of the polygon network into a GIS and linking of the individual polygons to a data table containing their attribute codes permitted development of a digital land type map for the area (). The surface area of Whiterocks Meadow was determined, and the percent of the Whiterocks River headwaters region covered by similar meadows was calculated. Finally, a supervised classification was run on a composite (Bands 7, 5, 3) multispectral Landsat TM image covering the entire Uinta Mountain region (2 July 1989) to determine the total area of meadow similar to the study site elsewhere in the Uinta Mountains.
Results
EXTENT OF SUBALPINE MEADOWS IN THE UINTA MOUNTAINS
The land type map generated for the Whiterock River headwaters, along with satellite imagery of the broader Uinta Mountains region, permits analysis of the extent of features similar to the meadow studied in this project. Whiterocks Meadow has a surface area of 18 ha and a maximum slope, determined from a 30-m digital elevation model, of 7°. Of the 3378 ha mapped in the headwaters of the Whiterocks River (), 1395 ha have a slope of <7°, and dry meadows similar to Whiterocks Meadow cover 14% of this area. Expanding to the entire Uinta Mountains region, dry meadows cover 270 km2 of the land surface with a slope of <7°, also corresponding to 14%. Overall, therefore, dry meadows like Whiterocks Meadow are important and widespread components of the environment at higher elevations in the Uinta Mountains.
SOIL PARENT MATERIALS
All five pedons are developed in unconsolidated sediment and contain textural discontinuities reflecting multiple parent materials (). Large cobbles and boulders comprise 25 to 60% of the volume of the surface horizon, and are numerous on the soil surface. A dramatic decrease in coarse fragment percentage, with a concurrent increase in sand content, marks the transition to the second parent material. The mean sand content of the surface horizons is 34%, while that of the underlying second parent material is 75%, a difference that is highly significant (P < 0.001, Z = 3.62, Mann-Whitney U test). Textures of the second parent material vary among the five pedons, ranging from sand with <1% clay, to loam with 11% clay. Finally, the bottom 30 to 80 cm of all pedons is marked by an abrupt increase in silt content associated with changes in soil structure and color. Fine silt/coarse silt ratios in the lowest parent material average 1.2, compared with 0.6 for the middle parent material, a difference that is highly significant (P = 0.0073, Z = −2.44, Mann-Whitney U test).
Figure 2 Texture-depth plots for the five soil profiles. Black (left) is clay, dark gray (right) is sand, and the light gray in between represents silt. All values are in percent. The superposition of the three parent materials (loess over glaciofluvial sand over glacial till) in these soils is obvious, and the overall patterns are quite similar. However, clay content is higher under the meadow vegetation (Sites 3, 4, and 5) and B/A clay ratios are higher at the forested sites (1 and 2).
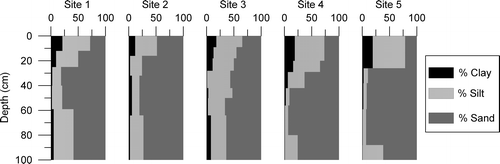
SOIL DEVELOPMENT
Soil profiles vary fundamentally with position across the forest-meadow ecotone. The horizon sequence under forest vegetation (Sites 1 and 2) is O and/or A/E/Bt while the meadow soils (Sites 3, 4, and 5) feature an A over Bw horizon (). Mean annual temperatures at 50 cm in these soils range from 1.2 to 3.8 °C (). Soils at Sites 1 and 2 have an isofrigid temperature regime (mean annual soil temperature between 0 and 8 °C, and <6 °C difference between summer and winter), while Sites 3, 4, and 5 are frigid (>6 °C seasonal difference). The soil at Site 1 has an ochric epipedon, while the others have umbric epipedons. Accordingly, the soils at Sites 1 and 2 are classified as Inceptic Haplocryalfs, while the other profiles are Typic Humicryepts (CitationSoil Survey Staff, 2010).
Figure 3 Twelve-month average temperatures for Whiterocks Meadow dataloggers at the soil surface, and depths of 10 and 50 cm. Sites 1 and 2 were located in the forest, Sites 4 and 5 in the meadow, and Site 3 in the open transition zone between the two communities. The dashed line marked the forest-meadow ecotone.
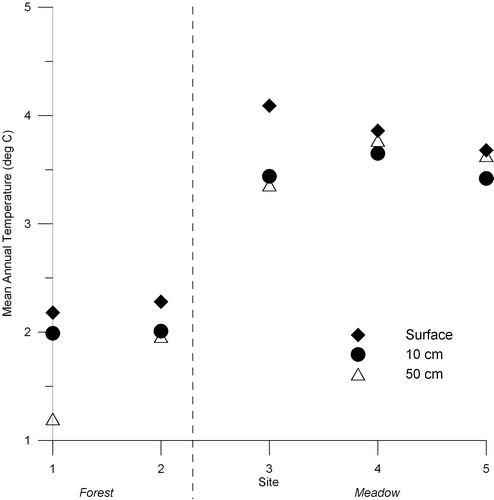
Table 2 Morphological properties of soils along the Whiterocks Meadow transect.*
The A horizons of the five pedons have silt loam, loam, or fine sandy loam textures, with 9 to 21% clay and Munsell colors from 7.5YR 2.5/2 to 10YR 2/2 (). Primary structure in the A horizons is moderately well developed, and ranges from very fine granular to medium subangular blocky. Peds are friable, and roots of all sizes are common. Total A horizon thickness is greater in the meadow, with the thickest A horizon found at Site 4.
