Abstract
Due to shallow depth and high surface area—to—volume ratio, ponds of the Hudson Bay Lowlands are vulnerable to climatic and hydrological changes, but relations between hydrological processes and limnological conditions remain unknown. Here, we measured water balance and limnological variables (water chemistry, suspended sediments, chlorophyll-a) at 20 ponds near Churchill (Manitoba) three times during the ice-free season of 2010 to explore relations among hydrological connectivity, basin morphometry, and waterchemistry variations. Using principal components analysis, we identified that the ponds followed one of four distinctive “seasonal water chemistry trajectories” (SWCT1–4). Most of the ponds that lacked apparent hydrologic connectivity displayed SWCT1, characterized by rising alkalinity and ionic content between early June and late July due to evaporative concentration. In contrast, most ponds with apparent hydrological connectivity displayed SWCT2 or SWCT3, characterized by marked changes in suspended sediment and total nitrogen concentrations due to inflow that transferred allochthonous materials from the catchment. Ponds in SWCT2 likely possessed temporary hydrological connections during periods of relatively high water supply and exhibited marked decline of suspended sediment and total nitrogen content when hydrological connection was lost. Most ponds in SWCT3 maintained active hydrological connections during all or most of the ice-free season and possessed relatively high suspended sediment and total nitrogen concentrations throughout the season. Ponds in SWCT4 possessed relatively stable water chemistry due to greater water depth and local features that reduced wind-induced sediment resuspension. We conclude that hydrological connectivity and basin morphometry exert important influence on seasonal pond water-chemistry dynamics.
Introduction
Tundra ponds dominate many northern landscapes and provide productive habitat and refuge for microorganisms, plants, and animals in regions with extreme environmental conditions (CitationSmol and Douglas, 2007; CitationVincent et al., 2011). These important aquatic habitats exist, in large part, due to the presence of permafrost, which creates an impermeable barrier and limits the amount and rate that precipitation enters the subsurface (CitationRouse et al., 1997). As a consequence, water flows over land surfaces or through the shallow active layer and pools in depressions to form ponds (CitationWoo and Winter, 1993). These shallow-water systems are among the most dynamic elements of permafrost landscapes (CitationJones et al., 2011). Because of their high surface area—to—depth ratio, they are highly responsive to variations in hydro-climatic conditions (CitationRouse et al., 1997). For example, periods of elevated evaporation without sufficient precipitation can lead to rapid water-level drawdown and markedly alter physical, chemical,and biological conditions and habitat availability (CitationSmol and Douglas, 2007). Thus, interactions among permafrost, the land surface, and climatic conditions play important roles in determining the genesis, development, and fate of tundra ponds, and the critical habitat they provide. To a large extent, these interactions are mediated by hydrological processes such as the transfer of water by precipitation, evaporation, and surface and subsurface flows (e.g., CitationRouse et al., 1997; CitationJones et al., 2011; CitationWolfe et al., 2011).
Although tundra ponds are responsive to variations in climate, it remains difficult to anticipate the direction of change in the waterbalance to ongoing climate warming. As the climate warms in Canada's north, ice-free season duration will continue to increase (CitationProwse et al., 2011). Longer duration of ice-free conditions is expected to cause more negative pond water balances, because pond evaporation exceeds precipitation during the ice-free season in many regions (CitationRouse et al., 1997; CitationSchindler and Smol, 2006). Thawing permafrost and accelerating thermokarst processes can also lead to alteration of pond surface area and depth, especially if they lead to sudden drainage via deepening of taliks under ponds (CitationWoo et al., 1992) or lateral breach of shorelines (CitationTurner et al., 2010; CitationJones et al., 2011). But, permafrost thaw can also increase inflow to ponds.
Beyond raising temperature, climate warming also modifies patterns of precipitation due to shifting and decreased influence of cold, dry arctic air masses, accompanied by increased influence of warmer moisture-laden air masses (CitationRouse et al., 1997). For example, atmosphere-ocean models suggest that shrinking seaice cover will result in increased precipitation over adjacent land areas (CitationGagnon and Gough, 2005). As a consequence, many regions are likely to experience marked changes in annual precipitation, the frequency and intensity of precipitation events, and the timing and relative proportions of rain and snow. Our ability to predict changes in precipitation remains less certain than for temperature, but changes in the timing, amount, and form of precipitation are anticipated to exert the strongest influence on limnological conditions of tundra ponds, via changes in pond water balance (e.g., CitationBoudreau and Rouse, 1995; CitationMacrae et al., 2004; CitationWolfe et al., 2011), biogeochemical cycles (CitationMacrae et al., 2004; CitationBos and Pellatt, 2012), and habitat availability (CitationRouse et al., 1997; CitationMallory et al., 2010; CitationRautio et al., 2011). Unfortunately, linkages between hydrological processes and limnological conditions of shallow tundra ponds remain under-studied, even though such knowledge is critical to anticipate pond responses to climate-driven hydrological changes (CitationRouse et al., 1997).
The Hudson Bay Lowlands (HBL) occupy an extensive subarctic wetland landscape of low topographic relief, where concerns continue to grow about consequences of climate warming on tundra ponds (CitationVincent et al., 2011). The HBL encompasses 475,000 km2 along the eastern, southern, and western shores of Hudson Bay (CitationRouse, 1991; CitationGriffis et al., 2000). Shallow (<1 m) tundra ponds are a dominant feature in this ecologically significant wetland landscape. They cover ∼25% of the surface area in northwestern HBL and provide extensive shoreline habitat for nesting waterfowl, shorebirds, and other animals (CitationMacrae et al., 2004). Instrumental records identify an overall trend of rising air temperature during the past 50 years (CitationDyke and Sladen, 2010; CitationGagnon and Gough, 2005), including both the eastern (CitationBhiry et al., 2011) and western (CitationMacrae et al., 2014) shores of Hudson Bay. Temperatures cooled between the 1960s and mid-1990s (CitationChapman and Walsh, 1993; CitationGough et al., 2004; CitationRouse, 1991; CitationMacrae et al., 2014) but have increased since then. Associated with the warming, sea-ice cover has declined (CitationHochheim and Barber, 2010; CitationGagnon and Gough, 2005). Reduction of sea-ice cover and longer ice-free seasons on Hudson Bay are expected to alter the seasonal amounts and timing of rainfall to adjacent areas (CitationGagnon and Gough, 2005; CitationSauchyn and Kulshreshtha, 2008). Already, annual rainfall has increased by ∼50% (1943–2008), based on measurements at the Churchill Airport meteorological station, with most of the increase occurring in late summer and fall (CitationMacrae et al., 2014). Dyke and Sladen (Citation2010) suggested that with continued warming, permafrost degradation may occur in this region, leading to collapse of peat plateaus and expansion of fens. Also, they suggested that the number and size of ponds will likely increase as runoff accumulates in subsiding areas. This may lead to the coalescence of ponds, as has been observed in other areas of the HBL (e.g., CitationPayette et al., 2004), or may lead to the drainage of water bodies (CitationTurner et al., 2010). Collectively, these changes have the potential to modify surface and subsurface flows that control the water balances, limnological conditions, and biogeochemical cycling in ponds of the HBL (CitationMacrae et al., 2004; CitationWolfe et al., 2011; CitationMacrae et al., 2014).
Hydro-limnological responses of tundra ponds in the HBL to climate warming remain unknown, but initial studies suggest responses will be diverse and dependent upon individual basin characteristics and the timing and degree of hydrological connectivity (CitationMacrae et al., 2004; CitationWolfe et al., 2011). Macrae et al. (Citation2004) identified that hydrological connection between ponds and the surrounding terrestrial landscape strongly influenced pondwater carbon cycling, as ponds transitioned from net sources to net sinks of CO2 as hydrological connectivity to the terrestrial landscape was lost. Wolfe et al. (Citation2011) tracked seasonal patterns of change in water balance of ponds using isotopes and found that differences in hydrological connectivity strongly influenced pond water balances, with evaporation exerting a strong influence on water levels during the ice-free season in ponds that lacked hydrological connection with their catchment. But, evaporation losses were offset by inflows in ponds with continuous hydrological connections via surface and subsurface flows. Although these studies have greatly increased our understanding of the hydrological factors that influence water balance and carbon exchange of tundra ponds in the HBL, knowledge remains insufficient to anticipate a potentially broader range of limnological responses to climate-mediated alteration of hydrological processes.
