Abstract
NAD+ is a fundamental molecule in metabolism and redox signaling. In diabetes and its complications, the balance between NADH and NAD+ can be severely perturbed. On one hand, NADH is overproduced due to influx of hyperglycemia to the glycolytic and Krebs cycle pathways and activation of the polyol pathway. On the other hand, NAD+ can be diminished or depleted by overactivation of poly ADP ribose polymerase that uses NAD+ as its substrate. Moreover, sirtuins, another class of enzymes that also use NAD+ as their substrate for catalyzing protein deacetylation reactions, can also affect cellular content of NAD+. Impairment of NAD+ regeneration enzymes such as lactate dehydrogenase in erythrocytes and complex I in mitochondria can also contribute to NADH accumulation and NAD+ deficiency. The consequence of NADH/NAD+ redox imbalance is initially reductive stress that eventually leads to oxidative stress and oxidative damage to macromolecules, including DNA, lipids, and proteins. Accordingly, redox imbalance-triggered oxidative damage has been thought to be a major factor contributing to the development of diabetes and its complications. Future studies on restoring NADH/NAD+ redox balance could provide further insights into design of novel antidiabetic strategies.
Introduction
Chronic elevation of blood glucose, known as diabetic hyperglycemia, is a hallmark of diabetes mellitus.Citation1–Citation4 This persistent hyperglycemia can lead to long term damage to tissues such as the kidney, eyes, nerves, blood vessels, and heart. Citation3,Citation5,Citation6 For non-insulin-dependent tissues, a high level of blood glucose would mean a high level of glucose metabolism as glucose entry into the cells is not limited by insulin deficiency.Citation7,Citation8 Since one of the major purposes of glucose metabolism is to provide electrons that are stored mainly in NADH and FADH2 for ATP production via the processes of glycolysis and mitochondrial metabolic pathways, NADH would be in an oversupply state when glucose overload occurs. This excess NADH can break the redox balance between NADH and NAD+, and eventually can lead to oxidative stress and a variety of metabolic syndromes.Citation9–Citation13 Hence, it suffices to say that diabetes is a redox imbalance disease.Citation14,Citation15
In this review, we delineate the sources and the pathways that contribute to NADH/NAD+ redox imbalance, and the potential consequences of this redox imbalance in diabetes. Regarding pathways that contribute to NADH/NAD+ redox imbalance, we focus on both the conventional glucose metabolic pathways and polyol pathway that get activated by high level of blood glucose.Citation16–Citation18 We also discuss the pathways that utilize NAD+ as substrates such as sirtuins deacetylation pathwaysCitation19,Citation20 and poly ADP ribosylation pathway.Citation21,Citation22 Additionally, NADH/NAD+-recycling enzymes such as lactate dehydrogenase (LDH) and mitochondrial complex I (NADH-ubiquinone oxidoreductaseCitation23,Citation24) are also discussed. We believe that the consequences triggered by NADH/NAD+ redox imbalance are eventually reflected by oxidative stress and cell death that are known to be involved in the pathogenesis of diabetes and its complications.
NADH production by the conventional glucose metabolic pathways
The pair of NADH and NAD+ plays a crucial role in metabolism and redox signaling.Citation25–Citation30 The central pathways involved in complete glucose breakdown and electron storage in NADH are the glycolytic pathway and the Krebs cycle. As shown in , glyceraldehyde 3-phosphate dehydrogenase in the glycolytic pathway makes NADH from NAD+. This is followed by pyruvate dehydrogenase complex that also makes NADH from NAD+, whereby the actual enzyme catalyzing NADH formation is dihydrolipoamide dehydrogenase.Citation31,Citation32 After acetyl-CoA enters into the Krebs cycle, more molecules of NADH are produced, which can be ascribed to the action of isocitrate dehydrogenase, α-ketoglutarate dehydrogenase, and malate dehydrogenase, respectively. Fatty acid β-oxidation fueling the production of acetyl-CoA can also be a significant source of NADH.Citation33 Additionally, glutamate dehydrogenase, a central enzyme involved in α-ketoglutarate formation from glutamate,Citation34 can also make NADH from NAD+.Citation35,Citation36 Under hyperglycemic conditions, both the glycolytic pathway and the Krebs cycle can be intensively fluxed by glucose.Citation37 Therefore, NADH can be overproduced in diabetes via these pathways,Citation38 and excess NADH is known to cause reductive stress.Citation13,Citation39–Citation43
Figure 1 Metabolic pathways and enzymes involved in NADH production using NAD+ as their cofactor.
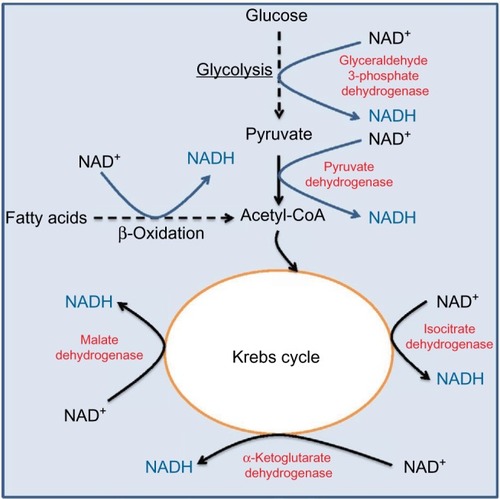
NADH production by polyol pathway
The polyol pathway, as shown in , involves two consecutive reactions that are catalyzed by aldose reductase and sorbitol dehydrogenase, respectively. This pathway is usually rather inactive under euglycemic conditionCitation16 but can become a highly active glucose disposal pathway under diabetic hyperglycemic condition.Citation44,Citation45 The major feature of this pathway is the production of NADH, sorbitol, and fructose.Citation16,Citation46–Citation48 Each of these intermediates or products plays a role in the pathogenesis of diabetes and its complications.Citation16,Citation46–Citation49 For example, sorbitol can accumulate in retinal and renal tissues and causes osmotic stress and cell death,Citation50,Citation51 and fructose can cause nonenzymatic protein glycation or nitrationCitation52,Citation53 and contributes to pathogenesis of nonalcoholic fatty liver disease.Citation54 More importantly, a massive NADH production by this pathway is known to perturb redox imbalance between NADH and NAD+, and consumption of NADPH can impair the function of glutathione reductase, leading to accumulation of oxidized form of glutathione and further accentuation of redox imbalance.Citation13,Citation55 As such, inhibition or deletion of aldose reductase, a rate-limiting enzyme in the polyol pathway, has been demonstrated to be antidiabetic.Citation56–Citation60
Figure 2 Polyol pathway.