E horizons are present in the profiles developed under coniferous vegetation (Sites 1 and 2), but are absent in the meadow soils (). The E horizon in both profiles is a loamy sand with 2% clay. E horizons hues are fairly red (2.5YR and 5YR) reflecting the color of the parent materials.
Soil B horizons are generally redder than surface horizons (hues of 2.5YR and 5YR, and maximum chroma of 6), and contain less clay and more sand (). Boundaries between A and B horizons are abrupt or clear, and either wavy or broken. Soils at Sites 1 and 2 have weak argillic horizons represented by slight increases in clay content relative to the overlying horizon, and the presence of clay skins on ped faces. Roots are less common in the B horizons, and the majority of roots were restricted to the upper 25 cm under forest vegetation, and 30 cm in the meadow.
None of the excavations encountered unaltered parent material, although the pits at Sites 3, 4, and 5 penetrated slightly altered parent material, designated by “BC” in . Hues of the lowest horizons in all profiles are 2.5YR and 5YR, reflecting the influence of the reddish bedrock. None of the horizons reacted with hydrochloric acid.
Mean horizon properties and profile quantities of weathering products were calculated for each of the pedons from the physical and chemical data presented in and . presents the mean values for the forest soils and meadow soils (excluding Site 3, the profile located at the ecotone). Total clay in the A horizon is greater in the meadow soils, as is A horizon thickness. In contrast, B horizon clay content is greater under forest vegetation, as is the B/A horizon clay ratio, indicating more effective illuviation. Profile exchangeable Ca is somewhat greater in the meadow, as is the amount of organic carbon. Exchangeable Mg, acidity, and cations, along with extractable Al, are all greater in the forest. Mean profile pH is variable, and no trend is apparent in total profile quantities of exchangeable K or in total exchangeable bases. Finally, pedon mass is essentially invariant between the five study sites.
Table 3 Chemical properties of soils along the Whiterocks Meadow transect.
Table 4 Comparison of horizon properties and profile quantities of weathering products.
AIR TEMPERATURE
The maximum air temperature during the observation period was 20.8 °C, while the minimum was −28.5 °C. The overall mean for the period of record was −1.5 °C. The mean summer (June/July/August) temperature in 1999 was 8.7 °C, while the mean July temperature was 10.9 °C. To compare these measurements with typical conditions in Whiterocks Meadow, the mean temperatures for each day of the record were regressed on those recorded for the same time interval at the Chepeta SNOTEL station located 2 km away. Conditions are extremely comparable between the two sites (r2 = 0.98). Regressions for daily minimum and daily maximum temperatures are similarly robust (r2 = 0.93). Overall the summer of 1999 at the Chepeta SNOTEL did not differ significantly from the period of record (1990–2010) mean at that station; the mean summer temperature (8.7 °C) was 0.8 °C cooler than the mean of 9.5 °C (s = 1.2 °C), and July (11 °C) was 0.4 °C cooler than the mean of 11.4 °C (s = 1.5 °C). Given the strength of the correlation between the Whiterocks study site and the Chepeta SNOTEL station, therefore, the summer of 1999 in Whiterocks Meadow can be considered typical for the period of record.
SOIL TEMPERATURES
The results from the dataloggers at the five study sites provide considerable detail about the soil thermal regime across the forest-meadow ecotone. Mean temperatures at all depths (0, 10, and 50 cm) are warmer in the meadow compared with the forest ( and ). The mean surface temperatures for Sites 3, 4, and 5 shown in are likely underestimates because these loggers routinely reached their maximum temperature threshold (37 °C) on sunny afternoons. The mean annual surface temperature at Site 3 is slightly warmer (∼0.3 °C) than Sites 4 and 5 because the latter two sites are located at somewhat lower elevations (<5 m difference) where cool air collects during still summer nights. also illustrates that the mean temperature of the 50-cm (bottom) logger was greater than the 10-cm (middle) temperature at the two sites in the meadow (4 and 5). This result indicates that the intermediate depths of the meadow soil are affected more by winter cold than the 50-cm depth, but are not warmed as much by summer sun as the surface. This situation likely reflects a thinner, less insulating, snow cover in the meadow compared to the forest.
Table 5 Summary statistics for the 15 soil temperature dataloggers.
The number of summer (June/July/August) freezing events at the soil surface varied greatly between the meadow and forest sites. The surface loggers in the forest (Sites 1 and 2) never recorded a summer air temperature below 0 °C in 1998 or 1999. In contrast, the loggers in the meadow (Sites 3, 4, and 5) recorded freezing temperatures about one out of every three nights (38% in 1998 and 31% in 1999), with Site 4 recording temperatures below freezing more than half of the nights (56%) during the summer of 1998. During the summer of 1999, the air temperature control logger recorded subzero temperatures only four nights, while the temperature at the meadow surface dropped below 0 °C 16 (Site 5) to 30 (Site 4) times.
