Abstract
Biscarbamate cross-linked polyethylenimine derivative (PEI-Et) has been reported as a novel nonviral vector for efficient and safe gene transfer in our previous work. However, it had no cell-specificity. To achieve specific delivery of genes to hepatocytes, galactosylated poly(ethylene glycol)-graft-polyethylenimine derivative (GPE) was prepared through modification of PEI-Et with poly(ethylene glycol) and lactobionic acid, bearing a galactose group as a hepatocyte-targeting moiety. The composition of GPE was characterized by proton nuclear magnetic resonance. The weight-average molecular weight of GPE measured with a gel permeation chromatography instrument was 9489 Da, with a polydispersity of 1.44. GPE could effectively condense plasmid DNA (pDNA) into nanoparticles. Gel retardation assay showed that GPE/pDNA complexes were completely formed at weigh ratios (w/w) over 3. The particle size of GPE/pDNA complexes was 79–100 nm and zeta potential was 6–15 mV, values which were appropriate for cellular uptake. The morphology of GPE/pDNA complexes under atomic force microscopy appeared spherical and uniform in size, with diameters of 53–65 nm. GPE displayed much higher transfection efficiency than commercially available PEI 25 kDa in BRL-3A cell lines. Importantly, GPE showed good hepatocyte specificity. Also, the polymer exhibited significantly lower cytotoxicity compared to PEI 25 kDa at the same concentration or weight ratio in BRL-3A cell lines. To sum up, our results indicated that GPE might carry great potential in safe and efficient hepatocyte-targeting gene delivery.
Introduction
The curing effect of gene therapy greatly depends on safe and efficient delivery of therapeutic gene to the target site.Citation1,Citation2 In recent years, nonviral vectors have been investigated intensively for gene delivery, due to their ease of production and chemical modification, safety, lower immune response, and the capacity to delivery larger DNA molecules.Citation3–Citation5 Consequently, alternative gene carriers have been proposed based on nonviral vectors, such as cationic lipidsCitation6,Citation7 and cationic polymers.Citation8–Citation13 However, there are still many obstacles that can hamper the delivery capability of nonviral carriers in vivo.Citation14 For example, without modification with an appropriate targeting moiety, the carrier will have an increased risk of entering undesired cells and possibly cause damage to healthy tissues. Therefore, targeted transfer of nucleic acid drugs to specific tissues is a significantly important concern to address in the field of gene delivery.
Receptor-mediated endocytosis has been shown to be a promising way to achieve specific delivery of genes to certain cell types or tissues. Surface modification of nanosized vectors like nanoparticles is usually used for specific targeting purposes. Various ligands, including antibody,Citation15 folate,Citation16,Citation17 asialoglycoprotein,Citation18 galactose,Citation19,Citation20 mannose,Citation21 epidermal growth factor,Citation22 and transferrinCitation23 have been conjugated with nonviral carriers for cell specificity. It was reported that the asialoglycoprotein receptor (ASGPR) was abundantly expressed in normal hepatocytes and hepatoma cell lines, such as BRL-3A, HepG2, and parental human hepatocellular carcinoma BEL-7402 cells. There are on average 500,000 ASGPRs on every hepatocyte. ASGPR can selectively bind to galactose or N-acetylgalactosamine residues of desialylated glycoproteins.Citation24 ASGPR has received much attraction in gene targeting and has also acted as a model system for studying receptor-mediated endocytosis due to its high affinity and rapid internalization rate. Therefore, the delivery of genes to hepatocytes through ASGPR-mediated endocytosis using galactosylated polymers has gained significant interest. For instance, as Gref et alCitation25 reported, galactose-modified oligosaccharides displayed a high affinity for ASGPR in liver tumor cells. Gao et alCitation26 reported a gene carrier based on galactosylated chitosans that showed obvious targeting in hepatoma cells HepG2, SMMC-7721, and normal hepatic cell L-02. Kim et alCitation27 conjugated galactose to poly(ethylene) glycol (PEG)–polyethylenimine (PEI) to obtain a hepatocyte-targeting gene carrier; the polymer they synthesized exhibited improved transfection efficiency in hepatoma cells.
In our previous work, we synthesized biscarbamate cross-linked PEI derivative (PEI-Et) as a nonviral gene carrier. Our results showed that PEI-Et displayed significantly enhanced transfection efficiency and much lower cytotoxicity than commercially available PEI 25 kDa in three cell lines (COS-7, BRL-3A, and HeLa).Citation28 However, the polymer had no cell-specificity. Therefore, in the present study, galactosylated PEG-graft-PEI derivative (GPE) was prepared to achieve hepatocyte specificity. Two chemical modifiers, PEG and galactose, were included in GPE. Galactose was acted as a hepatocyte-targeting moiety. PEG modification promoted the formation of complexes with diminished aggregation, and reduced opsonization with serum proteins in the bloodstream.Citation29 Furthermore, PEGylation provided a polyplex with improved solubility, lower cytotoxicity, and longer circulation time in vivo.Citation30 In this paper, the synthesized GPE was characterized with proton nuclear magnetic resonance (1H-NMR) and GPC. GPE/plasmid DNA (pDNA) complexes were prepared and investigated by particle size, zeta potential, gel retardation ability, and morphology under atomic force microscopy (AFM). Moreover, cytotoxicities of GPE and GPE/pDNA complexes were examined in terms of cell viability, and transfection efficiencies as well as hepatocyte specificity of GPE/pDNA complexes were examined with luciferase activity assay, fluorescence microscopy, and fluorescence-activated cell-sorting analysis (FACS).