In northwestern HBL, most limnological studies are quite recent and have generally employed short-term spatial surveys to describe the limnological status of ponds and explore patterns of variation in water-chemistry variables. For example, Bos and Pellatt (Citation2012) conducted one-time sampling of 32 ponds within and adjacent to Wapusk National Park in July 2004, Rautio et al. (Citation2011) sampled three ponds near the Churchill Northern Studies Centre during July of 2005, and Symons et al. (Citation2012) sampled 21 basins within Wapusk National Park in July of 2009. Collectively, their results demonstrate that the ponds in northwestern HBL are shallow (mostly ≤1 m), well mixed, dilute, alkaline, and oligotrophic. Using seven-day incubations, Symons et al. (Citation2012) found that phytoplankton growth was limited by phosphorus (P) in 26% of the ponds, by nitrogen (N) in 13% of the ponds, and co-limited by N and P in 26% of the ponds. Notably, phytoplankton was not limited by N or P in 38% of the ponds, despite low concentrations of nutrients in the water. These studies, however, did not involve repeated sampling of water-chemistry variables in the ponds to determine patterns of water-chemistry variation during the icefree season, and they did not assess relations between hydrological processes and water chemistry. However, the presence or absence of hydrological connections between ponds and their catchments exerts strong control on pond water balances (CitationWolfe et al., 2011) and carbon cycling and pH (CitationMacrae et al., 2004) and so also have strong potential to regulate seasonal patterns of water-chemistry variation.
Here, we measured hydrological variations and limnological variables (water chemistry, suspended sediments, chlorophyll-a) during the ice-free season of 2010 at 20 shallow ponds within the Churchill Wildlife Management Area (CWMA), near Churchill, Manitoba, in northwestern HBL, to explore relations among hydrological connectivity, basin morphometry, and limnological conditions. We determined the presence and absence of hydrological connections based on visual detection of water flow paths in oblique aerial photos collected during summer of 2011 and observations during sampling. This study is exploratory given the relatively low number of study sites and the low number of sampling episodes (one year with three sampling episodes).
Study Area
The HBL extends inland from the eastern, southern, and western shores of Hudson Bay and James Bay to an elevation of less than 200 m above sea level (CitationRouse, 1991). The HBL is unique because it is the southernmost region in North America with permafrost (CitationRouse, 1991). The presence of permafrost results in abundant bogs, fens, and ponds (CitationRoulet et al., 1992; CitationWoo and Winter, 1993; CitationHamilton et al., 1994; CitationChapin et al., 2000; CitationPayette et al., 2004). The Arctic treeline boundary also occurs within the HBL, where the terrestrial vegetation shifts from boreal forest in the south to Arctic tundra in the north (CitationDuguay and Lafleur, 2003). The HBL has been undergoing isostatic rebound since the retreat of the Laurentide ice sheet. The HBL is underlain by Ordovician and Silurian limestone and dolomite, beneath which Precambrian rocks such as sedimentary gneiss, granitoid, and volcanic rocks are found (CitationDredge, 1992). A layer composed of a mixture of marine silts and Pleistocene glacial till is found on top of the bedrock, which is overlain by peat that ranges in depth from a minimum of a few centimeters by the coast of Hudson Bay to a maximum of ∼1 m adjacent to the most inland locations of our study ponds (CitationDredge, 1992).
For this study, 20 ponds were selected from within CWMA in northwestern HBL to capture a representative range of hydroecological conditions in the region (), based on information provided by Macrae et al. (Citation2004). Ponds in this study are <1.2 m deep and freeze completely in winter. Most of these ponds possess thick deposits of organic-rich sediments, which also freeze in winter (CitationMacrae et al., 2004). The ponds are underlain by continuous permafrost, and to our knowledge taliks do not exist beneath them. The active layer is limited to 0.4 m below the surface in peat plateaus and ∼0.8–1.0 m below the surface in fens (CitationMacrae et al., 2004). As a consequence, most runoff to ponds is through peat rather than through mineral sediments. Pond freeze-up usually occurs during October to November, and break-up typically occurs in May or June (CitationDuguay et al., 2003; CitationMacrae et al., 2014). Due to their shallow depth, ponds in this region are well mixed and isothermal throughout the ice-free season (CitationStewart and Rouse, 1976; CitationBos and Pellatt, 2012). The ponds were selected to span a range of hydrological “connectivity” (connected via channel fens versus isolated in peat plateaus) that exist in the landscape (CitationWolfe et al., 2011). All of the study ponds are situated near Hudson Bay and lie within the Hudson Plains ecozone. Most of the ponds are located where catchment vegetation consists of peat bogs supporting a heathlichen community and channel fens that are dominated by sedges and graminoids (). Four ponds (Erin, Larch, Orange, and Strange) are situated slightly farther inland within a transitional zone characterized by a mixture of polygonal peat plateaus and sedge fens. Here, the vegetation consists of sparse cover by small shrubs (e.g., Betula glandulosa, dwarf Salix species) and coniferous trees (Picea mariana, P. glauca, and Larix laricina) that are stunted and sparsely arranged in Krumholtz formation. Serene Pond is located farthest inland within the spruce-lichen forest, where the vegetation is taller and denser than at the other study ponds. Overall, 19 of the 20 study ponds are situated less than 6 km south of the Hudson Bay coast, whereas Serene Pond lies 16 km south of the coast.
FIGURE 1. Map illustrating locations of the 20 shallow tundra ponds located within the Churchill Wildlife Management Area of western Hudson Bay Lowlands that were selected for analyses of water chemistry and water isotope composition in this study.
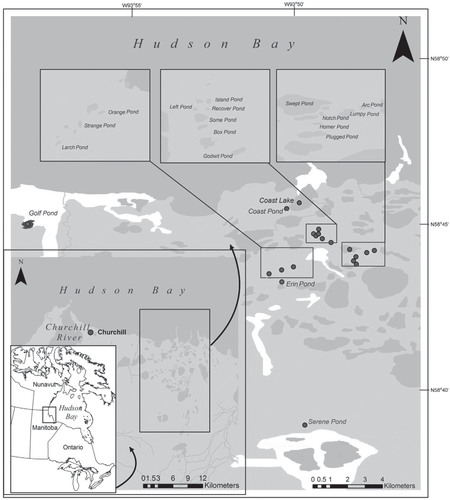
TABLE 1 Geographic location (latitude, longitude), surface area (ha), mean depth (m), and surface area-to-depth ratio for the 20 study ponds within the Churchill Wildlife Management Area.
Compared to climate normals for 1971–2000 (CitationEnvironment Canada, 2011a, Citation2011b), temperatures during 2010 were warmer in January through April and October through December (). Relative humidity was higher than the climate normals during all months of 2010, except for May and June. Prior to the ice-free season of 2010, winter snowfall was below climate normals (January–April). From May through September of 2010, monthly precipitation was comparable to the climate normals, except for below-average precipitation in June and above-average rainfall in August. A large rain event on 24 August (∼105 mm) caused the monthly total to become more than double that of the climate normal ().
Methods
FIELD AND LABORATORY
To assess the seasonal variability in hydrological and water-chemistry conditions, water samples and limnological measurements were obtained from near the center of each of the 20 study ponds on 5 June, 28 July, and 20 September, 2010. Ponds in the region became ice-free during the last days of May (CitationLight, 2011). These sampling dates were selected to capture temporal variations during the ice-free season, including the influence of snowmelt (early June), dry conditions during mid-summer (late July), and late-summer precipitation (mid-September). This pattern is typical of the Churchill region (CitationRouse, 1998; CitationMacrae et al., 2004).
FIGURE 2. Comparison of (a) mean monthly air temperature, (b) mean monthly relative humidity, and (c) monthly precipitation during 2010 with climate normal data for the period 1971–2000 recorded at the airport at Churchill, Manitoba (Meteorological Station 5060601; CitationEnvironment Canada, 2011a, Citation2011b).
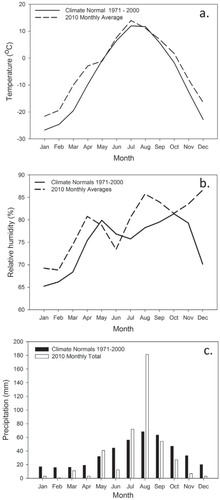
At each pond, water temperature, pH, and conductivity were measured in situ using a YSI 600QS handheld multimeter positioned ∼10 cm below the water surface. Water samples were collected from ∼10-cm water depth for laboratory analysis of suspended sediments (total [TSS], and inorganic [ISS] and organic [OSS] fractions), chlorophyll-a (Chl-a), water chemistry (alkalinity, pH, and concentrations of major nutrients and ions), and water isotope composition. An inflatable kayak was used to obtain the measurements and water samples. For analysis of suspended sediments, measured volumes of pond water were filtered at the field base (Churchill Northern Studies Centre) through pre-combusted (1 h @ 550 °C), pre-weighed Whatman® GF/C filters (pore size 1.2 µm). For analysis of Chl-a, measured volumes of pond water were filtered through Whatman® GF/F filters (pore size 0.7 µm). Immediately after filtration, all filters were stored frozen until analysis at the University of Waterloo. The filters were then analyzed for suspended sediments using standard methods (CitationLind, 1974), and for Chl-a concentrations using standard fluorometric techniques (CitationParsons and Strickland, 1963; CitationSCOR/ UNESCO, 1966).