To further compare the climate of the study sites, the mean 4:00 a.m. temperature at the surface was determined for the period 5 July to 29 August for 1998 and 1999. The lowest temperatures occurred at Site 4, mirroring the frequency of freezing events. The mean of all 4:00 a.m. forest temperatures in both years during this period was 5.2 °C, while meadow temperatures averaged 2.2 °C (F-statistic = 156, P < 0.01, single factor ANOVA) indicating that the air temperature at the soil surface in the open meadow is significantly colder than that in the forest during growing season nights.
On the basis of the temperature data from the surface loggers, continuous snow cover is inferred to have begun at all five sites on 10 October 1998 when diurnal temperature fluctuations disappeared. Diurnal fluctuations returned to the meadow sites ∼7 days earlier than to Sites 1 and 2 suggesting that the meadow was snow-free about a week before the forest (). The duration of snow cover ranged from 236 to 239 days in the meadow, compared with 243 to 246 days in the forest (). For comparison, the duration of the annual snow cover ranged from 184 to 269 days (mean of 222 days) at the forested Chepeta SNOTEL station during the period 1981–1999 (CitationWestern Regional Climate Center, 2010).
Thermoisopleth diagrams presenting the full temperature time-series from the 10 subsurface loggers () illustrate that winter temperatures at 10 and 50 cm hovered around freezing despite the subzero temperatures recorded by the air temperature logger (absolute minimum of −28.5 °C, with 82% of the hourly measurements during the snow-covered period below 0 °C). This contrast underscores the efficacy of the snowpack at insulating the ground and leaves open the possibility that meltwater produced at the base of the snowpack could slowly percolate through these soils all winter long. An isothermal period during which the temperature was constant is also visible in these diagrams (). The isothermal temperature was between 0.0 and −0.1 °C for the loggers at 10 cm, and 0.0 to 0.3 °C for the deeper loggers (). This situation likely reflects saturation of the soil with meltwater derived from the snowpack surface as the sun becomes more intense in late spring. Evidence for snowpack ripening is also seen in the surface loggers, which record isothermal conditions in late spring immediately preceding loss of the thermally buffered water-ice mixture at the base of the snowpack.
Figure 4 Thermoisopleth diagrams illustrating temperature time-series at 10 and 50 cm below the soil surface at the five study sites. The y-axis on all plots is in hours. The 50-cm logger at Site 1 was deployed later than the others. Stippled pattern marks temperatures near 0 °C. All loggers remained isothermal at, or just below, freezing for several months during the winter. This isothermal period ended nearly simultaneously at the shallow and deep loggers in the open meadow sites (3, 4, and 5), but the 10-cm logger warmed appreciably before the deep logger under the closed forest canopy. Daily maximum temperatures attained by the 10-cm loggers are much greater beneath the open meadow. All dates recorded as mm/d/yy.
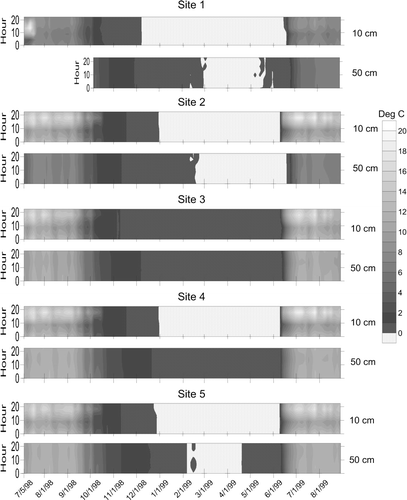
Thermal gradients [(T10−T50)/cm] between the 10- and 50-cm loggers during the snow-free period were positive except for a few hours each morning centered on 8:00 a.m. when the overnight cold wave reached the depth of the 10-cm logger. This effect was noted at all sites, but is more pronounced in the meadow soils, reflecting their lower overnight surface temperatures. Open meadow sites (4 and 5) experience this effect every night of the record. At Site 3 the inversion is less dramatic in the fall and absent in spring. Site 2 under the forest vegetation exhibits the most subdued version of this inversion, and in summer it does not happen at all. All sites are inverted all winter long, with the 50-cm logger ∼2 °C warmer than the logger at 10 cm, and all sites exhibit a dramatic single-day end to the winter inversion occurring between 30 May (Site 3) and 21 June (Site 2), roughly 6 days after release of the surface logger from snow cover.
AGE CONTROL
On the basis of the tree-ring counting, the largest of the scattered living trees in the meadow date to the early 1900s, with the oldest tree germinating in a.d. 1901. In contrast, the sample cut from the stump returned a radiocarbon age of 650 ± 50 years (Beta-146449), which calibrates to between a.d. 1275 and 1400 (at 2σ).