Materials and methods
Materials
Branched PEI (25 kDa), lactobionic acid (LA), ethidium bromide, (EB) and 3-(4,5-dimethylthiazol-2-yl)-2,5-diphenyltetrazoliumbromide (MTT) were sourced from Sigma-Aldrich (St Louis, MO, USA). PEI-Et was synthesized in our laboratory. The heterobifunctional PEG derivative NH2-PEG-COOH (molecular weight [MW] 2000 Da) and methoxy-PEG-succinimidyl carbonate (mPEG-sc; MW 2000 Da) were purchased from Yarebio (Shanghai, People’s Republic of China). A Micro BCA protein assay kit was purchased from Thermo Fisher Scientific (Waltham, MA, USA). A luciferase assay kit was purchased from Promega (Fitchburg, WI, USA). All other chemicals used were of analytical grade.
Trypsin ethylenediaminetetraacetic acid, Dulbecco’s modified Eagle’s medium (DMEM), and fetal bovine serum were sourced from PAA (Cölbe, Germany). The plasmids were pEGFP-N1 (Clontech, Palo Alto, CA, USA), encoding enhanced green fluorescent protein (EGFP), and pGL3-control (Promega), encoding firefly luciferase.
Cell culture
Normal rat liver cell (BRL-3A) and human cervix epithelial carcinoma cell (HeLa) were incubated in DMEM medium containing 10% fetal bovine serum at 37°C in a humidified atmosphere supplemented with 5% CO2.
Synthesis of GPE
The polymer GPE was synthesized in two steps. In the first step, galactosylated PEG (Gal-PEG) was prepared by an amide-formation reaction between activated carboxyl groups of galactose bearing LA and amine groups of NH2-PEG-COOH in accordance with a previous report,Citation31 with some changes. Briefly, LA (1.5 mmol) dissolved in 30 mL of 2-(N-morpholino)ethanesulfonic acid (MES) buffer solution (0.1 M, pH 6.5) was activated with a mixture of N-hydroxysuccinimide (NHS) (6 mmol) and 1-ethyl-3-(3-dimethylaminopropyl)-carbodiimide hydrochloride (EDC) (6 mmol). After activating the carboxyl groups for 30 minutes, 0.075 mmol of PEG was added. The reaction was performed in an ice bath for 12 hours, followed by an additional 12 hours at room temperature. Then the sample was dialyzed against distilled water in a dialysis tube (MW cutoff 1000 Da) for 3 days, followed by lyophilization. The resulting polymer Gal-PEG was stored at −20°C for further use.
In the second step, GPE was synthesized by an amide-formation reaction between activated carboxyl groups of Gal-PEG and amine groups of PEI-Et. PEI-Et was synthesized according to our previous study.Citation28 Gal-PEG (0.02 mmol) dissolved in 10 ml of MES buffer solution (0.1 M, pH 6.5) was activated with a mixture of NHS (0.2 mmol) and EDC (0.2 mmol). After activating the carboxyl groups for 30 minutes, 0.02 mmol of PEI-Et was added. The reaction was performed in an ice bath for 12 hours, followed by an additional 12 hours at room temperature. Then the sample was dialyzed against distilled water in a dialysis tube (MW cutoff 3500 Da) for 3 days and lyophilized to obtain the polymer GPE. The reaction scheme is shown in .
Synthesis of PEG-Et
PEI-Et (0.04 mmol) was dissolved in 0.1 M sodium bicarbonate, followed by the addition of 0.04 mmol of mPEG-Sc and stirred for 4 hours at room temperature. The resultant PEG-Et was dialyzed against distilled water in a dialysis tube (MW cutoff 3500 Da) for 2 days, followed by lyophilization. The resulting polymer PEG-Et was stored at −20°C for further use.
Characterization of GPE
1H-NMR spectra of GPE were recorded on a Varian Unity 300 MHz spectrometer (Mercury plus 400; Varian, Palo Alto, CA, USA), using D2O as a solvent. GPC relative to PEG standards (molecular weight range Mp 106, 430, 633, 1400, 4290, 7130, 12,600, 20,600 Da) was used to measure the MW of GPE by a Waters (Milford, MA, USA) high-pressure liquid chromatography (HPLC) system. The mobile phase of HPLC was formic acid.