Chemical analyses of the water samples included alkalinity and concentrations of total phosphorus (TP), soluble reactive phosphorus (SRP), nitrate and nitrite (NO3 - + NO2 -), ammonium (NH4 +), total Kjeldahl nitrogen (TKN), and major ions (Cl-, SO4 2-, Na+, K+, Mg2+, Ca2+). For analysis of dissolved chemical species (SRP, NO3 - + NO2-, NH4+), water samples were filtered through a 0.45-µm cellulose acetate filter at the field base and kept at 4 °C in the dark until analysis. Subsamples for determination of phosphorus were preserved with H2SO4 (0.2% final concentration). Waterchemistry analyses were performed using standard colorimetric techniques (Bran Luebbe AA3, Seal Analytical, Seattle, U.S.A., Methods G-189-097 [TKN], G-188-097 [TP], G-102-93 [NH4 +], G-109-94 [NO3 - + NO2-], G-103-93 [SRP], G-148-95 [alkalinity])and ion chromatography (DIONEX ICS 3000, IonPac AS18 and CS16 analytical columns).
Pond samples for water isotope composition were collected from each study pond in 30-mL HDPE bottles and measured using standard techniques (CitationEpstein and Mayeda, 1953; CitationMorrison et al., 2001). Water isotope compositions are expressed as δ-values, representing deviations in per mil (‰) from Vienna Standard Mean Ocean Water (VSMOW) such that δsample = [(Rsample/RVSMOW) − 1] × 103, where R is the 18O/16O or 2H/1H ratio in sample and VSMOW. Results of δ18O and δ2H analyses are normalized to −55.5‰ and −428‰, respectively, for Standard Light Antarctic Precipitation (CitationCoplen, 1996). Analytical uncertainties are ±0.2‰ for δ18O and ±2.0‰ for δ2H.
To assist interpretation of the role of hydrological connectivity in mediating changes in pond water chemistry and water isotope compositions, we used visual inspection of oblique aerial photographs obtained by M. Macrae during airplane surveillance in summer of 2011 to determine the presence or absence of apparent hydrological connections at each of the study ponds in addition to observations made at ponds during field sampling. Connectivity was either assessed by the presence or absence of an open-water channel, or, was inferred using vegetation patterns and topography.
NUMERICAL ANALYSIS
A principal components analysis (PCA) was performed on the data set of limnological variables obtained during the three sampling periods to explore patterns of variation in water chemistry among the study ponds during the ice-free season in 2010 in relation to hydrological changes captured by the water isotope tracers. PCA, a method of indirect gradient analysis based on a linear response model, is well suited for analysis of multivariate environmental data (Citationter Braak and Šmilauer, 2002). The waterchemistry variables were entered as active explanatory variables, whereas water temperature, water isotope composition (δ18O, δ2H), and TN concentration were included as passive variables, so they did not influence the ordination results. The PCA was run using the software CANOCO version 4.5 (Citationter Braak and Šmilauer, 2002) following methods used by Sokal et al. (Citation2010) and Wiklund et al. (Citation2012) to explore temporal patterns of change in water chemistry of shallow ponds. Prior to running the PCA, SRP concentration was removed because values were below detection limits (1 µg L-1) in more than 25% of the study ponds. TP concentration was also removed, because extremely high values (>400 µg L-1) were obtained for a few samples collected in early June and late July. A cause for the high values could not be identified, but they were considered to be unreliable because ponds in the region possess much lower TP concentrations (CitationBos and Pellatt, 2012; CitationSymons et al., 2012). To avoid reducing the sample size, the TP data were omitted from numerical analyses (rather than deleting the ponds with the high TP values). Based on data in Symons et al. (Citation2012), concentration of TP is correlated with TKN in adjacent shallow ponds. Thus, TKN concentration served as a surrogate for TP concentration. Prior to analysis by PCA, all limnological variables were assessed for normality using Kolmogorov-Smironov tests (alpha = 0.05) performed with SPSS version 19.0 software. Those variables with non-normal distributions were transformed using ln(x + b) (where b = 0.5 × the minimum non-zero value), and normality was confirmed by reassessment.
Results
WATER-CHEMISTRY CHARACTERISTICS AND TEMPORAL VARIATION
The 20 study ponds were, on average, alkaline (mean pH = 8.66; mean alkalinity = 118.81 mg CaCO3 L-1), with low to moderate ionic content (mean conductivity = 440.3 µS cm-1; Appendix –). They were typically oligotrophic, based on concentration of total nitrogen (mean TKN = 0.93 mg L-1), Chl-a (1.42 µg L-1), and SRP (mostly below the detection limit). Based on visual observations during pond sampling, shorelines consisted of organic soils and peat, and the pond bottoms consisted of fine-grained organic sediment in most ponds. However, cobbles dominated the bottom of Coast Lake. Macrophyte cover was extremely sparse, and pond bottoms often possessed luxurious growth of a benthic algal biofilm.
Temporal patterns were observed in many of the measured water-chemistry variables. On average, pond-water pH decreased by 0.6 units from early June to mid-September, while conductivity tripled and alkalinity and concentrations of Ca2 +, Cl-, and SO4 2- increased (–). Average TKN concentration increased between early June and late July (0.77 mg L-1 to 1.30 mg L-1), and then declined between late July and mid-September (to 0.73 mg L-1). Similarly, average concentrations of Na+, K+, and TSS increased between early June and late July, and then declined from late July to mid-September (–). Average concentrations of inorganic nitrogen (NO3 - + NO2 -, NH4+) decreased in the ponds from early June to late July, but remained fairly constant between late July and mid-September. Chl-a concentration, on average, remained relatively constant among the sampling episodes (1.31, 1.54, and 1.40 µg L-1 in early June, late July, and mid-September, respectively).
Principal components analysis was used to explore the main gradients of difference in water chemistry among ponds. PCA axis 1 captured 36.3% of the variation in limnological conditions among the study ponds, and PCA axis 2 explained a further 19.5% of the variation (total = 55.8%). Alkalinity, conductivity, and concentrations of the major ions (Ca2+, K+, Mg2+, Na+, Cl-, and SO4 2- ) were positively correlated with PCA axis 1, while concentration of NO3- + NO2- was negatively correlated with axis 1 (). Concentrations of Chl-a, suspended sediments (TSS, ISS, OSS), and total Kjeldahl nitrogen (TKN) were negatively correlated with PCA axis 2, with weaker positive correlation along axis 1 (). Concentration of NH4+ and pH were negatively correlated with PCA axes 1 and 2. Sample scores that plotted to the right along PCA axis 1 identified ponds with relatively higher alkalinity, conductivity, and concentrations of major ions and, to a lesser extent, higher concentrations of total nitrogen and suspended sediment. Sample scores positioned to the left along PCA axis 1 identified ponds with lower values of these variables and relatively high concentrations of NO3- + NO2-, NH4+, and high pH. Sample scores positioned low on PCA axis 2 identified ponds with relatively high concentrations of Chl-a, total nitrogen, and suspended sediments (TSS, ISS, OSS). Vectors for water isotope composition (δ2H and δ18O), TN concentration, and water temperature, included as passive variables, were negatively correlated with axis 2, suggesting that ponds with isotopically enriched values possessed relatively high concentrations of most major ions, total nitrogen, phytoplankton biomass (as Chl-a), and suspended sediments.
The temporal patterns of variation in the PCA sample scores during the three sampling episodes in 2010 allowed us to identify that the 20 study ponds followed one of four different “seasonal water-chemistry trajectories” (SWCTs) (). For one group (hereafter referred to as SWCT1), sample scores increased markedly along PCA axis 1 between early June and late July, with relatively little change along PCA axis 2 (, part a). This pattern contrasts with ponds in the other SWCT categories, where sample scores moved markedly downward (SWCT2, SWCT3) or upward (SWCT4) between early June and late July. Between late July and mid-September, sample scores for the eight ponds in SWCT1 (Arc, Box, Coast Pond, Godwit, Left, Notch, Plugged, and Recover) characteristically increased along PCA axis 2, with relatively small movement along PCA axis 1. This temporal pattern indicated that alkalinity and concentrations of major ions increased markedly in these ponds between early June and late July, and that changes in concentration of total nitrogen (TKN, TN), Chl-a, and suspended sediments were relatively small compared to ponds in the other SWCT categories. Between late July and mid-September, movement was negatively correlated with vectors for water isotope composition and concentrations of total nitrogen, Chl-a, and suspended sediments, indicating their values declined. At this time, the direction of movement was orthogonal to vectors for alkalinity, pH, and concentrations of major ions and NH4+, indicating very little change in their values.