Abbreviations: GSSG, oxidized glutathione; GSH, reduced glutathione.
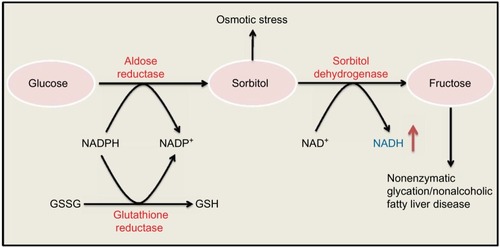
NAD+-degradation pathways
NAD+ is not only an electron acceptor but can also serve as a substrate and be degraded during enzyme-catalyzed reactions. Two major enzymatic pathways that use NAD+ as their substrate are sirtuins and poly ADP ribose polymerases (PARPs).Citation27,Citation61 As shown in , sirtuins use NAD+ for their deacetylation reactions, whereby NAD+ is degraded and nicotinamide and 2′-O-acetyl-ADP ribose are formed. Sirtuins are inducible enzymes.Citation62,Citation63 Therefore, if NAD+ level is low, sirtuin protein content would be low.Citation63,Citation64 As acetylated proteins usually exhibit impaired functions,Citation65,Citation66 deacetylation by sirtuins usually improve the function of the target proteins.Citation67 Therefore, sirtuins can be activated by starvation or caloric restriction to safeguard cell survival.Citation68,Citation69 On the other hand, overnutrition such as in diabetes that usually produces excess NADH with diminished NAD+ content can often lead to attenuation of sirtuin protein content.Citation20,Citation70 Therefore, enhancing sirtuin expression in diabetic tissues has been suggested as a therapeutic approach for treating diabetes and its complications.Citation71,Citation72 It should be noted that among the seven members of the sirtuin family,Citation19,Citation73 sirtuin 4 does not possess deacetylation activity but rather exhibits mono- or poly ADP ribosyltransferase activity.Citation74
Figure 3 Two enzyme systems that are involved in NAD+ degradation.
Abbreviation: 2′-O-acetyl-ADPR, 2′-O-acetyl-ADP ribose.
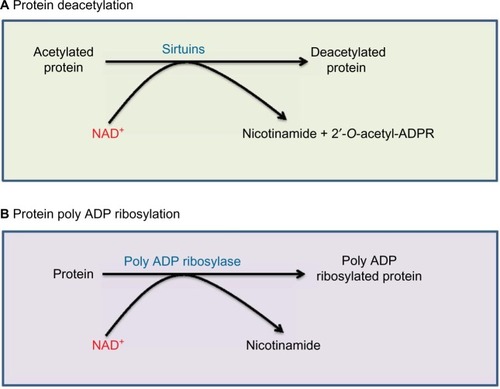
While numerous studies demonstrate that elevating sirtuin protein content, such as that of sirtuin 3, ameliorates diabetes and its complications,Citation67,Citation75–Citation77 a question arising is that whether it is possible that elevated levels of sirtuins consume more NAD+ and make the redox imbalance situation worse. This seemingly is not the case. It is probable that elevated levels of sirtuins alter the profiles of a given acetylated/deacetylated proteome, rendering metabolic pathways more efficient, which leads to more NADH utilization and thus more NAD+ regeneration.Citation74 It has been reported that deacetylation by sirtuin protein can enhance NADPH production, which may be involved in restoring cellular redox balance.Citation78 Nonetheless, whether elevation of sirtuin levels in diabetes could restore or improve NADH/NAD+ redox balance needs to be further thoroughly investigated.
Another enzyme system that consumes and degrades NAD+ is PARPs, especially PARP-1Citation79 that can be activated by DNA damage.Citation22,Citation80 As shown in , the products of PARP-catalyzed reaction are poly ADP ribosylated proteins and nicotinamide derived from NAD+. The problem caused by activation of PARP in diabetes is that the enzyme is often overactivated,Citation81–Citation83 resulting in potential depletion of NAD+, which would further perturb NADH/NAD+ redox balance, leading to cell death.Citation21,Citation79,Citation84,Citation85 PARP has been touted as a promising target for antidiabetic therapy. Indeed, knocking out or knocking down PARP expression can prevent animals from developing diabetes.Citation86–Citation88 Drugs that inhibit PARP activity have also been developed and tested for antidiabetic therapy.Citation89–Citation93 For example, 1,5-isoquinolinediol as a PARP inhibitor has been shown to improve corneal epithelial innervation in diabetic rats,Citation94 and PARP inhibition could improve erectile function in diabetic rodents.Citation95
Regeneration of NAD+ from NADH
For metabolism to continue, NAD+ has to be regenerated from NADH. There are two major pathways that can achieve this task, namely, LDHCitation8 and mitochondrial complex I that is the first electron entry point in the electron transport chain.Citation96–Citation98 In anaerobic metabolism such as in erythrocytes where no mitochondria exist, LDH is responsible for NAD+ regenerationCitation8,Citation99 (). Under aerobic condition, however, mitochondrial complex I is responsible for NAD+ regenerationCitation8,Citation98 (). Hence, it is imaginable that NADH oversupply could overwhelm LDHCitation100 or complex I.Citation101 Indeed, it has been shown that diabetic hyperglycemia increases the enzyme activity of LDH in red blood cells and in small platelets to handle NADH over-influx.Citation102,Citation103 On the other hand, changes in complex I function in diabetes and its complications remain very sketchy. Nonetheless, it has been reported that complex I activity is decreased in diabetic skeletal musclesCitation104 but increased in diabetic kidneys.Citation105 Therefore, it seems that changes in complex I activity are tissue dependent in diabetic subjects. It would be interesting to survey complex I activity from tissue to tissue in diabetic rodents or possibly humans.