Discussion
SOIL PARENT MATERIALS
All five pedons are developed in a laterally continuous sequence of superposed parent materials. The large quartzite stones common in the surface layers are interpreted as coarse supraglacial sediment deposited during final meltout of the latest Pleistocene glacier in Whiterocks Basin (CitationBryant, 1992; CitationMunroe and Laabs, 2009). The space between the stones is infilled by fine-grained sediment interpreted as loess that accumulated during the Holocene. This interpretations is based on the greater average silt content of A horizons (46%) compared with subsurface horizons (22%), which is clearly visible in , as well as investigations that have revealed the presence of an eolian silt cap on soils in the eastern Uinta Mountains (CitationBockheim and Koerner, 1997; CitationBockheim et al., 2000; CitationMunroe, 2007) and the Colorado Rockies (CitationLitaor, 1987; CitationMuhs and Benedict, 2006). The well-sorted sand underneath the supraglacial sediment is interpreted as a glaciofluvial deposit (). No sedimentary structures were visible in this unit, but the uniformly high sand content (50 to 93%) is best explained by fluvial deposition. Finally, the dense silt-rich diamicton encountered near the bottom of all five profiles is interpreted as basal till. The sequence of sedimentary events, therefore, includes: deposition of basal till under active glacial ice; subsequent accumulation of subglacial glaciofluvial sediment; deposition of boulder-rich supraglacial sediment during final ice wastage; and post-glacial deposition of a loess cap that partially buried and infilled the supraglacial deposit.
SOIL DEVELOPMENT
The soil-forming factors of parent material, time, and topography are similar at all five sites. Microclimate does vary slightly between the sites, with meadow soils experiencing generally drier conditions and warmer temperatures in the upper solum. However, the development of these soils is primarily a function of vegetation, and the soils studied comprise a biosequence. Coniferous vegetation, such as at Sites 1 and 2, produces organic acids that facilitate the leaching of soluble ions from the near-surface horizons and downward translocation of clays (CitationUgolini et al., 1990). Deposition of clay and iron in the B horizon produces an argillic horizon, and changes in color (CitationSoil Survey Staff, 2010). In contrast, meadow vegetation (Sites 3, 4, and 5) is characterized by dense roots that produce a thicker A horizon with high amounts of organic carbon. Argillic horizon formation is inhibited under meadow vegetation, perhaps due to higher Ca content in the meadow A horizons (), and Bw horizons are only modestly developed in the meadow soils.
The soil properties suggest that pedogenesis at Sites 1 and 2 is converting a former meadow soil to a forest soil. Enhanced clay translocation in the forest soils is illustrated by fairly thick (41 and 32 cm) argillic horizons featuring clay skins (), and much higher B to A horizon clay ratios (). In contrast, subsoil (Bw) horizon development in the meadow soil profiles is limited when compared with the reddish hues of the parent material. Profile sums of organic carbon and Ca are greater in the meadow soils, but these amounts can change rapidly, building up under the influence of meadow vegetation, and dissipating under the acidic litter produced by conifers. Evidence of this may be seen in the profile at Site 2, which contains a weak umbric epipedon, possibly reflecting the lingering effects of the meadow vegetation that occupied this site before limited forest expansion. Finally, the forest soils are more acidic, and extractable Al values drop from 304 kmol/ha at Site 1, to 152 kmol/ha at Site 2, to less than 100 kmol/ha in the meadow, trends consistent with a forest encroaching into a meadow.
SOIL TEMPERATURES
The dramatic differences between the temperature regimes of the soils across this ecotone are primarily a result of the vegetation communities established on these soils. However these temperature differences also exert a reinforcing influence on the vegetation in several ways. First, surface temperatures are more variable in the open meadow, with extreme daily highs, and frequent summer freezes. CitationBallard (1972) reported similar results in a study of subalpine soil temperatures in British Columbia where temperatures >49 °C were reached at the surface of meadow soils during the summer months, and bare ground and meadow sites had considerably greater diurnal temperature amplitudes when not covered by snow. These observations are notable because extreme temperature fluctuations have proven fatal to Picea engelmannii seedlings in previous studies (CitationPatten, 1963; CitationHellmers et al., 1970; CitationNoble and Alexander, 1977; CitationAlexander and Shepherd, 1990; CitationWoodward et al., 1995).
Second, meadow soils also experience a shorter duration of snow cover, allowing for a slightly longer growing season. This characteristic also allows meadow soils to dry quicker in the spring, while saturated conditions persist under the forest canopy several weeks longer.
Finally, subsurface soil temperatures are dependent on depth. While mean annual soil temperatures at 50-cm are similar among the five sites, temperatures at the 10-cm depth are influenced more by air temperature, with mean temperatures in the meadow soils less than those at 50 cm (). Meadow soils also experience a seasonal temperature range of ∼11 °C compared with 7 °C for the forest soils (). Intermediate levels of the meadow soils warm faster in the spring, therefore, and reach higher temperatures during the growing season, properties that are important for plant growth and rhizome production.
MEADOW AGE
The isolated trees cored in the meadow lack fire scars that could be attributed to a forest-clearing fire. Moreover, charcoal was not found in any of the soil pits or on the meadow surface. These observations suggest that the isolated trees germinated in favorable locations within the meadow, rather than surviving from a forest that existed before the meadow formed. Therefore, the meadow must be older than the trees, all of which germinated after a.d. 1901.
Scattered stumps near the modern meadow edge, one of which was radiocarbon dated to a.d. 1275 to 1400, provide a further age constraint. The stumps represent trees that grew during a period when the forest was slightly expanded into the meadow, but their concentration near the meadow border argues against a period of total forest cover. Together, this evidence suggests that the meadow has existed with slightly varying dimensions for at least 600 years, which is consistent with the amount of time necessary to develop the organic-enriched umbric epipedons in the soil profiles in the open meadow and near the forest boundary at Site 2 (CitationBirkeland, 1984).