For a second group of six ponds (SWCT2: Golf, Island, Larch, Lumpy, Orange, and Swept), sample scores declined markedly along PCA axis 2 between early June and late July (, part b). Although of lesser magnitude, there was also net movement to the right along PCA axis 1 at this time. Then, between late July and mid-September, sample scores rose markedly along PCA axis 2, but the direction of movement along PCA axis 1 was inconsistent among ponds. Although sample scores moved farther along PCA axis 2 than PCA axis 1 between adjacent sampling episodes, the net change between early June and mid-September was characterized by a modest shift to the right along PCA axis 1. This temporal pattern indicated that concentrations of suspended sediments (TSS, ISS, and OSS), total nitrogen (TKN, TN), and Chl-a increased markedly between early June and late July in all these ponds. The net increase in PCA axis 1 scores also indicated that modest increases in alkalinity, conductivity, and concentrations of most major ions (Ca2+, K+, Mg2+, Na+, Cl-, and SO42-) occurred between early June and late July. Between late July and mid-September, ponds in SWCT2 experienced marked declines in concentrations of suspended sediments, total nitrogen, and Chl-a, and in water isotope composition.
FIGURE 3. Principal component analysis (PCA) ordination diagram illustrating the main gradients of limnological differences among the 20 study ponds based on 16 limnological variables measured during early June, late July, and mid-September 2010. The ponds are located within the Churchill Wildlife Management Area of western Hudson Bay Lowlands. The variables δ18O, δ2H, total nitrogen (TN), and water temperature (lnWtemp) were included as passive variables. Sample scores are labeled using the first three or four letters of their corresponding pond name, and the number represents the sampling episode (1 = early June, 2 = late July, 3 = mid-September). SWCT = seasonal water-chemistry trajectories.
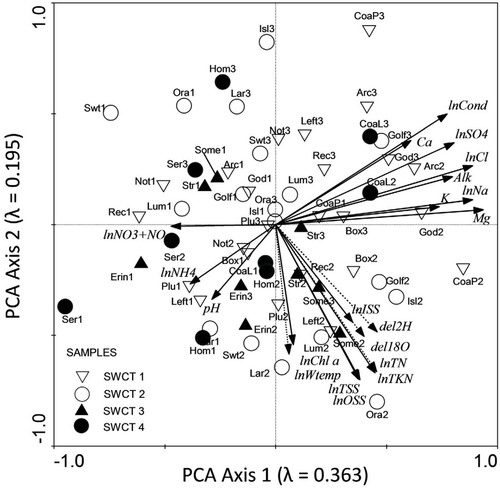
Similar to ponds in SWCT2, sample scores for a third group of ponds (SWCT3; three ponds: Erin, Some, and Strange) moved downward along PCA axis 2 and to the right along PCA axis 1 between early June and late July of 2010 (, part d). For ponds in SWCT3, however, most of the changes occurred between early June and late July, with relatively constant conditions between late July and mid-September (as indicated by relatively short, near-vertical arrows in , part d). These patterns indicated ponds in SWCT3 were characterized by low initial concentration of suspended sediments and ions, followed by marked increases in concentrations of suspended sediments and total nitrogen (TKN, TN) between early June and late July, and stable water chemistry for the remainder of the ice-free season.
Sample scores for ponds in the fourth category (SWCT4; three ponds: Coast Lake, Homer, and Serene) were positioned toward the lower left quadrant of the PCA plot in early June and consistently moved higher along PCA axis 2 as the ice-free season progressed (, part e). Between early June and late July, sample scores also moved toward the right along PCA axis 1, but little movement was observed along PCA axis 1 between late July and early September. This temporal pattern indicated ponds in SWCT1 initially had low ionic content with high pH and high concentrations of NH4 + and NO3- + NO2- in early June relative to ponds in the other SWCT categories and high water-isotope composition and high concentrations of Chl-a, total nitrogen (TKN, TN), and suspended sediments. The direction of movement of sample scores between early June and late July indicated that concentrations of NH4+ and pH declined, while conductivity, alkalinity, and concentrations of the major ions (Ca2+, K+, Mg2+, Na+, Cl-, and SO4 2-) increased markedly. The direction of movement was orthogonal to vectors for concentrations of suspended sediments and total nitrogen, indicating these variables did not change appreciably during this time. Net movement of sample scores upward along axis 2 between late July and early September indicated modest declines in concentrations of total nitrogen, Chl-a, and suspended sediments, and a decrease in water isotope composition, but with little to no change in pH, alkalinity, and concentrations of major ions.
BASIN MORPHOMETRY, WATER ISOTOPE COMPOSITION, AND HYDROLOGICAL CONNECTIVITY
The study ponds were generally shallow (median = 0.33 m) and small (median = 0.7 ha; ). The deepest pond had mean depth of 1.2 m, and only 3 of the 20 ponds were ≥1 m deep. Only 3 of the 20 ponds were >4 ha in surface area. The three ponds in SWCT4 were deeper than all but one of the ponds in the other categories. Ponds in SWCT categories 1 and 3 were consistently the smallest, were relatively shallow, and thus possessed the smallest water volume (, parts b and c).
In , the temporal pattern of variation in pond-water δ18O values were compared among the SWCT categories based on samples obtained at the same time as those for the water chemistry analyses. Pond-water δ2H values were closely correlated with δ18O signatures (R 2 = 0.90, P < 1.0 × 10-9, d.f. = 58); thus, only the δ18O data were presented as a way to compare patterns of pond waterbalance variation within and among the SWCT categories. This information provided a way to explore relations between water balance and differences in water-chemistry behavior and basin morphometry of ponds in the four SWCT categories. In general, all 20 study ponds displayed a common pattern of variation in pond water δ18O, characterized by low values in early June (generally below -10‰) shortly after melt of snow and ice, followed by higher values in late July when precipitation was low and evaporation was high, and by a return to lower values in mid-September after substantial rainfall occurred in late August ( and ). Only Godwit Pond and Notch Pond (both in SWCT1) deviated from this general pattern; these ponds exhibited relatively constant δ18O values between late July and mid-September.
Beyond the general pattern of pond-water δ18O values, as described above, there were notable similarities among ponds within each of the SWCT categories and differences among the SWCT categories (). For example, six of the eight ponds in SWCT1 (Box, Coast Pond, Left, Notch, Plugged, and Recover) all experienced marked increases in δ18O between early June and late July to values near the estimated value for δ18OSSL, the terminal basin steady-state value that corresponds to a pond water balance where inflow equals evaporation. All six of these ponds lacked apparent hydrological connections with their catchments, suggesting they are closed-drainage basins (e.g., Left Pond in ). At four (Box, Coast Pond, Left, and Recover) of these six ponds, δ18O in late July exceeded δ18OSSL, indicating net evaporative water loss. In contrast, two of the eight ponds in SWCT1 (Arc and Godwit) experienced less increase in δ18O between early June and late July (). Oblique aerial photos and observations during fieldwork identified that Arc Pond is hydrologically connected to wetlands and ponds via flow channels and that Godwit is intermittently connected.
The δ18O values were consistent among the six ponds in SWCT2 in early June and late September (). Four of the six SWCT2 ponds experienced less evaporative 18O-enrichment between early June and late July in comparison to the hydrologically closed-drainage ponds in SWCT1 (). Channels connect these four SWCT2 ponds to adjacent ponds or wetlands (e.g., Larch Pond in ). In contrast, the remaining two ponds in SWCT2 (Island and Lumpy) experienced more marked increase in δ18O between early June and late July. Hydrological connections were absent at Lumpy Pond and intermittent at Island Pond. Ponds in SWCT2 spanned a relatively broad range of depth and surface area, though they were generally shallower and smaller than most ponds in SWCT4, which were the deepest and largest ponds in the data set ().
Of the three ponds in SWCT3, two (Erin and Strange) displayed a pattern of change in pond-water δ18O that is comparable to ponds in SWCT2 with apparent hydrological connections. Consistently, Erin Pond and Strange Pond received inflow via apparent hydrological connections to adjacent ponds and wetlands. However, evaporative 18O-enrichment was more evident at Some Pond between early June and late July when δ18O exceeded δ18OSSL. Correspondingly, aerial photos, water isotope composition, and a previous hydrologic study (CitationMacrae et al., 2004) showed no evidence of an inflow channel to Some Pond. Compared to the other SWCT categories, ponds in SWCT3 possessed intermediate depth and consistently small surface area ().
All three of the ponds in SWCT4 displayed less-variable δ18O values during the ice-free season of 2010, and values in late July remained relatively low compared to ponds in the other SWCT categories (). Also, SWCT4 ponds were deeper than all but one of the ponds in the other categories (). Coast Lake was the largest and deepest of the study ponds and, thus, holds the largest volume of water (), but Homer and Serene ponds possessed comparable small surface area to most other study ponds (, , part c). Aerial photos and field-based observations indicated that Coast Lake and Serene Pond had apparent hydrological connections, whereas Homer Pond lacked detectable hydrological connections. Overall, greater depth and presence of hydrological connections for SWCT4 ponds corresponded with relatively less evaporative 18O-enrichment between early June and late July compared to most ponds in other SWCT categories.