Preparation of polymer/pDNA complexes
Both pDNA and polymer were separately diluted to the required concentration in phosphate-buffered saline (PBS; pH 7.4). After that, the polymer/pDNA complexes were prepared by adding polymer solution to the pDNA solution at the desired weight ratio with gentle vortexing. The polymer/pDNA complexes were incubated at room temperature for 30 minutes prior to use.
Gel retardation assay
The gel retardation ability of GPE was evaluated using agarose gel electrophoresis with pEGFP-N1. GPE and pDNA solutions were mixed at various weight ratios from 1 to 70 and incubated for 30 minutes at room temperature. The complexes and naked pDNA were electrophoresed on 1% (w/v) agarose gels pretreated with EB (0.5 μg/mL of the gel) in 1 × Tris-acetate buffer at 80 V for 40 minutes.
Particle-size and zeta-potential measurements
A particle-size analyzer (90Plus; Brookhaven Instruments, Holtsville, NY, USA) was used to examine the particle size and zeta potential of GPE/pDNA complexes. GPE/pDNA complexes at various w/w ratios from 1 to 70 were prepared and incubated for 30 minutes at room temperature before measurement. Each sample was performed in triplicate.
Atomic force microscopy
The morphology of GPE/pDNA complexes at w/w 70 was examined under AFM (E-Sweep; Hitachi High-Tech Science, Tokyo, Japan). The complexes were deposited on a mica disk and dried for 3 hours at room temperature. Then it was observed under AFM.
Cytotoxicity assay
Cytotoxicity evaluation of the polymer was measured with MTT assay. PEI 25 kDa was used as a control. BRL-3A cells were grown in 96-well plates at an initial density of 5000 cells/well in 100 μL of DMEM and incubated for 24 hours. After that, the media were changed with fresh serum-free DMEM pretreated with polymers at various concentrations (5, 10, 20, 50, and 100 μg/mL) or polymer/pDNA complexes at various w/w ratios (2, 5, 10, 20, 30, and 50). After further incubation for 4 hours, the media were replaced with fresh serum-free DMEM, and 25 μL MTT solution (5 mg/mL in PBS) was added per well. After an additional incubation for 6 hours, 150 μL of DDDT was added. Then the plate was agitated for 15 minutes. Finally, the absorbance was recorded with an enzyme-linked immunosorbent assay reader (MK3; Thermo Fisher Scientific) at 570 nm (with 630 nm as a reference wavelength). The data from five separate experiments were expressed as a percentage of viable cells over untreated control.
In vitro transfection experiments
The transfections mediated by GPE were performed in BRL-3A and HeLa cells. Cells were grown in 48-well plates at an initial density of 5 × 104 cells/well in 500 μL of DMEM and incubated for 24 hours. After that, wells were washed with PBS, and polymer/pGL3-control (500 ng) complexes at desired w/w ratios were added to the cells. After an additional incubation for 4 hours, the media were replaced with fresh and complete DMEM and allowed to incubate for 44 hours. Luciferase assays were performed according to the manufacturer’s suggested protocol (Promega). The luciferase activity was expressed in terms of relative light units/mg protein. Each sample was performed in triplicate. The optimal w/w ratio of GPE/pEGFP-N1 complexes from the luciferase activity assay was selected for the GFP-expression experiment. The transfection efficiency was estimated by scoring the percentage of cells expressing GFP using a FACSCalibur system (BD, Franklin Lakes, NJ, USA). Each sample was performed in triplicate. The data were presented as means ± standard deviation. For the competition assay, BRL-3A cells were preincubated with galactose (1, 10, and 100 mM) for 15 minutes, then the cells were incubated with GPE/pDNA and PEG-Et/pDNA complexes for 3 hours. The luciferase activity was determined as described above after 45 hours’ further incubation.Citation28
Statistical analysis
Data were expressed as means ± standard deviation. Statistical analysis was performed with SPSS software (v 19.0; IBM, Armonk, NY, USA). Student’s t-test (two-tailed) was used to test the significance of the differences between two groups. Data were considered significantly different at the level of P < 0.05 and very significantly different at the level of P < 0.01.
Results and discussion
GPE was successfully synthesized
schematically illustrates the procedures for the synthesis of GPE. The intermediate Gal-PEG was synthesized by an amide-formation reaction between activated carboxyl groups of LA and amine groups of NH2-PEG-COOH, and the resulting polymer GPE was synthesized by an amide-formation reaction between activated carboxyl groups of Gal-PEG and amine groups of PEI-Et. The structure of GPE was confirmed using 1H-NMR. As shown in , the proton peaks appeared at 2.4–3.3 ppm in the GPE attributed to PEI (–NHCH2CH2–), indicating that PEI-Et was successfully conjugated to the Gal-PEG chain. The weight-average MW of GPE measured with GPC was 9489 Da, with a polydispersity of 1.44. These results indicated that GPE was successfully synthesized.