MEADOW ORIGIN AND STABILITY
Because all five pedons are developed in the same parent materials, lateral variability in parent material properties cannot be considered a primary control on the location of the forest-meadow ecotone. Furthermore, the presence of both Picea engelmannii and Pinus contorta above the elevation of the study site elsewhere in the Whiterocks basin suggests that the meadow could support continuous forest. Therefore, the meadow represents an interruption in the forest that was likely caused by disturbance at some point in the past. This disturbance may have been a catastrophic fire, although destructive fires are rare in these relatively moist high-elevation forests (S. Goodrich, Ashley National Forest, personal communication). Alternatively, a devastating outbreak of insects or parasites might have locally decimated the forest, creating a clearing in which meadow vegetation took hold. Either way, the disturbance must have occurred long enough ago that charred wood and/or deadfall from the former forest have completely decayed away. Both fires and infestations would have been encouraged by a drier climate. Pollen evidence from a lake on the north slope of the Uintas, ∼10 km from Whiterocks Meadow, indicates that conditions were warmer-than-modern from 5.3 ka BP through 0.6 ka BP, with the peak of warmth before 2.1 ka BP (CitationCarrara et al., 1985). Sediment cores from two lakes 6 km west of Whiterocks Meadow contain evidence of low water levels between 4 and 2.7 ka BP (CitationCorbett and Munroe, 2010) and pollen evidence from another site on the north slope, ∼35 km northwest of Whiterocks Meadow, also indicates warmer and drier conditions during the middle Holocene (CitationMunroe, 2003b). Therefore, the initial forest opening in which Whiterocks Meadow became established may have formed a few millennia ago, with the extent of trees in the meadow since that time limited to isolated individuals.
Once meadow vegetation became established in the clearing, three main factors combined to preclude conifer seedlings from broadly colonizing the meadow. First, the climate of the open meadow is hostile to conifer seedlings. The frequency of subzero growing season temperatures, documented by the dataloggers and related to radiative cooling both of the ground surface and near-ground air during clear summer nights, is particularly important. CitationSchauer et al. (1998) concluded that extreme variability of air temperatures at the ground surface contributed to meadow persistence in northwestern Colorado, and growing season temperature is commonly considered a control on the position of alpine treeline worldwide (CitationKörner, 1998). In addition, Picea seedlings grow best in at least partial shade, a requirement that is not met in the open meadow (CitationAlexander and Shepherd, 1990).
Second, soils containing prominent textural discontinuities are susceptible to soil drought (CitationBrady and Weil, 1996). Fine-textured surface horizons must be completely saturated before water can move past the discontinuity into the coarser subsoil. CitationDoering and Reider (1992) invoked this mechanism to explain the continued existence of Cinnabar Park in the Medicine Bow Mountains of Wyoming. In the Whiterocks Meadow soils, the textural contrast between fines in the surface horizon (derived from loess) and the subsurface glaciofluvial sand is particularly striking (, ). The availability of water at the rooting depth for forest vegetation during the growing season is limited by this discontinuity, whereas the moisture content of the overlying fine-textured A horizon remains sufficient for growth of grasses.
Competition between grasses and tree seedlings is a third factor likely contributing to the exclusion of conifers from the meadow. Picea engelmannii and Pinus contorta seeds perform best when they germinate in mineral soil, and can be out-competed by more opportunistic grass species (CitationDunnewald, 1934; CitationStahelin, 1943; CitationRoe et al., 1970; CitationLotan and Critchfield, 1990).
Taken together, multiple mechanisms appear to operate collectively to inhibit substantial recruitment of new seedlings in Whiterocks Meadow. The isolated mature trees that were cored in the open meadow probably represent the fortunate seeds that landed in fortuitous, but anomalous, areas of bare mineral soil during years of reduced summer freezing frequency.
Despite these obstacles, juvenile trees are present along the meadow perimeter, probably because the shade provided by trees at the ecotone makes the edge of the meadow slightly more mesic (CitationPatten, 1969; CitationFranklin et al., 1971). Similar mechanisms enable the migration of tree islands in the alpine zone (CitationHoltmeier and Broll, 1992; Citationvan Miegroet et al., 2000). The thermal inertia and insulating properties of the trees serves to dampen the amplitude of diurnal temperature fluctuations and reduces the frequency of subzero summer nights. The slightly earlier release from snow cover of sites at the meadow edge may also assist the establishment of tree seedlings (CitationPatten, 1963). Finally, due to downwind dilution of seeds during dispersal, the flux of seeds should be higher closer the forest-meadow ecotone. This higher rate of seed delivery ensures that any local exposures of mineral soil, or summers with temperatures favoring seedling survival, will be exploited, resulting in slow migration of trees into the meadow. This long-term effect of this process may be seen in the soil profiles at Sites 1 and 2, which illustrate overprinting of a forest soil on a profile developed beneath meadow vegetation. However, the apparent persistence of the meadow for centuries indicates that this encroachment process has historically operated quite slowly.