In summary, evidence suggested that 9 of the 20 study ponds are hydrologically closed-drainage ponds. Of these, 6 were placed in SWCT1 (Box, Coast Pond, Left, Notch, Plugged, and Recover), 1 was placed in SWCT2 (Lumpy), 1 was placed in SWCT3 (Some), and 1 was placed in SWCT4 (Homer) based on their temporal pattern of water-chemistry variation. For the remaining 11 ponds, evidence suggested they have inflow channels that provided waters from adjacent ponds, wetlands, or both ponds and wetlands. Nine of these 11 ponds were placed into SWCT2, SWCT3, or SWCT4 categories, whereas only 2 (Arc and Godwit) were placed in SWCT1, along with most of the hydrologically closed-drainage ponds.
FIGURE 4. Principal component analysis (PCA) ordination diagrams illustrating four distinctive patterns of change in limnological conditions among the 20 study ponds during the ice-free season of 2010. The four distinctive seasonal water-chemistry trajectories (SWCT) are shown in separate panels: (a) SWCT1 (n = 8 ponds); (b) SWCT2 (n = 6); (d) SWCT3 (n = 3); and (e) SWCT4 (n = 3). (c) Vectors for the environmental variable (solid lines represent active variables [n = 16], dotted lines represent passive variables [n = 4]).
![FIGURE 4. Principal component analysis (PCA) ordination diagrams illustrating four distinctive patterns of change in limnological conditions among the 20 study ponds during the ice-free season of 2010. The four distinctive seasonal water-chemistry trajectories (SWCT) are shown in separate panels: (a) SWCT1 (n = 8 ponds); (b) SWCT2 (n = 6); (d) SWCT3 (n = 3); and (e) SWCT4 (n = 3). (c) Vectors for the environmental variable (solid lines represent active variables [n = 16], dotted lines represent passive variables [n = 4]).](/cms/asset/2fefd50b-db52-497e-a45c-42e2445bbba4/uaar_a_11957725_f0005.jpg)
FIGURE 5. Comparison of basin morphometry among the four seasonal water-chemistry trajectory (SWCT) categories of ponds. (a) Boxplots comparing maximum pond depth (m) among the four SWCT categories. (b) Boxplots comparing pond surface area (ha) among the four SWCT categories. (c) Scatterplot illustrating relations between water depth and surface area of the ponds in the four SWCT categories.
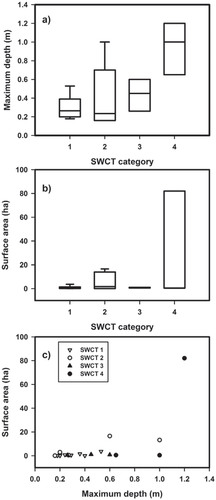
Discussion
Due to their shallow depth, large ratio of surface area to water volume, and influence of permafrost, tundra ponds have become well recognized as dynamic and vulnerable to effects of hydrological changes (CitationRouse et al., 1997; CitationJones et al., 2011; CitationVincent et al., 2011). Yet, relations between hydrological processes and limnological conditions of tundra ponds have remained poorly understood. This is true for the HBL, where more than 10,000 shallow tundra ponds cover ∼25% of the land area (CitationDuguay and Lafleur, 2003; CitationMacrae et al., 2004), but where integrated hydrolimnological studies remain sparse (e.g., CitationMacrae et al., 2004) despite a recent increase in studies assessing spatial variation of water chemistry and controls on primary production (CitationRautio et al., 2011; CitationBos and Pellatt, 2012; CitationSymons et al., 2012). Here, based on exploratory limnological measurements performed at three times during the ice-free season of 2010 at 20 shallow ponds within the CWMA of northwestern HBL, we identify distinct trajectories of water-chemistry variation. In conjunction with hydrological behavior, based on water isotope composition, basin morphometry, and additional supporting evidence, we suggest that these trajectories correspond well with apparent differences in hydrological connectivity of the ponds to their adjacent catchments, and that differences in pond morphometry and other physiographic factors are also important.
A study by Wolfe et al. (Citation2011) used analyses of water isotope tracers and water-level loggers to characterize hydrological processes that influence present-day water balances at three of the ponds that were also included in our study (Left, Larch, and Erin). Water-level records (daily averages based on measurements recorded every 30 minutes) at these three ponds, coupled with water isotope analyses (including biweekly water isotope sampling at Left Pond), allowed for more detailed characterization of hydrological processes. They identified that inputs from rain during the preceding autumn and from spring snowmelt led to depleted water isotopic signatures in June of 2010 in all three ponds, but hydrological patterns differed during the remainder of the ice-free season in the magnitude of evaporative isotopic enrichment during summer and subsequent isotopic depletion due to precipitation in late August (see also ). In the absence of apparent hydrologic connection between Left Pond and its catchment, net evaporation caused isotopic enrichment and water-level drawdown by mid-July (see also and ). In contrast, apparent hydrological connections (via channel fens) at Larch and Erin Ponds provided greater water inflow from their catchments, which resulted in less evaporative enrichment and water-level drawdown (see also and ). Based on paleolimnological evidence provided by records of cellulose-inferred pond-water δ18O, Wolfe et al. (Citation2011) showed that water balances of Left and Larch Ponds became increasingly influenced by evaporation since the early 20th century, likely in response to longer duration of the ice-free season as the climate warmed. But, hydrological conditions diverged in the 1960s at Larch Pond, which was speculated to have been associated with the establishment of a channel fen leading to greater hydrological connectivity. The channel intermittently conveys water flow to Larch Pond from an adjacent pond and contributing wetlands, which offsets evaporative losses compared to Left Pond. Consequently, Left Pond has experienced greater evaporative enrichment since the 1960s than has occurred at Larch Pond, because Left Pond has remained hydrologically disconnected from adjacent ponds and peatlands. Paleolimnological evidence indicates Erin Pond's water balance has remained relatively constant during the past three centuries due to hydrological connection with its larger contributing basin that supplies sufficient precipitation to offset 20th century increases in pond-water evaporation. Notably, these three hydrologically distinctive ponds displayed sufficiently different patterns of water-chemistry variation that they were placed into different SWCT categories identified during our study—a finding that suggests water chemistry of these shallow tundra ponds is strongly regulated by hydrological processes. Below, we examine further how differences in seasonal patterns of water-chemistry variation between the ponds may relate to hydrological connectivity and other factors.
FIGURE 6. Seasonal variation of δ18O compositions of water in the 20 study ponds during the ice-free season of 2010, based on water samples collected in early June, late July, and mid-September. The data are organized by the four seasonal water-chemistry trajectory (SWCT) categories: (a) SWCT1, (b) SWCT2, (c) SWCT3, and (d) SWCT4. The horizontal dashed line in each panel identifies the terminal basin steadystate δ18O value that corresponds to a pond water balance where inflow equals evaporation (δSSL; δ18OSSL = -7.5‰) (further details may be found in CitationWolfe et al., 2011).
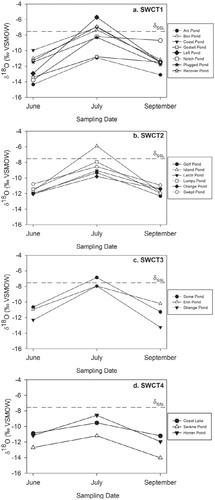
The pattern of change in water chemistry at Left Pond during the ice-free season of 2010 was comparable to patterns that occurred at the other ponds in SWCT1 (). Water isotope data indicated that a negative water balance characterized Left Pond between early June and mid-August (i.e., water isotope composition exceeded δSSL, the terminal basin steady-state value), and water-level declined (CitationWolfe et al., 2011; see also ). As late summer rains replenished water levels in Left Pond, water isotope composition decreased and water levels rose (CitationWolfe et al., 2011). These changes, coupled with the absence of an apparent inflow channel (), indicate that Left Pond is hydrologically closed. Most of the other ponds classified as SWCT1 displayed a similar pattern of change in δ18O as occurred in Left Pond during the icefree season of 2010. Indeed, six of the eight ponds in SWCT1 (Box, Coast Pond, Left, Notch, Plugged, and Recover) show marked increase in water isotope composition between early June and late July to values near δ18OSSL. Also, all these ponds similarly lack visible hydrological connections with their catchments. For ponds in SWCT1, evaporative 18O-enrichment between early June and late July was associated with marked increases in conductivity, alkalinity, and concentrations of major ions (Ca2+, K+, Mg2+, Na+,Cl-, SO4 2-), with also modest increase of concentrations of total nitrogen, suspended sediments, and Chl-a. After late-autumn rains replenished the ponds and water isotope composition declined, concentrations of major ions remained relatively unchanged, and concentrations of total nitrogen, suspended sediments, and Chl-a declined slightly. Corresponding increases in water isotope composition and concentration of ions and nutrients during early to late summer is consistent with strong control of evaporation on water chemistry and water balance in the absence of hydrological connectivity ( and ). An explanation for the absence of a decline in concentrations of major ions when late-autumn rains caused 18O depletion is more challenging. We suggest that the concentration of ions in the runoff at that time must have been similar to that in the ponds. Given that 105 mm of rain fell on 24 August, 2010, it is possible that ions became concentrated in the rainwater as it moved across the land surface and entered the ponds as runoff. Thus, a lack of apparent hydrological connection between ponds and their catchments appears to be important in mediating hydro-limnological behavior of ponds in SWCT1. Other factors are likely also at play, however, because two of the eight ponds in SWCT1 (Arc and Godwit) possess apparent hydrological connections via channel fens and did not exhibit a marked rise in water isotope composition between early June and late July comparable to the disconnected SWCT1 ponds. For these two ponds, basin morphometry may play an important role. Arc Pond and Godwit Pond are similarly shallow as Left Pond (20–24 cm mean depth), but their surface area is 10-fold larger than Left Pond. Evaporation from their pond surfaces could have sufficiently lowered water levels during the arid summer period to sever their hydrological connection. If so, ponds with a large ratio of surface area to volume and intermittent or weak hydrological connections to their catchment may, in some instances, produce comparable water-chemistry variations as disconnected ponds.