Detrimental effects of redox imbalance in diabetes
When excess NADH accumulates, the enzymes that produce NADH from NAD+ will be inhibited. For example, both glyceraldehyde 3-phosphate dehydrogenase and dihydrolipoamide dehydrogenase in the pyruvate dehydrogenase complex can be inhibited by NADH,Citation106,Citation107 leading to potential reactive oxygen species (ROS) production.Citation82,Citation108,Citation109 Moreover, mitochondrial electron transport chain can be overloaded by this electron donor.Citation110 The direct pressure of this NADH overload would be on complex I, which is a major site for generation of ROS.Citation111–Citation116 The feature of this 45-subunit complexCitation117 in ROS production is that the more NADH it oxidizes, the more ROS it will produce.Citation112,Citation114,Citation118–Citation120 Therefore, oxidants will overwhelm cellular antioxidant systems, leading to mitochondrial membrane permeability transition pore openingCitation121,Citation122 and mitochondrial dysfunction that are concurrent with extensive oxidative damage to proteins, DNA, and lipidsCitation123–Citation127 (). These oxidized macromolecules can accumulate over time, manifest diabetic glucotoxicity,Citation128–Citation131 and eventually lead to insulin resistance,Citation132–Citation135 β-cell insulin deficiency,Citation136–Citation138 and global cell death and tissue dysfunction.Citation82,Citation139–Citation144 Indeed, oxidative damage and oxidative stress have been demonstrated to be involved in the pathogenesis of diabetes and its complications.Citation145–Citation148 Relevantly, inhibition of complex I has been shown to activate 5′-AMP-activated protein kinase and improves glucose metabolism in diabetes,Citation149–Citation152 supporting the observation that complex I ROS production plays a role in diabetes.Citation153–Citation157 Therefore, restoring redox balance or attenuating oxidative stress should be a promising approach to treating these chronic age-related diseases. Additionally, roles of antioxidants in antidiabetic therapy should also be highly appreciated as antioxidants usually work by ultimately improving redox balance.Citation158–Citation163
Figure 5 Consequences of NADH/NAD+ redox imbalance.
Abbreviations: ROS, reactive oxygen species; PARP, poly ADP ribose polymerase.
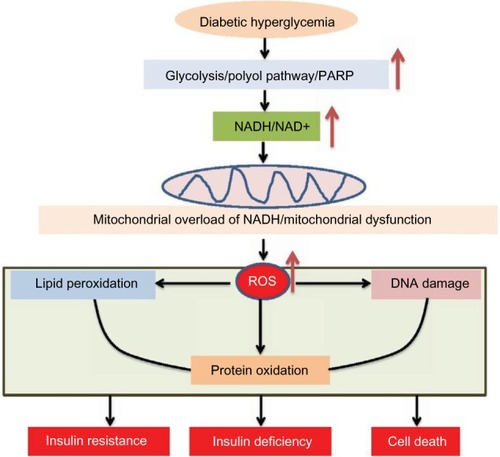
Summary and future perspectives
As has been discussed earlier, there is a severe redox imbalance problem occurring in diabetes and its complications. For cells whose glucose uptake is not dependent on insulin, glucose oversupply can lead to NADH overproduction by both the conventional glucose combustion pathways () and the polyol pathway (). On the other hand, overactivation of PARP can diminish or deplete the cellular NAD+ pool (), thereby potentially downregulating sirtuins expression and making the redox imbalance situation worse. While drugs inhibiting aldose reductase in the polyol pathwayCitation164,Citation165 or PARPCitation89,Citation90 will continue to remain as active areas of investigation in the future, NAD+ regeneration enzymes such as complex I should also be studiedCitation38 to provide insights into how excess NADH can be oxidized under glucose overload conditions. Additionally, administration of NAD+ precursors or analogsCitation166,Citation167 can also serve as an approach to treating diabetes and its complications. The ultimate goals of all these prospective studies are to restore NADH/NAD+ redox balance in diabetes and its complications for therapeutic purposes.
Acknowledgments
LJ Yan was supported in part by the National Institute of Neurological Disorders and Stroke (grant: R01NS079792).
Disclosure
The authors report no conflicts of interest in this work.
References
- DeFronzo RA Pathogenesis of type 2 diabetes mellitus Med Clin North Am 2004 88 4 787 835 ix 15308380
- Abdul-Ghani MA DeFronzo RA Oxidative stress in type 2 diabetes Miwa S Beckman KB Muller FL Oxidative Stress in Aging Totowa, New Jersey Humana Press 2008 191 212
- Barnett AH Type 2 Diabetes 2nd ed Oxford, UK Oxford University Press 2012
- Tuch B Dunlop M Proietto J Diabetes Research: A Guide for Postgraduates Amsterdam, the Netherlands Harwood Academic Publishers 2000
- Dedoussis GV Kaliora AC Panagiotakos DB Genes, diet and type 2 diabetes mellitus: a review Rev Diabet Stud 2007 4 1 13 24 17565412
- Del Prato S Role of glucotoxicity and lipotoxicity in the pathophysiology of Type 2 diabetes mellitus and emerging treatment strategies Diabet Med 2009 26 12 1185 1192 20002468
- Gallwitz B Kazda C Kraus P Nicolay C Schernthaner G Contribution of insulin deficiency and insulin resistance to the development of type 2 diabetes: nature of early stage diabetes Acta Diabetol 2013 50 1 39 45 21861172
- Lieberman M Marks AD Marks’ Basic Medical Biochemistry: A Clinical Approach 4th ed Philadelphia, PA Wolters Kluwer/Lippincott Williams & Wilkins 2013
- Humphries KM Szweda PA Szweda LI Aging: a shift from redox regulation to oxidative damage Free Radic Res 2006 40 12 1239 1243 17090412
- Ido Y Williamson JR Hyperglycemic cytosolic reductive stress ‘pseudohypoxia’: implications for diabetic retinopathy Invest Ophthalmol Vis Sci 1997 38 8 1467 1470 9224273