Conclusions
Physical, chemical and thermal properties of five soil pedons were studied across the ecotone surrounding a representative dry meadow in the Uinta Mountains. Similar meadows cover 14% of the gently sloping land at higher elevations, thus these forest openings are important components of the subalpine habitat mosaic. Soils under both vegetation types are developed in the same three superposed parent materials: basal till, glaciofluvial sand, and supraglacial sediment with infilled loess. Parent material properties do not control the position of the ecotone. Meadow soils are warmer, and contain more Ca, organic carbon, and A horizon clay. Epipedons are umbric, and weathering results in cambic horizons. In contrast, forest soils exhibit evidence of clay translocation, are more acidic, and contain more Mg and total exchangeable cations. Epipedons in the forest soils are ochric or marginally umbric, and argillic horizons are present at depth.
Air temperatures drop below freezing approximately one out of every three nights at the meadow surface during the growing season, but never fall below 0 °C in the forest. Mean 4:00 a.m. temperatures during the summer are significantly warmer under forest vegetation. Continuous snow cover ends a week earlier in the meadow than in the forest, and all soils remain at ∼0 °C through the winter under an insulating snow blanket (CitationJones, 1999).
Whiterocks Meadow likely originated following an initial disturbance, possibly a destructive fire or insect infestation, which locally eliminated forest cover. Once meadow vegetation became established, tree seedlings were excluded from all but the meadow perimeter by a combination of frequent summer freezing events, moisture stresses resulting from textural discontinuities between soil parent materials, and competition between tree seedlings and grasses. Slow advance of trees into the meadow is generally possible only at the meadow edge, where the presence of trees in the adjacent forest produces more temperate and mesic conditions.
The results of this study demonstrate the complex nature of the physical and biotic processes that combine to govern the distribution of plants across ecotones. A single process does not control the position of the boundary surrounding Whiterocks Meadow. Instead, soil properties, microclimates, moisture availability, and seedling mortality combine to produce a dynamic boundary that is in quasi-equilibrium with current conditions. External forces affecting this equilibrium (i.e. climate changes, insect infestation) can induce response of the ecotone, either through tree mortality, leading to meadow expansion, or seedling recruitment, leading to forest encroachment and meadow diminution (e.g. CitationRochefort and Peterson, 1996; CitationHessl and Baker, 1997).
Future climate changes related to greenhouse gas emissions may produce warmer growing season temperatures at the elevations of subalpine meadows in the Uinta Mountains and elsewhere in the western U.S.A., reducing the frequency of summer freezing events that currently inhibit forest encroachment (CitationKarl et al., 2009). Climate warming over the past several decades has been implicated in changes in forest structure at high elevations, including expansion into meadows, noted in various high-elevation locations around the western U.S.A. (e.g. CitationRochefort and Peterson, 1996; CitationDyer and Moffett, 1999; CitationMillar et al., 2004) and from elsewhere in the Whiterocks River headwaters (CitationSiderius, 2004). Because meadows represent important grazing grounds for ungulates, this result could have important consequences for the distribution and density of elk herds in the western U.S.A. On the other hand, climate warming might make forests more susceptible to stand-clearing fires and disease, leading to meadow expansion at the expense of trees (e.g. CitationWesterling et al., 2006; Citationvan Mantgem et al., 2009). Further study involving both field data and climate models is necessary to better predict future behavior of the forest-meadow ecotone in high-elevation ecosystems and to permit downscaling of global climate predictions to relevant local scales (e.g. CitationTrivedi et al., 2008).
Acknowledgments
The Ashley National Forest provided many of the dataloggers. J. Duque, D. Douglass, and S. Munroe assisted in the field. D. Hammer at the University of Missouri performed the soil chemistry analyses. The University of Wisconsin–Madison Department of Geology & Geophysics, the Geological Society of America, and the Ashley National Forest provided financial support. J. Bockheim and P. Birkeland provided constructive comments on an early draft of this manuscript.
References Cited
- Alexander, E. B. 1989. Bulk density equations for southern Alaska soils. Canadian Journal of Soil Science 69:177–180.
- Alexander, R. R. and W. D. Shepherd . 1990. Engelmann spruce. In Burns, R. M. and B. H. Honkala . (eds.). Silvics of North America: 1. Conifers; 2. Hardwoods. Washington, DC U.S. Department of Agriculture, Forest Service, Agriculture Handbook 654, vol. 2.
- Ballard, T. M. 1972. Subalpine soil temperature regimes in southwestern British Columbia. Arctic and Alpine Research 4:139–146.
- Birkeland, P. W. 1984. Soils and Geomorphology. New York Oxford University Press. 372 pp.
- Bockheim, J. G. and D. Koerner . 1997. Pedogenesis in alpine ecosystems of the eastern Uinta Mountains, Utah. Arctic and Alpine Research 29:164–172.
- Bockheim, J. G. , J. S. Munroe , D. C. Douglass , and D. Koerner . 2000. Soil development along an elevational gradient in the southeastern Uinta Mountains, Utah, USA. Catena 39:169–185.
- Brady, N. C. and R. R. Weil . 1996. The Nature and Properties of Soils. New York Prentice Hall. 639 pp.