FIGURE 7. Aerial photos showing 3 of the study ponds in July 2011. The left panel illustrates that Left Pond (SWCT1) is hydrologically isolated from the surrounding terrestrial landscape, as illustrated by absence of apparent inflow or outflow channels. The right panel shows 5 ponds which are hydrologically connected by channel fens, which appear as dark green vegetated areas between the ponds. Hydrological information provided by Wolfe et al. (Citation2011) suggests that Larch Lake (SWCT2) lost hydrological connection during the summer months of 2010, whereas Erin Lake (in SWCT3) remained hydrologically connected throughout the ice-free season of 2010. Differences in the duration and timing of the hydrological connections appear to differentiate seasonal patterns of limnological change in ponds of the SWCT categories.
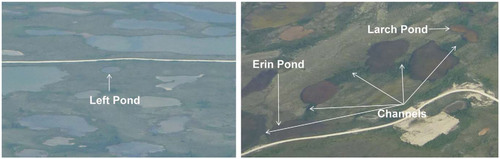
The pattern of change in water chemistry at Larch Pond was comparable to the other SWCT2 ponds during the ice-free season of 2010. Between early June and late July, concentrations of suspended sediments and total nitrogen typically increased in ponds in the SWCT2 category, while increases in ionic content were relatively small (). Between late July and mid-September, these patterns reversed, with marked declines in concentrations of suspended sediments and total nitrogen and relatively little change in ionic content. Seasonality in hydrologic connection between Larch Pond and adjacent ponds may explain these temporal changes in limnological conditions of ponds in SWCT2. Early in the ice-free season, isotopic enrichment and water-level change at Larch Pond were considerably less than at Left Pond (CitationWolfe et al., 2011), likely because lateral water flow delivered to Larch Pond via the channel fen () offsets the influence of evaporation (CitationWolfe et al., 2011). We hypothesize that inflow via the channel fen likely increased transport of suspended sediments and nitrogen to Larch Pond and reduced evaporative concentration of dissolved ions. However, after samples were collected in late July, the water level declined sufficiently in Larch Pond that hydrological connection via the channel fen became reduced or lost (CitationWolfe et al., 2011), and, correspondingly, the concentration of suspended sediments and total nitrogen declined. Most of the other ponds classified as SWCT2 displayed a similar pattern of change in δ18O as occurred in Larch Pond during the ice-free season of 2010. Indeed, four (Golf, Larch, Orange, and Swept) of the six ponds in SWCT2 showed muted increases in water isotope composition between early June and late July, and all four of them possess hydrological connections with their catchments that are visible in aerial photos. Presumably, the flow channels become periodically active when water flows and pond levels are sufficiently high, which leads to a fluctuating supply of suspended sediments and nutrients. However, the mechanism producing elevated concentrations of total nitrogen and suspended sediments in late July at Island and Lumpy Ponds remains unknown. To our knowledge, these two ponds are hydrologically disconnected from surrounding wetlands, and so supply from the catchment is not likely. Wind-driven resuspension of bottom sediments is a possible source, because these ponds are relatively shallow and lack steep banks to protect them from wind action. Island Pond is the shallowest of all the study ponds (16.2 cm mean depth; ). Lumpy Pond is only slightly deeper (19.5 cm), but it has the largest surface area of all the ponds shallower than 35 cm. Thus, these ponds are particularly prone to suspension of sediments in the pond water due to wind. Consistent with the role of sediment resuspension, Orange Pond shares comparable shallow depth with Island Pond (16.2 cm) and had the highest concentrations of suspended sediments and total nitrogen compared to all other study ponds in late July. Thus, the increased concentrations of suspended sediments and total nitrogen in these ponds in late July, typical of the SWCT2 category, may have resulted from wind-driven resuspension of bottom sediments rather than from inflow via the channel fens. Activities of waterfowl also may have promoted the observed increase of nutrients and suspended sediment concentration in late summer at Island and Lumpy Ponds, because they are unique among the 20 study ponds in the consistent presence (since the 1990s) of numerous geese nests on their islands and near the shore (M. Macrae, personal observation).
The changes in water chemistry at Erin Pond were comparable to changes at the two other ponds in SWCT3, characterized by modest increases in concentrations of suspended sediments and total nitrogen between sampling episodes in early June and late July, followed by near-constant values between late July and mid-September. For Erin Pond, this trajectory of limnological conditions can be explained by transport of suspended sediments and nutrients via inflow channels that maintain influential and continuous, or near-continuous, hydrologic connection with the surrounding landscape throughout the ice-free season (CitationWolfe et al., 2011; ). Evidently, inflow waters are elevated in concentrations of total nitrogen and suspended sediments that may influence the water chemistry in Erin and Strange Ponds, compared to ponds without apparent hydrologic connections (e.g., SWCT1; ). A similar pattern of water-chemistry behavior was observed at Some Pond, but this pond apparently lacks hydrological connection to its catchment. Some Pond is deeper (45 cm) than the hydrologically disconnected ponds in SWCT2, so it is uncertain whether wind-driven sediment resuspension may similarly account for its pattern of water-chemistry variation.
Hydro-limnological conditions of the three ponds in SWCT4 were characterized by lowest concentrations of major ions in early June and lowest magnitude of change in water isotope composition and most water-chemistry variables ( and ). Unfortunately, none of the ponds in SWCT4 was included in the study by Wolfe et al. (Citation2011), so comparable, more-detailed knowledge of pond hydrology and seasonal water-level fluctuations is not available, as it is for the other SWCT categories. Two of the ponds (Coast Lake and Serene Pond) appear to receive inflow from adjacent ponds and wetlands via hydrological connections, similar to many of the ponds in SWCT2 and SWCT3, but their concentrations of total nitrogen and suspended sediments did not similarly increase between early June and late July (as observed for ponds of SWCT2 and SWCT3; see above). Homer Pond exhibited comparable water-chemistry variation as the other two ponds in SWCT4, but does not possess apparent hydrological connections. Thus, the presence or absence of hydrological connections does not fully explain limnological variations observed in this category. In the spatial survey of Bos and Pellatt (Citation2012), ionic content of ponds differed with distance from the shore of Hudson Bay. But, this is not likely to account for waterchemistry differences among our study ponds, because they are all situated relatively close to the Hudson Bay shore (within 16 km) and the most distant (Serene) and closest (Coast Lake) sites were placed in the same SWCT category (SWCT4) on the basis of comparable variation of water chemistry. Instead, we speculate that unique catchment characteristics resulted in low supply of suspended sediments and total nitrogen (a major constituent of sediment in these ponds; CitationWolfe et al., 2011; CitationEichel et al., 2014) to the ponds in SWCT4, thus causing water chemistry to vary uniquely compared to study ponds in the other SWCT categories. Serene Pond is the only study pond that lies within the spruce-lichen forest, where thicker peat and taller, denser vegetation can serve to retain sediments and, thus, reduce the load of suspended sediments to this pond. Also, Serene Pond is small (0.45 ha), yet the second deepest (1.0 m). These features predispose Serene Pond to be less vulnerable to sediment resuspension by wind. The bottom of Coast Lake consists mainly of flat cobbles and larger sized rocks, and accumulation of finer-grained sediments occurs only in the spaces between the rocks and in the central deepest part of the lake (∼1 m). This differs from the other study ponds, where organic-rich, fine-grained sediments dominate the bottom surface, and suggests that sediment supply from the catchment is particularly low at Coast Lake. Homer Pond is deeper than all other study ponds with comparably small surface area, and it possesses markedly deeper and steeper banks along the shoreline, thus reducing the frequency of resuspension of bottom sediments into the water column by wind. Because the magnitude of waterchemistry variation was lowest for ponds in SWCT4, as illustrated by PCA (), they are likely the least responsive to seasonal hydrological changes.