Figure 2 Representative proton nuclear magnetic resonance spectra of galactosylated poly(ethylene glycol)-graft-polyethylenimine derivative (GPE).
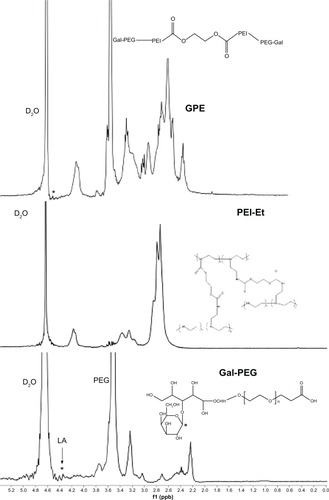
Characterization of GPE/pDNA complexes was appropriate for cellular uptake
As for cationic polymers, the condensation of pDNA into small particles is an important prerequisite for gene delivery.Citation32 The gel retardation ability of GPE was measured with agarose gel electrophoresis. Naked pDNA was used as the control group. As indicated in , GPE completely retarded the migration of pDNA when the w/w ratio was 3, suggesting that GPE/pDNA complexes were completely formed at w/w ratios over 3. Interaction of cationic polymers with nucleic acid could protect the nucleic acid from enzymatic degradation,Citation33,Citation34 which facilitated efficient gene transfection.
Figure 3 Agarose gel electrophoresis of galactosylated poly(ethylene glycol)-graft-polyethylenimine derivative/plasmid DNA (pDNA) complexes at various w/w ratios.
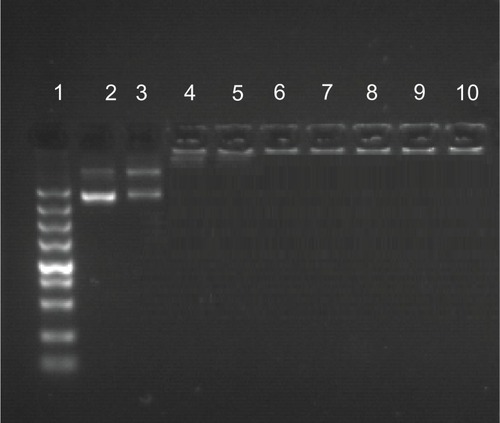
The particle size of the polymer/pDNA complexes was an important factor for hepatocyte gene delivery. As Hashida et al mentioned, the majority of the fenestrate of the liver sinusoid was smaller than 200 nm in diameter.Citation35 Therefore, it was hard for large particles to arrive at the parenchymal cells of the liver. In addition, gene carriers with diameters larger than 200 nm are readily scavenged nonspecifically by monocytes and the reticuloendothelial system.Citation36 A positive surface charge of GPE, which comes from the protonated amino groups on PEI, may be an advantage for cellular uptake, due to the electrostatic interaction between the negatively charged cellular membrane and the positively charged complexes.Citation37,Citation38 As shown in , at a w/w ratio of 1, the particle size of GPE/pDNA complexes was 108 nm and the zeta potential was −8.9 mV, indicating that the complexation between GPE and pDNA was incomplete. However, when the w/w ratios were over 5, GPE could condense pDNA into nanoparticles with relatively constant diameters of 79–100 nm, implying that stable complexes were formed with a size appropriate for cellular uptake. Meanwhile, zeta potential ranged from 6 mV to 15 mV. These results accorded well with the results of the gel retardation assay.
Figure 4 Particle size (A) and zeta potential (B) of galactosylated poly(ethylene glycol)-graft-polyethylenimine derivative/plasmid DNA complexes, as determined by dynamic light scattering at various w/w ratios.