- Bryant, B. 1992. Geologic and structure maps of the Salt Lake City 1° × 2° quadrangle, Utah and Wyoming. United States Geological Survey Miscellaneous Investigations Series Map I-1997, 1:125,000 scale.
- Carrara, P. E. , S. K. Short , and R. R. Shroba . 1985. A pollen study of Holocene peat and lake sediments, Leidy Peak area, Uinta Mountains, Utah. Geology Studies 32:1–7.
- Corbett, L. B. and J. S. Munroe . 2010. Investigating the influence of hydrogeomorphic setting on the response of lake sedimentation to climatic changes in the Uinta Mountains, Utah, USA. Journal of Paleolimnology 44:311–325.
- Doering, W. R. and R. G. Reider . 1992. Soils of Cinnabar Park, Medicine Bow Mountains, Wyoming, USA: indicators of park origin and persistence. Arctic and Alpine Research 24:27–39.
- Dunnewald, T. J. 1934. Soil factors in germination of pine seeds. University of Wyoming Publications in Science–Botany 1:267–274.
- Dunwiddie, P. W. 1977. Recent tree invasion of subalpine meadows in the Wind River Mountains, Wyoming. Arctic and Alpine Research 9:393–399.
- Dyer, J. M. and K. E. Moffett . 1999. Meadow invasion from high-elevation spruce-fir forest in south-central New Mexico. The Southwestern Naturalist 44:444–456.
- Fisher, R. F. , M. J. Jenkins , and W. F. Fisher . 1987. Fire and the prairie-forest mosaic of Devils Tower National Monument. American Midland Naturalist 117:250–257.
- Fonda, R. W. and L. C. Bliss . 1969. Forest vegetation of the montane and subalpine zones, Olympic Mountains, Washington. Ecological Monographs 39:271–301.
- Franklin, J. F. , W. H. Moir , G. W. Douglas , and C. Wiberg . 1971. Invasion of subalpine meadows by trees in the Cascade Range. Arctic and Alpine Research 3:215–224.
- Gruell, G. E. 1983. Fire and vegetative trends in the northern Rockies: interpretations from 1871–1982 photographs. Ogden, Utah U.S. Department of Agriculture, Forest Service, Intermountain Research Station, General Technical Report INT-158.
- Hellmers, H. , M. K. Genthe , and F. Ronco . 1970. Temperature affects growth and development of Engelmann spruce. Forest Science 16:447–452.
- Hessl, A. E. and W. L. Baker . 1997. Spruce and fir regeneration and climate in the forest-tundra ecotone of Rocky Mountain National Park, Colorado, U.S.A. Arctic and Alpine Research 29:173–183.
- Hintze, L. F. 1980. Geologic map of Utah. Salt Lake City Utah Geological and Mineral Survey, 1:500,000 scale.
- Holtmeier, F. K. and G. Broll . 1992. The influence of tree islands and microtopography on pedoecological conditions in the forest-alpine tundra ecosystem on Niwot Ridge, Colorado Front Range, U.S.A. Arctic and Alpine Research 24:216–228.
- Jakubos, B. and W. Romme . 1993. Invasion of subalpine meadows by lodgepole pine in Yellowstone National Park, Wyoming. USA. Arctic and Alpine Research 25:382–390.
- Jones, H. G. 1999. The ecology of snow-covered systems: a brief overview of nutrient cycling and life in the cold. Hydrological Processes 13:2135–2147.
- Karl, T. R. , M. Melillo , and T. C. Peterson . (eds.). 2009. Global Climate Change Impacts in the United States. New York Cambridge University Press. 188 pp.
- Körner, C. 1998. A re-assessment of high elevation treeline positions and their explanation. Oecologia 115:445–459.
- Litaor, M. I. 1987. The influence of eolian dust on the genesis of alpine soils in the Front Range, Colorado. Soil Science Society of America Journal 51:142–147.
- Lotan, E. J. and W. B. Critchfield . 1990. Lodgepole pine. In Burns, R. M. and B. H. Honkala . (eds.). Silvics of North America: 1. Conifers; 2. Hardwoods. Washington, DC U.S. Department of Agriculture, Forest Service, Agriculture Handbook 654, vol. 2.
- Millar, C. I. , R. D. Westfall , D. L. Delany , J. C. King , and L. J. Graumlich . 2004. Response of subalpine conifers in the Sierra Nevada, California, U.S.A. to 20th-century warming and decadal climate variability. Arctic, Antarctic, and Alpine Research 36:181–200.
- Muhs, D. R. and J. B. Benedict . 2006. Eolian additions to Late Quaternary alpine soils, Indian Peaks Wilderness Area, Colorado Front Range. Arctic, Antarctic, and Alpine Research 38:120–130.
- Munroe, J. S. 2003a. Estimates of Little Ice Age climate inferred through historical rephotography, northern Uinta Mountains, U.S.A. Arctic, Antarctic, and Alpine Research 35:489–498.
- Munroe, J. S. 2003b. Holocene timberline and palaeoclimate of the northern Uinta Mountains, northeastern Utah, U.S.A. The Holocene 13:175–185.
- Munroe, J. S. 2007. Properties of alpine soils associated with well-developed sorted polygons in the Uinta Mountains, Utah, U.S.A. Arctic, Antarctic, and Alpine Research 39:578–591.