The 20 shallow ponds included in our exploratory study were accessible by road from the Churchill Northern Studies Centre and were selected to be representative of many other ponds in the northwestern HBL based on information in Macrae et al. (Citation2004). However, larger datasets are likely needed to capture the full range of hydro-limnological conditions that exist among the ponds in the HBL. While we identified four distinct patterns of waterchemistry variation that appear to correspond with differences in hydrological connectivity to the terrestrial landscape (disconnected, intermittently connected, continuously connected) and pond morphometry, other patterns of hydro-limnological variation may exist. Future studies of longer duration and more frequent sampling are required to better assess seasonal and interannual variability of hydro-limnological relations.
Conclusions
This exploratory study, which tracked hydrological and limnological conditions at 20 shallow ponds in northwestern HBL over the course of the ice-free season in 2010, identified that they followed one of four distinct temporal patterns of variation in water chemistry (SWCT1–4). Based on hydrological evidence provided by water isotope tracers, water-level loggers, and oblique aerial photos at 3 of the ponds, differences in apparent hydrologic connectivity between ponds and the surrounding terrestrial landscape correspond well with the different patterns of water-chemistry variation. For most ponds that lacked hydrologic connectivity with the catchment (i.e., most ponds in SWCT1), alkalinity and ionic content of pond water appears to vary in concert with evaporative concentration during periods of moisture deficit, with little to no change in the concentration of total nitrogen (TKN, TN) and suspended sediment. In contrast, when ponds possess active hydrological connection to the surrounding terrestrial landscape, their waters have relatively higher concentrations of suspended sediment and total nitrogen due to lateral water flow that transfers allochthonous materials from the catchment. SWCT2 ponds appear to be those that temporarily establish hydrological connections during periods of high water levels and high flow conditions (e.g., following snowmelt), but which subsequently lose these connections when rates of water supply decline sufficiently (e.g., during a period without sufficient rainfall in summer and following winters with low snow accumulation). Loss of hydrological connection results in a marked decline of suspended sediment and TKN content of pond water. Ponds that maintain active hydrological connections during all, or at least most, of the ice-free season are expected to display water-chemistry changes associated with SWCT3, characterized by relatively high and constant concentrations of suspended sediment and total nitrogen throughout the ice-free season. Local differences in topographic features of the pond's basin and catchment likely distinguish ponds in SWCT2 and SWCT3. Ponds in SWCT3 likely have deeper channel fens and larger catchment area than ponds in SWCT2, increasing their ability to sustain water levels and maintain hydrological connections in the face of seasonal variations in precipitation and evaporation. Ponds in SWCT4 are relatively deep and possess local features that reduce incidence of wind-induced sediment resuspension in the water column (e.g., cobble bottom at Coast Lake, tall and dense terrestrial vegetation at Serene Pond) and have less variable water chemistry (e.g., greater “inertia” due to their larger water volume). Thus, basin morphometry and catchment vegetation also appear to exert an important influence on seasonal water-chemistry variability.
The apparent role of hydrological connectivity between the ponds and the surrounding terrestrial landscape provides a starting point to anticipate how limnological conditions may respond to continued climate warming. Based on paleolimnological evidence from Larch Pond (CitationWolfe et al., 2011), rising temperatures may promote development of channel fens that connect closed-drainage ponds to their catchments (i.e., via elevated rates of permafrost thaw), causing them to switch from hydro-limnological conditions typical of ponds in SWCT1 to those of SWCT2. In contrast, a scenario of greater evaporation due to longer ice-free season duration may convert ponds in SWCT3 to conditions more typical of ponds in SWCT2. This change can occur due to declining water levels in channel fens that convert continuous hydrological connections to discontinuous connections. Also, declining water levels in SWCT3 ponds can increase frequency of wind-driven sediment resuspension into the water column. Conversion of ponds in SWCT3 to SWCT2 could result in a net decline in pond productivity because pondwater concentrations of total nitrogen and Chl-a appear to decline when hydrological connections become reduced. Closed-drainage ponds in SWCT1 will be the most vulnerable to desiccation and associated aquatic habitat loss, because they lack water “subsidies” provided by the terrestrial landscape. In contrast, the continuously connected ponds of SWCT3 and relatively deep ponds of SWCT4 are likely to be least affected by climate warming due to greater supply of inflow (SWCT3) and larger volume of water (SWCT4) that appear to dampen the magnitude of water-chemistry variations. We suggest that integrated hydro-limnological studies are needed at a larger number of ponds spanning a broader ecological gradient and over a longer study period to more adequately characterize the range of seasonal water-chemistry behavior in ponds of the HBL. In this regard, our research team is undertaking spatial surveys of 40 ponds in nearby Wapusk National Park over a three-year period to characterize interannual variability and better anticipate future climate-driven changes in hydrological and limnological conditions.
Acknowledgments
Funding for this study was provided by the Natural Sciences and Engineering Research Council of Canada (NSERC) via Discovery Grant/Northern Research Supplement/Northern Research Chair Programs, Aboriginal Affairs and Northern Development Canada's Northern Scientific Training Program, and the Churchill Northern Studies Centre's Northern Research Fund. We thank Lauren MacDonald, Nicole Farquharson, Kaleigh Eichel, and Jon Goetz for their logistical help in conducting the fieldwork, and Lauren MacDonald, Jon Goetz, Gillian Merritt, and Johan Wiklund for help with labwork and analysis involved in this research. Claude Duguay supported the airplane charter that provided aerial photographic evidence of hydrological connections at the study ponds.
References Cited
- Bhiry, N. , Delwaide, A. , Allard, M. , Bégin, Y. , Filion, L , Lavoie, M. , Nozais, C. , Payette, S. , Pienitz, R. , Saulnier-Talbot, E. , and Vincent, W. F. , 2011: Environmental change in the Great Whale River region, Hudson Bay: five decades of multidisciplinary research by Centre d'études nordiques (CEN). Écoscience , 18: 182–203.
- Bos, D. G. , and Pellatt, M. G. , 2012: The water chemistry of shallow ponds around Wapusk National Park of Canada, Hudson Bay Lowlands. Canadian Water Resources Journal , 37: 163–175.
- Boudreau, L. D. , and Rouse, W. R. , 1995: The role of individual terrain units in the water balance of wetland tundra. Climate Research , 5: 31–47.
- Chapin, F. S. , McGuire, A. D. , Randerson, J. , Pielke, R. , Baldocchi, D. , Hobbie, S. E. , Roulet, N. , Eugster, W. , Kasischke, E. , Rastetter, E. B. , Zimov, S.A. , and Running, S. W. , 2000: Arctic and boreal ecosystems of western North America as components of the climate system. Global Change Biology , 6: 211–223.
- Chapman, W. L. , and Walsh, J. E. , 1993: Recent variations of sea ice and air temperature in high latitudes. Bulletin of the American Meteorological Society , 74: 33–47.
- Coplen, T. B. , 1996: New guidelines for reporting stable hydrogen, carbon, and oxygen isotope-ratio data. Geochimica et Cosmochimica Acta , 60: 3359–3360.
- Dredge, L. , 1992: Field Guide to the Churchill Region, Manitoba. Ottawa, Canada: Geological Survey of Canada, Series 53, 52 pp.
- Duguay, C. R. , and Lafleur, P. M. , 2003: Determining depth and ice thickness of shallow sub-Artic lakes using space-borne optical and SAR data. International Journal of Remote Sensing , 24: 475–489.
- Duguay, C. R. , Flato, G. M. , Jeffries, M. O. , Menard, P. , Morris, K. , and Rouse, W. R. , 2003: Ice-cover variability on shallow lakes at high latitudes: model simulations and observations. Hydrological Processes , 17: 3465–3483.
- Dyke, L. D. , and Sladen, W. E. , 2010: Permafrost and peatland evolution in the northern Hudson Bay Lowland, Manitoba. Arctic , 63: 429–441.
- Eichel, K. A. , Macrae, M. L. , Hall, R. I. , Fishback, L. , and Wolfe, B. B. , 2014: Nutrient uptake and responses of planktonic and benthic algal communities from a subarctic pond to experimental nutrient enrichment in microcosms. Arctic, Antarctic, and Alpine Research , 46: 191–205.
- Environment Canada , 2011a: Canadian Climate Normals 1971–2000. <http://www.climate.weatheroffice.gc.ca/climate_normals/index_e.html> (accessed January 2011).
- Environment Canada , 2011b: Climate Data Online. <http://www.climate.weatheroffice.gc.ca/climateData/canada_e.html> (accessed January 2011).
- Epstein, S. , and Mayeda, T. K. , 1953: Variation of O18 content of waters from natural sources, Geochimica et Cosmochimica Acta , 4: 213–224.
- Gagnon, A. S. , and Gough, W. A. , 2005: Climate change scenarios for the Hudson Bay region: an intermodal comparison. Climate Change , 69: 269–297.
- Gough, W. A. , Cornwell, A. R. , and Tsuji, L. J. , 2004: Trends in seasonal sea ice duration in southwestern Hudson Bay. Arctic , 57: 299–305.