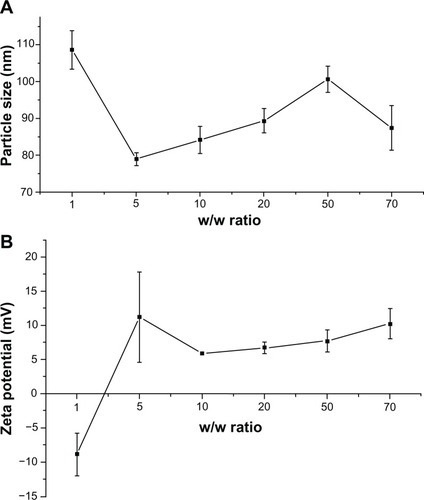
The representative morphologies of GPE/pDNA complexes (w/w 70) under AFM are shown in . The results show that the complexes appeared spherical in shape with compact structure, and the diameters of the complexes ranged from 53 nm to 65 nm, smaller than those determined by dynamic light scattering. This phenomenon was possibly due to the shrinkage of the PEG shell caused by the evaporation of water during drying before AFM examination.Citation39
GPE showed low cytotoxicity in BRL-3A cells
For polycationic gene carriers, cytotoxicity was a main hurdle for clinical application.Citation40 The cytotoxicity associated with GPE could be divided into two types: the immediate toxicity mediated by free GPE, and the delayed toxicity mediated by GPE/pDNA complexes. For this reason, cell viabilities of free GPE and GPE/pDNA complexes were assayed using BRL-3A cells. Free polymers were used in order to mimic a worst-case scenario and get much larger sensitivity results, because cytotoxicity was remarkably reduced with formation of polymer/pDNA complexes.Citation41
As shown in , cytotoxicity of GPE was much lower than PEI 25 kDa at the same concentration. In addition, GPE displayed negligible cytotoxicity at concentrations below 100 μg/mL. The cell viabilities were 104% ± 7% at a polymer concentration of 5 μg/mL. The value slightly decreased to 95% ± 6% with GPE concentration increasing to 100 μg/mL, implying that a wide dose range of GPE may be used for gene transfection. In contrast, with increasing concentrations of PEI 25 kDa, cell viability decreased drastically. For example, cell viability was from 88% ± 3% at a PEI 25 kDa concentration of 5 μg/mL to 23% ± 1% at a PEI 25 kDa concentration of 100 μg/mL. In the case of the cell viabilities of polymer/pDNA complexes, GPE/pDNA complexes also showed dramatically lower cytotoxicity than PEI 25 kDa/pDNA complexes. These results suggested that the immediate toxicity and the delayed toxicity of GPE were all lower than that of PEI 25 kDa, which demonstrated that GPE was a significantly promising carrier for safe gene transfer.
Figure 6 Cytotoxicity of the polymers at various concentrations (A) and cytotoxicity of the polymer/plasmid DNA complexes at various w/w ratios (B) in BRL-3A cell lines.
Abbreviations: GPE, galactosylated poly(ethylene glycol)-graft-polyethylenimine derivative; PEI, polyethylenimine.
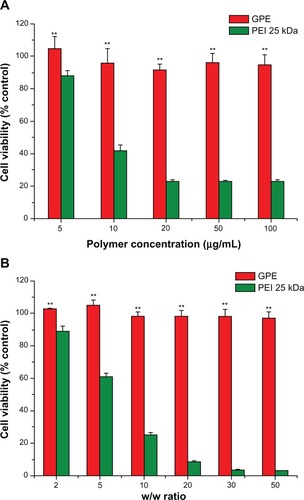
According to previous studies, chemical modification of PEI with PEG could help reduce the cytotoxicity by reducing the number of PEI amino groups.Citation42,Citation43 Therefore, GPE with much lower cytotoxicity than PEI 25 kDa was probably due to the properties of the hydrophilic groups of PEG. In addition, Bieber and Elsässer reported that a positive correlation existed between MW and cytotoxicity of PEI: cytotoxicity of PEI with low MW was much lower than PEI with high MW.Citation44 For this reason, the lower molecular weight of GPE was another factor that produced lower cytotoxicity than PEI 25 kDa.
GPE exhibited high transfection efficiency and good hepatocyte specificity in BRL-3A cells
To observe the in vitro transfection efficiency and hepatocyte specificity of GPE, BRL-3A and HeLa cells were transfected with polymer/pDNA complexes with various w/w ratios. PEI 25 kDa at optimal w/w ratio was used as a positive control. As illustrated in , the transfection efficiency was dependent on the GPE/pDNA weight ratio. Transfection efficiency of GPE increased with increasing w/w ratios below 70, and then decreased at higher w/w ratios. A reasonable explanation may be as follows: a low w/w ratio would produce unstable complexes and low transfection efficiency; however, a high w/w ratio yielded low transfection efficiency due to the stability, because the pDNA could not be released from the complexes.Citation28 In addition, the transfection efficiency of GPE was higher than that of PEI 25 kDa at w/w from 30 to 70 (P < 0.05). As for the naked pDNA, it produced almost negligible luciferase activity, indicating that pDNA without any vector showed significantly low transfection efficiency, which was in agreement with the previous study.Citation45,Citation46 As illustrated in , transfection efficiency of GPE was 4.6-fold higher than that of PEG-Et at a w/w ratio of 70 in BRL-3A cells (P < 0.01). Moreover, GPE showed a 13.2-fold higher transfection efficiency in BRL-3A cells in comparison to HeLa cells (P < 0.01), which did not express ASGPR, suggesting that the attachment of galactose residues in GPE might be beneficial for the recognition of ASGPR and lead to the significant improvement of transfection efficiency in BRL-3A cells.
Figure 7 (A) Transfection efficiency of GPE/pGL3-control complexes at various w/w ratios in BRL-3A cell lines, in comparison with that of polyethylenimine 25 kDa (w/w 2). (B) Transfection efficiency of galactosylated poly(ethylene glycol)-graft-polyethylenimine derivative (GPE)/pGL3-control and poly(ethylene) glycol (PEG)-Et/pGL3-control complexes prepared at a w/w ratio of 70 in HeLa and BRL-3A cells.