- Teodoro JS Rolo AP Palmeira CM The NAD ratio redox paradox: why does too much reductive power cause oxidative stress? Toxicol Mech Methods 2013 23 5 297 302 23256455
- Lipinski B Evidence in support of a concept of reductive stress Br J Nutr 2002 87 1 93 94 discussion 94 11895317
- Yan LJ Pathogenesis of chronic hyperglycemia: from reductive stress to oxidative stress J Diabetes Res 2014 2014 137919 25019091
- Hayden MR Tyagi SC Islet redox stress: the manifold toxicities of insulin resistance, metabolic syndrome and amylin derived islet amyloid in type 2 diabetes mellitus JOP 2002 3 4 86 108 12110767
- Hayden MR Sowers JR Redox imbalance in diabetes Antioxid Redox Signal 2007 9 7 865 867 17477794
- Dunlop M Aldose reductase and the role of the polyol pathway in diabetic nephropathy Kidney Int Suppl 2000 77 S3 S12 10997684
- Hotta N New concepts and insights on pathogenesis and treatment of diabetic complications: polyol pathway and its inhibition Nagoya J Med Sci 1997 60 3–4 89 100 9481088
- Lee AY Chung SS Contributions of polyol pathway to oxidative stress in diabetic cataract FASEB J 1999 13 1 23 30 9872926
- Morris BJ Seven sirtuins for seven deadly diseases of aging Free Radic Biol Med 2013 56 133 171 23104101
- Turkmen K Karagoz A Kucuk A Sirtuins as novel players in the pathogenesis of diabetes mellitus World J Diabetes 2014 5 6 894 900 25512793
- Pacher P Szabo C Role of poly(ADP-ribose) polymerase-1 activation in the pathogenesis of diabetic complications: endothelial dysfunction, as a common underlying theme Antioxid Redox Signal 2005 7 11–12 1568 1580 16356120
- Hassa PO Haenni SS Elser M Hottiger MO Nuclear ADP-ribosylation reactions in mammalian cells: where are we today and where are we going? Microbiol Mol Biol Rev 2006 70 3 789 829 16959969
- Schapira AH Human complex I defects in neurodegenerative diseases Biochim Biophys Acta 1998 1364 2 261 270 9593927
- Schapira AH Cooper JM Dexter D Clark JB Jenner P Marsden CD Mitochondrial complex I deficiency in Parkinson’s disease J Neurochem 1990 54 3 823 827 2154550
- Lin SJ Guarente L Nicotinamide adenine dinucleotide, a metabolic regulator of transcription, longevity and disease Curr Opin Cell Biol 2003 15 2 241 246 12648681
- Oka S Hsu CP Sadoshima J Regulation of cell survival and death by pyridine nucleotides Circ Res 2012 111 5 611 627 22904041
- Nikiforov A Kulikova V Ziegler M The human NAD metabolome: functions, metabolism and compartmentalization Crit Rev Biochem Mol Biol 2015 50 4 284 297 25837229
- Chiarugi A Dolle C Felici R Ziegler M The NAD metabolome–a key determinant of cancer cell biology Nat Rev Cancer 2012 12 11 741 752 23018234
- Mouchiroud L Houtkooper RH Auwerx J NAD(+) metabolism: a therapeutic target for age-related metabolic disease Crit Rev Biochem Mol Biol 2013 48 4 397 408 23742622
- Houtkooper RH Canto C Wanders RJ Auwerx J The secret life of NAD+: an old metabolite controlling new metabolic signaling pathways Endocr Rev 2010 31 2 194 223 20007326
- Yan LJ Yang SH Shu H Prokai L Forster MJ Histochemical staining and quantification of dihydrolipoamide dehydrogenase diaphorase activity using blue native PAGE Electrophoresis 2007 28 7 1036 1045 17315258
- Yan LJ Thangthaeng N Forster MJ Changes in dihydrolipoamide dehydrogenase expression and activity during postnatal development and aging in the rat brain Mech Ageing Dev 2008 129 282 290 18316113
- Ido Y Pyridine nucleotide redox abnormalities in diabetes Antioxid Redox Signal 2007 9 7 931 942 17508915
- Li M Li C Allen A Stanley CA Smith TJ Glutamate dehydrogenase: structure, allosteric regulation, and role in insulin homeostasis Neurochem Res 2014 39 3 433 445 24122080
- Gohring I Mulder H Glutamate dehydrogenase, insulin secretion, and type 2 diabetes: a new means to protect the pancreatic beta-cell? J Endocrinol 2012 212 3 239 242 22232141
- Otter S Lammert E Exciting times for pancreatic islets: glutamate signaling in endocrine cells Trends Endocrinol Metab 2016 27 3 177 188 26740469
- Jankovic A Korac A Buzadzic B Redox implications in adipose tissue (dys)function-a new look at old acquaintances Redox Biol 2015 6 19 32 26177468
- Luo X Li R Yan LJ Roles of pyruvate, NADH, and mitochondrial complex I in redox balance and imbalance in β cell function and dysfunction J Diabetes Res 2015 2015 512618 26568959
- Dawson TL Gores GJ Nieminen AL Herman B Lemasters JJ Mitochondria as a source of reactive oxygen species during reductive stress in rat hepatocytes Am J Physiol 1993 264 4 Pt 1 C961 C967 8386454
- Comporti M Signorini C Leoncini S Ethanol-induced oxidative stress: basic knowledge Genes Nutr 2010 5 2 101 109 20606811
- Jaeschke H Kleinwaechter C Wendel A NADH-dependent reductive stress and ferritin-bound iron in allyl alcohol-induced lipid peroxidation in vivo: the protective effect of vitamin E Chem Biol Interact 1992 81 1–2 57 68 1730148
- Yan LJ Levine RL Sohal RS Oxidative damage during aging targets mitochondrial aconitase Proc Natl Acad Sci U S A 1997 94 11168 11172 9326580
- Valadi H Valadi A Ansell R NADH-reductive stress in Saccharomyces cerevisiae induces the expression of the minor isoform of glyceraldehyde-3-phosphate dehydrogenase (TDH1) Curr Genet 2004 45 2 90 95 14652693
- Fantus IG The pathogenesis of the chronic complications of the diabetes mellitus Endocrinol Rounds 2002 2 4 1 8