- Griffis, T. J. , Rouse, W. R. , and Waddington, J. M. , 2000: Interannual variability of net ecosystem CO2 exchange at a subarctic fen. Global Biogeochemical Cycles , 14: 1109–1121.
- Hamilton, J. D. , Kelly, C. A. , Rudd, J. W. M. , Hesslein, R. H. , and Roulet, N. T. , 1994: Flux to the atmosphere of CH4 and CO2 from wetland ponds on the Hudson Bay Lowlands (HBLs). Journal of Geophysical Research—Atmospheres (1984–2012) , 99: 1495–1510.
- Hochheim, K. P. , and Barber, D. G. , 2010: Atmospheric forcing of sea ice in Hudson Bay during the fall period, 1980–2005. Journal of Geophysical Research , 115: C05009, doi: https://doi.org/http://dx.doi.org/10.1029/2009JC005334.
- Jones, B. M. , Grosse, G. , Arp, C. D. , Jones, M. C. , Walter Anthony, K. M. , and Romanovsky, V. E. , 2011: Modern thermokarst lake dynamics in the continuous permafrost zone, northern Seward Peninsula, Alaska. Journal of Geophysical Research , 116: G00M03, doi: https://doi.org/http://dx.doi.org/10.1029/2011JG001666.
- Light, E. , 2011: Characterizing the contemporary and past hydrological conditions of small ponds near Churchill, Manitoba using isotopic methods. M.Sc. thesis, Department of Geography and Environmental Studies, Wilfrid Laurier University, Waterloo, Ontario, Canada, 164 pp.
- Lind, O.T. , 1974: Handbook of Common Methods in Limnology. St.Louis, Missouri, U.S.A.: C. V. Mosby Company, 103–105.
- Macrae, M. L. , Bello, R. L. , and Molot, L. A. 2004: Long-term carbon storage and hydrological control of CO2 exchange in tundra ponds in the Hudson Bay Lowland. Hydrological Processes , 18: 2051–2069.
- Macrae, M. L. , Brown, L. C. , Duguay, C. R. , Parrott, J. A. , and Petrone, R. M. , 2014: Observed and projected climate change in the Churchill region of the Hudson Bay Lowlands and implications for pond sustainability. Arctic, Antarctic, and Alpine Research , 46: 272–285.
- Mallory, M. L. , Gaston, A. J. , Gilchrist, H. G. , Robertson, G. J. , and Braune, B. M. , 2010: Effects of climate change, altered sea-ice distribution and seasonal phenology on marine birds. In Ferguson, S. H. , Loseto, L. L. , and Mallory, M. L. (eds.), A Little Less Arctic: Top Predators in the World's Largest Northern Inland Sea, Hudson Bay. Dordrecht: Springer, 179–195.
- Morrison, J. , Brockwell, T. , Merren, T. , Fourel, F. , and Phillips, A. M. , 2001: A new on-line method for high precision stable-hydrogen isotopic analyses on nanolitre water samples, Analytical Chemistry , 73: 3570–3575.
- Parsons, T. R. , and Strickland, J. D. H. , 1963: Discussion of spectrophotometric determination of marine-plant pigments with revised equations for ascertaining chlorophylls and carotenoids. Journal of Marine Research , 21: 155–163.
- Payette, S. , Delwaide, A. , Caccianiga, M. , and Beauchemin, M. , 2004: Accelerated thawing of subarctic peatland permafrost over the last 50 years. Geophysical Research Letters , 31: doi: https://doi.org/http://dx.doi.org/10.1029/2004GL020358.
- Prowse, T. , Alfredsen, K. , Beltaos, S. , Bonsal, B. , Duguay, C. , Korhola, A. , McNamara, J. , Pienitz, R. , Vincent, W. F. , Vuglinsky, V. , and Weyhenmeyer, G. A. , 2011: Past and future changes in arctic lake and river ice. Ambio , 40: 53–62.
- Rautio, M. , Dufresne, F. , Laurion, I. , Bonilla, S. , Vincent, W. F. , and Christoffersen, K. S. , 2011: Shallow freshwater ecosystems of the circumpolar Arctic. Écoscience , 18: 204–222.
- Roulet, N. , Moore, T. , Bubier, J. , and Lafleur, P. , 1992: Northern fens: methane flux and climatic change. Tellus B , 44: 100–105.
- Rouse, W. R. , 1991: Impacts of Hudson Bay on the terrestrial climate of the Hudson Bay Lowlands. Arctic and Alpine Research , 23: 24–30.
- Rouse, W. R. , 1998: A water balance model for a subarctic sedge fen and its application to climatic change. Climatic Change , 38: 207–234.
- Rouse, W. R. , Douglas, S. V. , Hecky, R. E. , Hershey, A. E. , Kling, G. W. , Lesack, L. , Marsh, P. , Macdonald, M. , Nicholson, B. J. , Roulet, N. T. , and Smol, J. P. , 1997: Effects of climate change on the freshwaters of arctic and subarctic North America. Hydrological Processes , 11: 873–902.
- Sauchyn, D. , and Kulshreshtha, S. , 2008: Prairies. In Lemmen, D. S. , Warren, F. J. , Lacroix, J. , and Bush, E. , (eds.), From Impacts to Adaptation: Canada in a Changing Climate 2007. Ottawa, Ontario: Government of Canada, 275–328.
- Schindler, D. W. , and Smol, J. P. , 2006: Cumulative effects of climate warming and other human activities on freshwaters of Arctic and subarctic North America. Ambio , 35: 160–168.
- SCOR/UNESCO, 1966: Working Group on Photosynthetic Pigments:Monographs on Oceanographic Methodology. Paris: UNESCO Publishing.
- Smol, J. P. , and Douglas, M. S. V. , 2007: Crossing the final ecological threshold in High Arctic ponds. Proceedings of the National Academy of Sciences , 104: 12395–12397.
- Sokal, M. A. , Hall. R. I. , and Wolfe, B. B. , 2010: Inter-annual and seasonal effects of flooding on the water chemistry, diatom phytoplankton communities and macrophyte biomass of lakes in the Slave River Delta (Northwest Territories, Canada). Ecohydrology , 3: 41–54.
- Stewart, R. B. , and Rouse, W. R. , 1976: A simple method for determining the evaporation from shallow lakes and ponds. Water Resource Research , 12: 623–628.
- Symons, C. C. , Arnott, S. E. , and Sweetman, J. N. , 2012: Nutrient limitation of phytoplankton communities in subarctic lakes and ponds in Wapusk National Park, Canada. Polar Biology , 35: 481–489.
- ter Braak, C. J. F. , and Šmilauer, P. , 2002: CANOCO version 4.5.Wageningen, Netherlands: Biometrics—Plant Research International.
- Turner, K. W. , Wolfe, B. B. , and Edwards, T. W. D. , 2010: Characterizing the role of hydrological processes on lake water balances in the Old Crow Flats, Yukon Territory, Canada, using water isotope tracers, Journal of Hydrology , 386: 103–117.
- Vincent, W. F. , Callaghan, T. V. , Dahl-Jensen, D. , Johansson, M. , Kovacs, K. M. , Michel, C. , Prowse, T. , Reist, J. D. , and Sharp, M. 2011: Ecological implications of changes in the arctic cryosphere. Ambio , 40: 87–99.
- Wiklund, J. A. , Hall, R. I. , and Wolfe, B. B. , 2012: Timescales of hydrolimnological change in floodplain lakes of the Peace-Athabasca Delta, northern Alberta, Canada. Ecohydrology , 5: 351–367.
- Wolfe, B. B. , Light, E. M. , Macrae, M. L. , Hall, R. I. , Eichel, K. , Jasechko, S. , White, J. , Fishback, L. , and Edwards, T. W. D. , 2011: Divergent hydrological responses to 20th century climate change in shallow tundra lakes, western Hudson Bay Lowlands. Geophysical Research Letters , 38: L23402, doi: https://doi.org/http://dx.doi.org/10.1029/2011GL049766.
- Woo, M. K. , and Winter, T. C. , 1993. The role of permafrost and seasonal frost in the hydrology of northern wetlands in North America. Journal of Hydrology , 141: 5–31.
- Woo, M. K. , Lewkowicz, A. G. , and Rouse, W. R. , 1992: Response of the Canadian permafrost environment to climatic change. Physical Geography , 13: 287–317.
Appendix
TABLE A1 Values for the limnological variables measured in early June 2010 at the 20 study ponds located in the Churchill Wildlife Management Area. The ponds are arranged according to their surface water-chemistry trajectory (SWCT) category (see text for details).
TABLE A2 Values for the limnological variables measured in late July 2010 at the 20 study ponds located in the Churchill Wildlife Management Area. The ponds are arranged according to their surface water-chemistry trajectory (SWCT) category (see text for details).
TABLE A3 Values for the limnological variables measured in mid-September 2010 at the 20 study ponds located in the Churchill Wildlife Management Area. The ponds are arranged according to their surface water-chemistry trajectory (SWCT) category (see text for details).