Abbreviation: RLU, relative light units.
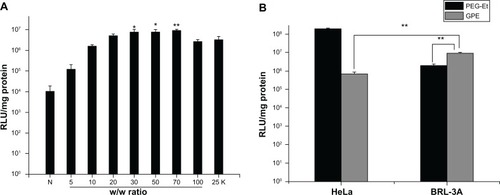
To confirm the hepatocyte specificity of GPE, gene-transfection efficiency was evaluated in BRL-3A and HeLa cells, using pEGFP-N1 as a reporter gene. displays typical fluorescence microscope images; BRL-3A cells transfected with GPE/pEGFP-N1 showed more bright fluorescent spots than PEG-Et/pEGFP-N1. In addition, transfection efficiency was monitored by flow cytometry. As shown in , GPE exhibited higher efficiency (33%) than PEG-Et (27%) in BRL-3A cells (P < 0.01). Also, transfection efficiency of GPE was higher in BRL-3A cells (33%) than in HeLa cells (26%) (P < 0.01). These results obtained also confirmed the results of luciferase activity assays, implying that GPE showed good hepatocyte specificity.
Figure 8 Typical fluorescence images (magnification 100×) (A) and flow cytometry analyzed graphs (B) of cell distribution expressing GFP of BRL-3A and HeLa cells transfected with PEG-Et/pEGFP-N1 (a and c), and GPE/pEGFP-N1(b and d) at w/w 70, and (C) transfection efficiency of PEG-Et and GPE as a percentage of EGFP positive cells per total amount of cells.
Abbreviations: PEG, poly(ethylene glycol); GPE, galactosylated poly(ethylene glycol)-graft-polyethylenimine derivative; pEGFP, plasmid enhanced green fluorescent protein.
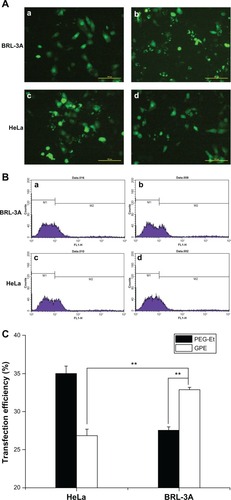
To confirm further the effect of galactose on receptor-mediated gene delivery, the competition assay was performed at the presence of free galactose (1, 10, and 100 mM) as a competitor. shows that the transfection efficiency of GPE in BRL-3A cells was reduced in the presence of free galactose. Especially, inhibition of the transfection efficiency of GPE in the presence of galactose depended on concentration of pretreated galactose, whereas the phenomenon was not observed on the transfection efficiency of PEG-Et. Transfection efficiency when using PEG-Et as a carrier was very low and was not affected irrespective of the addition of free galactose. These results indicated that pretreatment of free galactose as a competitor could reduce cellular uptake of GPE by competitive binding to ASGPR on the cell surface, although the inhibition of transfection efficiency was incomplete in the competition assay, because GPE still entered into BRL-3A cells via both nonspecific endocytosis and receptor-mediated endocytosis.
Figure 9 Competition assay of GPE/pGL3-control complexes on BRL-3A cells prepared at a w/w ratio of 70 by adding free galactose (1, 10, and 100 mM) as a competitor of the galactose functional group in GPE.
Abbreviations: PEG, poly(ethylene glycol); GPE, galactosylated poly(ethylene glycol)-graft-polyethylenimine derivative; RLU, relative light units.
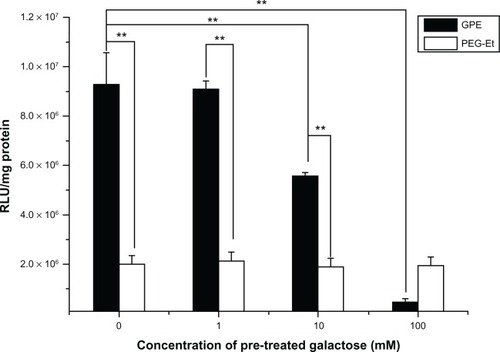
Conclusion
In the current study, a novel hepatocyte-targeting gene carrier, GPE, was successfully prepared. The polymer was constructed by a simple procedure, possessed a enhanced ability to condense pDNA effectively into nanoparticles with physicochemical properties appropriate for cellular uptake. GPE displayed significantly higher transfection efficiency and much lower cytotoxicity than commercially available PEI 25 kDa in BRL-3A cells. Importantly, GPE exhibited good hepatocyte specificity. To sum up, it is reasonable to conclude that GPE might carry potential for efficient and safe hepatocyte-targeting gene delivery.