- Boesten DM von Ungern-Sternberg SN den Hartog GJ Bast A Protective pleiotropic effect of flavonoids on NAD(+) levels in endothelial cells exposed to high glucose Oxid Med Cell Longev 2015 2015 894597 26180598
- Chung SS Chung SK Aldose reductase in diabetic microvascular complications Curr Drug Targets 2005 6 4 475 486 16026266
- Hodgkinson AD Sondergaard KL Yang B Cross DF Millward BA Demaine AG Aldose reductase expression is induced by hyperglycemia in diabetic nephropathy Kidney Int 2001 60 1 211 218 11422753
- Iwata K Nishinaka T Matsuno K The activity of aldose reductase is elevated in diabetic mouse heart J Pharmacol Sci 2007 103 4 408 416 17384488
- Kador PF Kinoshita JH Role of aldose reductase in the development of diabetes-associated complications Am J Med 1985 79 5A 8 12 3934965
- Gabbay KH The sorbitol pathway and the complications of diabetes N Engl J Med 1973 288 16 831 836 4266466
- Bagnasco SM Murphy HR Bedford JJ Burg MB Osmoregulation by slow changes in aldose reductase and rapid changes in sorbitol flux Am J Physiol 1988 254 6 Pt 1 C788 C792 3132044
- Takagi Y Kashiwagi A Tanaka Y Asahina T Kikkawa R Shigeta Y Significance of fructose-induced protein oxidation and formation of advanced glycation end product J Diabetes Complications 1995 9 2 87 91 7599353
- Prince PD Lanzi CR Toblli JE Dietary (−)-epicatechin mitigates oxidative stress, NO metabolism alterations, and inflammation in renal cortex from fructose-fed rats Free Radic Biol Med 2016 90 35 46 26569027
- Lirio LM Forechi L Zanardo TC Chronic fructose intake accelerates non-alcoholic fatty liver disease in the presence of essential hypertension J Diabetes Complications 2016 30 1 85 92 26597602
- Ying W NAD+/NADH and NADP+/NADPH in cellular functions and cell death: regulation and biological consequences Antioxid Redox Signal 2008 10 2 179 206 18020963
- Tang WH Martin KA Hwa J Aldose reductase, oxidative stress, and diabetic mellitus Front Pharmacol 2012 3 87 22582044
- Ohmura C Watada H Azuma K Aldose reductase inhibitor, epalrestat, reduces lipid hydroperoxides in type 2 diabetes Endocr J 2009 56 1 149 156 18997444
- Reddy AB Ramana KV Aldose reductase inhibition: emerging drug target for the treatment of cardiovascular complications Recent Pat Cardiovasc Drug Discov 2010 5 1 25 32 19886861
- Suzen S Buyukbingol E Recent studies of aldose reductase enzyme inhibition for diabetic complications Curr Med Chem 2003 10 15 1329 1352 12871133
- Tang J Du Y Petrash JM Sheibani N Kern TS Deletion of aldose reductase from mice inhibits diabetes-induced retinal capillary degeneration and superoxide generation PLoS One 2013 8 4 e62081 23614016
- Massudi H Grant R Guillemin GJ Braidy N NAD+ metabolism and oxidative stress: the golden nucleotide on a crown of thorns Redox Rep 2012 17 1 28 46 22340513
- Sauve AA Sirtuin chemical mechanisms Biochim Biophys Acta 2010 1804 8 1591 1603 20132909
- Vedantham S Thiagarajan D Ananthakrishnan R Aldose reductase drives hyperacetylation of Egr-1 in hyperglycemia and consequent upregulation of proinflammatory and prothrombotic signals Diabetes 2014 63 2 761 774 24186862
- Cerutti R Pirinen E Lamperti C NAD(+)-dependent activation of Sirt1 corrects the phenotype in a mouse model of mitochondrial disease Cell Metab 2014 19 6 1042 1049 24814483
- Yang T Sauve AA NAD metabolism and sirtuins: metabolic regulation of protein deacetylation in stress and toxicity AAPS J 2006 8 4 E632 E643 17233528
- Kosanam H Thai K Zhang Y Diabetes induces lysine acetylation of intermediary metabolism enzymes in the kidney Diabetes 2014 63 7 2432 2439 24677711
- Hirschey MD Shimazu T Jing E SIRT3 deficiency and mitochondrial protein hyperacetylation accelerate the development of the metabolic syndrome Mol Cell 2011 44 2 177 190 21856199
- Tucci P Caloric restriction: is mammalian life extension linked to p53? Aging 2012 4 8 525 534 22983298
- Wang Y Molecular links between caloric restriction and Sir2/SIRT1 activation Diabetes Metab J 2014 38 5 321 329 25349818
- de Kreutzenberg SV Ceolotto G Papparella I Downregulation of the longevity-associated protein sirtuin 1 in insulin resistance and metabolic syndrome: potential biochemical mechanisms Diabetes 2010 59 4 1006 1015 20068143
- Kitada M Kume S Kanasaki K Takeda-Watanabe A Koya D Sirtuins as possible drug targets in type 2 diabetes Curr Drug Targets 2013 14 6 622 636 23445543
- Huynh FK Hershberger KA Hirschey MD Targeting sirtuins for the treatment of diabetes Diabetes Manag (Lond) 2013 3 3 245 257 25067957
- Schlicker C Gertz M Papatheodorou P Kachholz B Becker CF Steegborn C Substrates and regulation mechanisms for the human mitochondrial sirtuins Sirt3 and Sirt5 J Mol Biol 2008 382 3 790 801 18680753
- Pereira CV Lebiedzinska M Wieckowski MR Oliveira PJ Regulation and protection of mitochondrial physiology by sirtuins Mitochondrion 2012 12 1 66 76 21787885
- Jing E Emanuelli B Hirschey MD Sirtuin-3 (Sirt3) regulates skeletal muscle metabolism and insulin signaling via altered mitochondrial oxidation and reactive oxygen species production Proc Natl Acad Sci U S A 2011 108 35 14608 14613 21873205
- Hou X Zeng H He X Chen JX Sirt3 is essential for apelin-induced angiogenesis in post-myocardial infarction of diabetes J Cell Mol Med 2015 19 1 53 61 25311234