- Munroe, J. S. and B. J. C. Laabs . 2009. Glacial geologic map of the Uinta Mountains area, Utah and Wyoming. Utah Geological Survey, Miscellaneous Publication 09-4DM, scale 1:100,000.
- Noble, D. L. and R. R. Alexander . 1977. Environmental factors affecting natural regeneration of Engelmann spruce in the central Rocky Mountains. Forest Science 23:420–429.
- Ostler, W. K. , K. T. Harper , K. B. McKnigh , and D. C. Anderson . 1982. The effects of increasing snowpack on a subalpine meadow in the Uinta Mountains, Utah, USA. Arctic and Alpine Research 14:203–214.
- Patten, D. T. 1963. Light and temperature influence on Engelmann spruce seed germination and subalpine forest advance. Ecology 44:817–818.
- Patten, D. T. 1969. Succession from sagebrush to mixed conifer forest in the northern Rocky Mountains. American Midland Naturalist 82:229–240.
- Rochefort, R. M. and D. L. Peterson . 1996. Temporal and spatial distribution of trees in subalpine meadows of Mount Rainier National Park, Washington, U.S.A. Arctic and Alpine Research 28:52–59.
- Roe, A. L. , R. R. Alexander , and M. D. Andrews . 1970. Engelmann spruce regeneration practices in the Rocky Mountains. Washington, DC U.S. Department of Agriculture, Forest Service, Products Research Report 115.
- Roush, W. , J. S. Munroe , and D. B. Fagre . 2007. Development of a spatial analysis method using ground-based repeat photography to detect changes in the alpine treeline ecotone, Glacier National Park, Montana, U.S.A. Arctic, Antarctic, and Alpine Research 39:297–308.
- Schauer, A. J. , B. K. Wade , and J. B. Sowell . 1998. Persistence of subalpine forest-meadow ecotones in the Gunnison Basin, Colorado. Great Basin Naturalist 58:273–281.
- Siderius, J. R. 2004. An investigation of alpine treeline in the Uinta Mountains, northeastern Utah, U.S.A. using age establishment analysis. M.S. thesis, University of Utah, Salt Lake City. 73 pp.
- Soil Survey Staff 1993. Soil Survey Manual. Washington DC U.S. Department of Agriculture, Handbook No. 18.
- Soil Survey Staff 2010. Keys to Soil Taxonomy. 11th edition. Washington, DC U.S. Department of Agriculture, Natural Resources Conservation Service.
- Stahelin, R. 1943. Factors influencing the natural restocking of high altitude burns by coniferous trees in the central Rocky Mountains. Ecology 24:19–30.
- Stuiver, M. , P. J. Reimer , E. Bard , W. E. Beck , G. S. Burr , K. A. Hughen , B. Kromer , F. G. McCormac , J. v.d. Plicht , and M. Spurk . 1998. INTCAL98 radiocarbon age calibration 0–24,000 BP. Radiocarbon 40:1041–1083.
- Stuiver, M. , P. J. Reimer , and R. W. Reimer . 2010. CALIB 6.0. Available at <http://www.calib.org>.
- Trivedi, M. R. , P. M. Berry , M. D. Morecroft , and T. P. Dawson . 2008. Spatial scale affects bioclimatic model projections of climate change impacts on mountain plants. Global Change Biology 14:1089–1103.
- Ugolini, F. C. , R. A. Dahlgren , and K. A. Vogt . 1990. The genesis of Spodosols and the role of vegetation in the Cascade Range of Washington, USA. In Kimble, J. and R. Yeck . (eds.). Proceedings of the ICOMOD-ISCOM workshop. New York Academic Press. 370–380.
- Vale, T. R. 1978. Tree invasion of Cinnabar Park in Wyoming. American Midland Naturalist 100:277–284.
- Vale, T. R. 1981. Tree invasion of montane meadows in Oregon. American Midland Naturalist 105:61–69.
- Van Mantgem, P. J. , N. L. Stephenson , J. C. Byrne , L. D. Daniels , J. F. Franklin , P. Z. Fulé , M. E. Harmon , A. J. Larson , J. M. Smith , A. H. Taylor , and T. T. Veblen . 2009. Widespread increase of tree mortality rates in the western United States. Science 323:521–524.
- Van Miegroet, H. V. , M. T. Hysell , and A. D. Johnson . 2000. Soil microclimate and chemistry of spruce-fir tree islands in northern Utah. Soil Science Society of America Journal 64:1515–1525.
- Westerling, A. L. , H. G. Hidalgo , D. R. Cayan , and T. W. Swetnam . 2006. Warming and earlier spring increase western U.S. forest wildfire activity. Science 313:940–943.
- Western Regional Climate Center 2010. <http://www.wcc.nrcs.usda.gov/snow/>.
- Woodward, A. , E. G. Schreiner , and D. G. Silsbee . 1995. Climate, geography, and tree establishment in subalpine meadows of the Olympic Mountains, Washington, U.S.A. Arctic and Alpine Research 27:217–225.
- Wundram, D. , R. Pape , and J. Löffler . 2010. Alpine temperature variability at multiple scales. Arctic, Antarctic, and Alpine Research 42:117–128.