Acknowledgemnts
This study was supported by grants from the National Natural Science Foundation of China (81001416 and 81270205), the Shanghai Science and Technology Committee, People’s Republic of China (10JC1408902), and the Research Fund for Integrated Medicine and Engineering of Shanghai Jiao Tong University (YG2011MS21).
Disclosure
The authors report no conflicts of interest in this work.
References
- Srinivas R Samanta S Chaudhuri A Cationic amphiphiles: promising carriers of genetic materials in gene therapy Chem Soc Rev 2009 38 3326 3338 20449052
- Park TG Jeong JH Kim SW Current status of polymeric gene delivery systems Adv Drug Deliv Rev 2006 58 467 486 16781003
- Niidome T Urakawa M Sato H Gene transfer into hepatoma cells mediated by galactose-modified alpha-helical peptides Biomaterials 2000 21 1811 1819 10905464
- Mintzer MA Simanek EE Nonviral vectors for gene delivery Chem Rev 2009 109 259 302 19053809
- Yu H Wagner E Bioresponsive polymers for nonviral gene delivery Curr Opin Mol Ther 2009 11 165 178 19330722
- Heinze M Brezesinski G Dobner B Langner A Novel cationic lipids based on malonic acid amides backbone: transfection efficiency and cell toxicity properties Bioconjug Chem 2010 21 696 708 20329785
- Yang XZ Dou S Sun TM Mao CQ Wang HX Wang J Systemic delivery of siRNA with cationic lipid assisted PEG-PLA nanoparticles for cancer therapy J Control Release 2011 156 203 211 21839126
- Sato T Ishii T Okahata Y In vitro gene delivery mediated by chitosan. effect of pH, serum, and molecular mass of chitosan on the transfection efficiency Biomaterials 2001 22 2075 2080 11432586
- Fischer D Li Y Ahlemeyer B Krieglstein J Kissel T In vitro cytotoxicity testing of polycations: influence of polymer structure on cell viability and hemolysis Biomaterials 2003 24 1121 1131 12527253
- Dong X Tian H Chen L Chen J Chen X Biodegradable mPEG-b-P(MCC-g-OEI) copolymers for efficient gene delivery J Control Release 2011 152 135 142 21457739
- Sun TM Du JZ Yan LF Mao HQ Wang J Self-assembled biodegradable micellar nanoparticles of amphiphilic and cationic block copolymer for siRNA delivery Biomaterials 2008 29 4348 4355 18715636
- Eliyahu H Joseph A Azzam T Barenholz Y Domb AJ Dextran-spermine-based polyplexes – evaluation of transgene expression and of local and systemic toxicity in mice Biomaterials 2006 27 1636 1645 16221492
- Xia J Tian H Chen L Oligoethylenimines grafted to PEGy-lated poly(β-amino ester)s for gene delivery Biomacromolecules 2011 12 1024 1031 21355539
- Kaneda Y Gene therapy: a battle against biological barriers Curr Mol Med 2001 1 493 499 11899093
- Jeong JH Lee M Kim WJ Anti-GAD antibody targeted non-viral gene delivery to islet beta cells J Control Release 2005 107 562 570 16139384
- Mansouri S Cuie Y Winnik F Characterization of folate-chitosan-DNA nanoparticles for gene therapy Biomaterials 2006 27 2060 2065 16202449
- Cho KC Jeong JH Chung HJ Joe CO Kim SW Park TG Folate receptor-mediated intracellular delivery of recombinant caspase-3 for inducing apoptosis J Control Release 2005 108 121 131 16139916
- Hashimoto M Morimoto M Saimoto H Shigemasa Y Sato T Lactosylated chitosan for DNA delivery into hepatocytes: the effect of lactosylation on the physicochemical properties and intracellular trafficking of pDNA/chitosan complexes Bioconjug Chem 2006 17 309 316 16536460
- Cook SE Park IK Kim EM Galactosylated polyethylenimine-graft-poly(vinyl pyrorolidone) as a hepatocytetargeting gene carrier J Control Release 2005 105 151 163 15878633
- Kim TH Park IK Nah JW Choi YJ Cho CS Galactosylated chitosan/DNA nanoparticles prepared using water-soluble chitosan as a gene carrier Biomaterials 2004 25 3783 3792 15020154
- Hashimoto M Morimoto M Saimoto H Gene transfer by DNA/mannosylated chitosan complexes into mouse peritoneal macrophages Biotechnol Lett 2006 28 815 821 16786247
- Lee H Kim TH Park TG A receptor-mediated gene delivery system using streptavidin and biotin-derivatized, pegylated epidermal growth factor J Control Release 2002 83 109 119 12220843
- Sakaguchi N Kojima C Harada A Enhancement of transfection activity of lipoplexes by complexation with transferrin-bearing fusogenic polymer-modified liposomes Int J Pharm 2006 325 186 190 16844328
- Schwartz AL Fridovich SE Knowles BB Lodish HF Characterization of the asialoglycoprotein receptor in a continuous hepatoma line J Biol Chem 1981 256 8878 8881 6267054