- Fritz KS Galligan JJ Hirschey MD Verdin E Petersen DR Mitochondrial acetylome analysis in a mouse model of alcohol-induced liver injury utilizing SIRT3 knockout mice J Proteome Res 2012 11 3 1633 1643 22309199
- Circu ML Aw TY Reactive oxygen species, cellular redox systems, and apoptosis Free Radic Biol Med 2010 48 6 749 762 20045723
- Puthanveetil P Zhang D Wang Y Diabetes triggers a PARP1 mediated death pathway in the heart through participation of FoxO1 J Mol Cell Cardiol 2012 53 5 677 686 22940604
- Mueller-Dieckmann C Kernstock S Lisurek M The structure of human ADP-ribosylhydrolase 3 (ARH3) provides insights into the reversibility of protein ADP-ribosylation Proc Natl Acad Sci U S A 2006 103 41 15026 15031 17015823
- Horvath EM Magenheim R Kugler E Nitrative stress and poly(ADP-ribose) polymerase activation in healthy and gestational diabetic pregnancies Diabetologia 2009 52 9 1935 1943 19597800
- Du X Matsumura T Edelstein D Inhibition of GAPDH activity by poly(ADP-ribose) polymerase activates three major pathways of hyperglycemic damage in endothelial cells J Clin Invest 2003 112 7 1049 1057 14523042
- Dolle C Rack JG Ziegler M NAD and ADP-ribose metabolism in mitochondria FEBS J 2013 280 15 3530 3541 23617329
- Obrosova IG Drel VR Pacher P Oxidative-nitrosative stress and poly(ADP-ribose) polymerase (PARP) activation in experimental diabetic neuropathy: the relation is revisited Diabetes 2005 54 12 3435 3441 16306359
- Chiu J Xu BY Chen S Feng B Chakrabarti S Oxidative stress-induced, poly(ADP-ribose) polymerase-dependent upregulation of ET-1 expression in chronic diabetic complications Can J Physiol Pharmacol 2008 86 6 365 372 18516100
- Masutani M Suzuki H Kamada N Poly(ADP-ribose) polymerase gene disruption conferred mice resistant to streptozotocin-induced diabetes Proc Natl Acad Sci U S A 1999 96 5 2301 2304 10051636
- Pieper AA Brat DJ Krug DK Poly(ADP-ribose) polymerase-deficient mice are protected from streptozotocin-induced diabetes Proc Natl Acad Sci U S A 1999 96 6 3059 3064 10077636
- Virag L Szabo C The therapeutic potential of poly(ADP-ribose) polymerase inhibitors Pharmacol Rev 2002 54 3 375 429 12223530
- Long CA Boulom V Albadawi H Poly-ADP-ribose-polymerase inhibition ameliorates hind limb ischemia reperfusion injury in a murine model of type 2 diabetes Ann Surg 2013 258 6 1087 1095 23549425
- Sarras MPJr Mason S McAllister G Intine RV Inhibition of poly-ADP ribose polymerase enzyme activity prevents hyperglycemia-induced impairment of angiogenesis during wound healing Wound Repair Regen 2014 22 5 666 670 25066843
- Szkudelski T Streptozotocin-nicotinamide-induced diabetes in the rat. Characteristics of the experimental model Exp Biol Med (Maywood) 2012 237 5 481 490 22619373
- Fukaya M Tamura Y Chiba Y Protective effects of a nicotinamide derivative, isonicotinamide, against streptozotocin-induced beta-cell damage and diabetes in mice Biochem Biophys Res Commun 2013 442 1–2 92 98 24246675
- Obrosova IG Minchenko AG Frank RN Poly(ADP-ribose) polymerase inhibitors counteract diabetes- and hypoxia-induced retinal vascular endothelial growth factor overexpression Int J Mol Med 2004 14 1 55 64 15202016
- Byun YS Kang B Yoo YS Joo CK Poly(ADP-ribose) polymerase inhibition improves corneal epithelial innervation and wound healing in diabetic rats Invest Ophthalmol Vis Sci 2015 56 3 1948 1955 25711635
- Li WJ Zhou J Li B Wang H Peng YB Wang Z PARP inhibition restores erectile function by suppressing corporal smooth muscle apoptosis in diabetic rats J Sex Med 2011 8 4 1072 1082 21235725
- Hirst J Mitochondrial complex I Annu Rev Biochem 2013 82 551 575 23527692
- Andrews B Carroll J Ding S Fearnley IM Walker JE Assembly factors for the membrane arm of human complex I Proc Natl Acad Sci U S A 2013 110 47 18934 18939 24191001
- Papa S Sardanelli AM Scacco S The NADH: ubiquinone oxidoreductase (complex I) of the mammalian respiratory chain and the cAMP cascade J Bioenerg Biomembr 2002 34 1 1 10 11860175
- Rapoport S The regulation of glycolysis in mammalian erythrocytes Essays Biochem 1968 4 69 103 4308730
- Leong HS Brownsey RW Kulpa JE Allard MF Glycolysis and pyruvate oxidation in cardiac hypertrophy–why so unbalanced? Comp Biochem Physiol A Mol Integr Physiol 2003 135 4 499 513 12890541
- He Q Wang M Petucci C Gardell SJ Han X Rotenone induces reductive stress and triacylglycerol deposition in C2C12 cells Int J Biochem Cell Biol 2013 45 12 2749 2755 24104397
- Lippi G Mercadanti M Aloe R Targher G Erythrocyte mechanical fragility is increased in patients with type 2 diabetes Eur J Intern Med 2012 23 2 150 153 22284245
- Zappacosta B De Sole P Rossi C Marra G Ghirlanda G Giardina B Lactate dehydrogenase activity of platelets in diabetes mellitus Eur J Clin Chem Clin Biochem 1995 33 8 487 489 8547431
- Antoun G McMurray F Thrush AB Impaired mitochondrial oxidative phosphorylation and supercomplex assembly in rectus abdominis muscle of diabetic obese individuals Diabetologia 2015 58 12 2861 2866 26404066
- Wu J Luo X Yan LJ Two dimensional blue native/SDS-PAGE to identify mitochondrial complex I subunits modified by 4-hydroxynonenal (HNE) Front Physiol 2015 6 98 25859224