- Gref R Rodrigues J Couvreur P Polysaccharides grafted with poly-esters: novel amphiphilic copolymers for biomedical applications Macromolecules 2002 35 9861 9867
- Gao S Chen J Xu X Galactosylated low molecular weight chitosan as DNA carrier for hepatocyte-targeting Int J Pharm 2003 255 57 68 12672602
- Kim EM Jeong HJ Park IK Asialoglycoprotein receptor targeted gene delivery using galactosylated polyethylenimine- graft-poly(ethylene glycol): in vitro and in vivo studies J Control Release 2005 108 557 567 16253376
- Wang YQ Su J Wu F Biscarbamate cross-linked polyethylenimine derivative with low molecular weight, low cytotoxicity and high efficiency for gene delivery Int J Nanomedicine 2012 7 693 704 22359448
- Oba M Miyata K Osada K Polyplex micelles prepared from u-cholesteryl PEG-polycation block copolymers for systemic gene delivery Biomaterials 2011 32 652 663 20932567
- Neu M Germershaus O Behe M Kissel T Bioreversibly cross-linked polyplexes of PEI and high molecular weight PEG show extended circulation times in vivo J Control Release 2007 124 69 80 17897749
- Jiang HL Kwon JT Kim EM Galactosylated poly(ethylene glycol)-chitosan-graft-polyethylenimine as a gene carrier for hepatocyte-targeting J Control Release 2008 131 150 157 18706946
- Neu M Fischer D Kissel T Recent advances in rational gene transfer vector design based on poly(ethylene imine) and its derivatives J Gene Med 2005 7 992 1009 15920783
- Harada A Togawa H Kataoka K Physicochemical properties and nuclease resistance of antisense-oligonucleotides entrapped in the core of polyion complex micelles composed of poly(ethylene glycol)-poly (L-lysine) block copolymers Eur J Pharm Sci 2001 13 35 42 11292566
- Jeong JH Kim SW Park TG Novel intracellular delivery system of antisense oligonucleotide by self assembled hybrid micelles composed of DNA/PEG conjugate and cationic fusogenic peptide Bioconjug Chem 2003 14 473 479 12643759
- Hashida M Takemura S Nishikawa M Takakura Y Targeted delivery of plasmid DNA complexed with galactosylated poly(L-lysine) J Control Release 1998 53 301 310 9741938
- Litzinger DC Buiting AM van Rooijen N Huang L Effect of liposome size on the circulation time and intraorgan distribution of amphipathic poly(ethylene glycol)-containing liposomes Biochim Biophys Acta 1994 1190 99 107 8110825
- Patiño T Nogués C Ibáñez E Barrios L Enhancing microparticle internalization by nonphagocytic cells through the use of noncovalently conjugated polyethyleneimine Int J Nanomedicine 2012 7 5671 5682 23152683
- Xu Z Chen L Gu W The performance of docetaxel-loaded solid lipid nanoparticles targeted to hepatocellular carcinoma Biomaterials 2009 30 226 232 18851881
- Suo A Qian J Yao Y Zhang W Galactosylated poly(ethylene glycol)-b-poly (l-lactide-co-β-malic acid) block copolymer micelles for targeted drug delivery: preparation and in vitro characterization Int J Nanomedicine 2010 5 1029 1038 21170351
- Lv H Zhang S Wang B Cui S Yan J Toxicity of cationic lipids and cationic polymers in gene delivery J Control Release 2006 114 100 109 16831482
- Kunath K von Harpe A Fischer D Low-molecular-weight polyethylenimine as a non-viral vector for DNA delivery: comparison of physicochemical properties, transfection efficiency and in vivo distribution with high-molecular-weight polyethylenimine J Control Release 2003 89 113 125 12695067
- Zhang X Pan SR Hu HM Poly(ethylene glycol)-block-polyethylenimine copolymers as carriers for gene delivery: effects of PEG molecular weight and PEGylation degree J Biomed Mater Res A 2008 84 795 804 17635020
- Kostarelos K Rational design and engineering of delivery systems for therapeutics: biomedical exercises in colloid and surface science Adv Colloid Interface Sci 2003 106 147 168 14672846
- Bieber T Elsässer HP Preparation of a low molecular weight polyethylenimine for efficient cell transfection Biotechniques 2001 30 74 77 80 81 11196323
- Gao Y Zhang Z Chen L Gu W Li Y Synthesis of 6-N, N, N- trimethyltriazole chitosan via “click chemistry” and evaluation for gene delivery Biomacromolecules 2009 10 2175 2182 19722556
- Gao Y Xu Z Chen S Gu W Chen L Li Y Arginine–chitosan/DNA self-assemble nanoparticles for gene delivery: in vitro characteristics and transfection efficiency Int J Pharm 2008 359 241 246 18479851