- Wilkinson KD Williams CHJr NADH inhibition and NAD activation of Escherichia coli lipoamide dehydrogenase catalyzing the NADH-lipoamide reaction J Biol Chem 1981 256 5 2307 2314 7007381
- Ussher JR Jaswal JS Lopaschuk GD Pyridine nucleotide regulation of cardiac intermediary metabolism Circ Res 2012 111 5 628 641 22904042
- Yan LJ Thangthaeng N Sumien N Forster MJ Serum dihydrolipoamide dehydrogenase is a labile enzyme J Biochem Pharmacol Res 2013 1 1 30 42 23646291
- Quinlan CL Goncalves RL Hey-Mogensen M Yadava N Bunik VI Brand MD The 2-oxoacid dehydrogenase complexes in mitochondria can produce superoxide/hydrogen peroxide at much higher rates than complex I J Biol Chem 2014 289 12 8312 8325 24515115
- Galloway CA Yoon Y Perspectives on: SGP symposium on mitochondrial physiology and medicine: what comes first, misshape or dysfunction? The view from metabolic excess J Gen Physiol 2012 139 6 455 463 22641640
- Bridges HR Jones AJ Pollak MN Hirst J Effects of metformin and other biguanides on oxidative phosphorylation in mitochondria Biochem J 2014 462 3 475 487 25017630
- Hirst J King MS Pryde KR The production of reactive oxygen species by complex I Biochem Soc Trans 2008 36 Pt 5 976 980 18793173
- Murphy MP How mitochondria produce reactive oxygen species Biochem J 2009 417 1 1 13 19061483
- Cooper JM Mann VM Krige D Schapira AH Human mitochondrial complex I dysfunction Biochim Biophys Acta 1992 1101 2 198 203 1633185
- Santidrian AF Matsuno-Yagi A Ritland M Mitochondrial complex I activity and NAD+/NADH balance regulate breast cancer progression J Clin Invest 2013 123 3 1068 1081 23426180
- Pryde KR Hirst J Superoxide is produced by the reduced flavin in mitochondrial complex I: a single, unified mechanism that applies during both forward and reverse electron transfer J Biol Chem 2011 286 20 18056 18065 21393237
- Carroll J Fearnley IM Skehel JM Shannon RJ Hirst J Walker JE Bovine complex I is a complex of 45 different subunits J Biol Chem 2006 281 43 32724 32727 16950771
- Treberg JR Quinlan CL Brand MD Evidence for two sites of super-oxide production by mitochondrial NADH-ubiquinone oxidoreductase (complex I) J Biol Chem 2011 286 31 27103 27110 21659507
- Esposti MD Ngo A Myers MA Inhibition of mitochondrial complex I may account for IDDM induced by intoxication with the rodenticide Vacor Diabetes 1996 45 11 1531 1534 8866557
- Fassone E Rahman S Complex I deficiency: clinical features, biochemistry and molecular genetics J Med Genet 2012 49 9 578 590 22972949
- Yan LJ Rajasekaran NS Sathyanarayanan S Benjamin IJ Mouse HSF1 disruption perturbs redox state and increases mitochondrial oxidative stress in kidney Antioxid Redox Signal 2005 7 3–4 465 471 15706094
- Yan LJ Christians ES Liu L Xiao X Sohal RS Benjamin IJ Mouse heat shock transcription factor 1 deficiency alters cardiac redox homeostasis and increases mitochondrial oxidative damage EMBO J 2002 21 19 5164 5172 12356732
- Ames BN Shigenaga MK Oxidants are a major contributor to aging Ann N Y Acad Sci 1992 663 85 96 1482105
- Helbock HJ Beckman KB Shigenaga MK DNA oxidation matters: the HPLC-electrochemical detection assay of 8-oxo-deoxyguanosine and 8-oxo-guanine Proc Natl Acad Sci U S A 1998 95 1 288 293 9419368
- Anderson EJ Katunga LA Willis MS Mitochondria as a source and target of lipid peroxidation products in healthy and diseased heart Clin Exp Pharmacol Physiol 2012 39 2 179 193 22066679
- Yan LJ Lodge JK Traber MG Packer L Apolipoprotein B carbonyl formation is enhanced by lipid peroxidation during copper-mediated oxidation of human low-density lipoproteins Arch Biochem Biophys 1997 339 1 165 171 9056246
- Berlett BS Stadtman ER Protein oxidation in aging, disease, and oxidative stress J Biol Chem 1997 272 33 20313 20316 9252331
- Luo X Wu J Jing S Yan LJ Hyperglycemic stress and carbon stress in diabetic glucotoxicity Aging Dis 2016 7 1 90 110 26816666
- Kaiser N Leibowitz G Nesher R Glucotoxicity and beta-cell failure in type 2 diabetes mellitus J Pediatr Endocrinol Metab 2003 16 1 5 22 12585335
- Brunner Y Schvartz D Priego-Capote F Coute Y Sanchez JC Glucotoxicity and pancreatic proteomics J Proteomics 2009 71 6 576 591 19027091
- Wu J Yan LJ Streptozotocin-induced type 1 diabetes in rodents as a model for studying mitochondrial mechanisms of diabetic beta cell glucotoxicity Diabetes Metab Syndr Obes 2015 8 181 188 25897251
- Kim JA Wei Y Sowers JR Role of mitochondrial dysfunction in insulin resistance Circ Res 2008 102 4 401 414 18309108
- Kaneki M Shimizu N Yamada D Chang K Nitrosative stress and pathogenesis of insulin resistance Antioxid Redox Signal 2007 9 3 319 329 17184170
- Henriksen EJ Diamond-Stanic MK Marchionne EM Oxidative stress and the etiology of insulin resistance and type 2 diabetes Free Radic Biol Med 2011 51 5 993 999 21163347
- Abel ED Free fatty acid oxidation in insulin resistance and obesity Heart Metab 2010 48 5 10 23646039
- Guo S Dai C Guo M Inactivation of specific beta cell transcription factors in type 2 diabetes J Clin Invest 2013 123 8 3305 3316 23863625
- Halban PA Polonsky KS Bowden DW Beta-cell failure in type 2 diabetes: postulated mechanisms and prospects for prevention and treatment Diabetes Care 2014 37 6 1751 1758 24812433
- Prentki M Nolan CJ Islet beta cell failure in type 2 diabetes J Clin Invest 2006 116 7 1802 1812 16823478
- Seo K Ki SH Shin SM Methylglyoxal induces mitochondrial dysfunction and cell death in liver Toxicol Res 2014 30 3 193 198 25343013
- Berg D Youdim MB Riederer P Redox imbalance Cell Tissue Res 2004 318 1 201 213 15365815
- Stadtman ER Protein oxidation and aging Science 1992 257 5074 1220 1224 1355616
- Stadtman ER Protein oxidation in aging and age-related diseases Ann N Y Acad Sci 2001 928 22 38 11795513
- Stadtman ER Protein oxidation and aging Free Radic Res 2006 40 12 1250 1258 17090414
- Stadler K Oxidative stress in diabetes Adv Exp Med Biol 2012 771 272 287 23393685
- Weaver JR Grzesik W Taylor-Fishwick DA Inhibition of NADPH oxidase-1 preserves beta cell function Diabetologia 2015 58 1 113 121 25277953
- Robertson RP Tanaka Y Takahashi H Tran PO Harmon JS Prevention of oxidative stress by adenoviral overexpression of glutathione-related enzymes in pancreatic islets Ann N Y Acad Sci 2005 1043 513 520 16037273
- Robertson RP Chronic oxidative stress as a central mechanism for glucose toxicity in pancreatic islet beta cells in diabetes J Biol Chem 2004 279 41 42351 42354 15258147
- Karunakaran U Park KG A systematic review of oxidative stress and safety of antioxidants in diabetes: focus on islets and their defense Diabetes Metab J 2013 37 2 106 112 23641350
- Srivastava RA Pinkosky SL Filippov S Hanselman JC Cramer CT Newton RS AMP-activated protein kinase: an emerging drug target to regulate imbalances in lipid and carbohydrate metabolism to treat cardio-metabolic diseases J Lipid Res 2012 53 12 2490 2514 22798688
- Huang SL Yu RT Gong J Arctigenin, a natural compound, activates AMP-activated protein kinase via inhibition of mitochondria complex I and ameliorates metabolic disorders in ob/ob mice Diabetologia 2012 55 5 1469 1481 22095235
- Zheng T Yang X Wu D Salidroside ameliorates insulin resistance through activation of a mitochondria-associated AMPK/PI3K/Akt/GSK3beta pathway Br J Pharmacol 2015 172 13 3284 3301 25754463
- Jenkins Y Sun TQ Markovtsov V AMPK activation through mitochondrial regulation results in increased substrate oxidation and improved metabolic parameters in models of diabetes PLoS One 2013 8 12 e81870 24339975
- Lefort N Glancy B Bowen B Increased reactive oxygen species production and lower abundance of complex I subunits and carnitine palmitoyltransferase 1B protein despite normal mitochondrial respiration in insulin-resistant human skeletal muscle Diabetes 2010 59 10 2444 2452 20682693
- Raza H Prabu SK John A Avadhani NG Impaired mitochondrial respiratory functions and oxidative stress in streptozotocin-induced diabetic rats Int J Mol Sci 2011 12 5 3133 3147 21686174
- Victor VM Rocha M Banuls C Mitochondrial complex I impairment in leukocytes from polycystic ovary syndrome patients with insulin resistance J Clin Endocrinol Metab 2009 94 9 3505 3512 19567514
- Batandier C Guigas B Detaille D The ROS production induced by a reverse-electron flux at respiratory-chain complex 1 is hampered by metformin J Bioenerg Biomembr 2006 38 1 33 42 16732470
- Hernandez-Mijares A Rocha M Apostolova N Mitochondrial complex I impairment in leukocytes from type 2 diabetic patients Free Radic Biol Med 2011 50 10 1215 1221 21262346
- Saini AS Taliyan R Sharma PL Protective effect and mechanism of Ginkgo biloba extract-EGb 761 on STZ-induced diabetic cardiomyopathy in rats Pharmacogn Mag 2014 10 38 172 178 24914284
- El-Awdan SA Abdel Jaleel GA Saleh AO Grape seed extract attenuates hyperglycemia-induced in rats by streptozotocin Bull Fac Pharm Cairo Univ 2013 51 203 209
- Niture NT Ansari AA Naik SR Anti-hyperglycemic activity of rutin in streptozotocin-induced diabetic rats: an effect mediated through cytokines, antioxidants and lipid biomarkers Indian J Exp Biol 2014 52 7 720 727 25059040
- Maritim A Dene BA Sanders RA Watkins JB3rd Effects of pycnogenol treatment on oxidative stress in streptozotocin-induced diabetic rats J Biochem Mol Toxicol 2003 17 3 193 199 12815616
- Parveen K Khan MR Mujeeb M Siddiqui WA Protective effects of Pycnogenol on hyperglycemia-induced oxidative damage in the liver of type 2 diabetic rats Chem Biol Interact 2010 186 2 219 227 20433812
- Acharya JD Pande AJ Joshi SM Yajnik CS Ghaskadbi SS Treatment of hyperglycaemia in newly diagnosed diabetic patients is associated with a reduction in oxidative stress and improvement in beta-cell function Diabetes Metab Res Rev 2014 30 7 590 598 24459082
- Yasunari K Kohno M Kano H Minami M Yoshikawa J Aldose reductase inhibitor improves insulin-mediated glucose uptake and prevents migration of human coronary artery smooth muscle cells induced by high glucose Hypertension 2000 35 5 1092 1098 10818070
- Yasunari K Kohno M Kano H Yokokawa K Horio T Yoshikawa J Aldose reductase inhibitor prevents hyperproliferation and hypertrophy of cultured rat vascular smooth muscle cells induced by high glucose Arterioscler Thromb Vasc Biol 1995 15 12 2207 2212 7489244
- Ying W Wei G Wang D Intranasal administration with NAD+ profoundly decreases brain injury in a rat model of transient focal ischemia Front Biosci 2007 12 2728 2734 17127275
- Pittelli M Felici R Pitozzi V Pharmacological effects of exogenous NAD on mitochondrial bioenergetics, DNA repair, and apoptosis Mol Pharmacol 2011 80 6 1136 1146 21917911