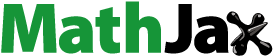
Abstract
In this study, new nano-fluor-hydroxyapatite (nFHA)/polyurethane composite scaffolds were fabricated for potential use in bone tissue engineering. Polyester urethane samples were synthesized from polycaprolactone, hexamethylene diisocyanate, and 1,4-butanediol as chain extender. Nano fluor-hydroxyapatite (nFHA) was successfully synthesized by sol-gel method. The solid–liquid phase separation and solvent sublimation methods were used for preparation of the porous composites. Mechanical properties, chemical structure, and morphological characteristics of the samples were investigated by compressive test, Fourier transform infrared, and scanning electron microscopy (SEM) techniques, respectively. The effect of nFHA powder content on porosity and pore morphology was investigated. SEM images demonstrated that the scaffolds were constituted of interconnected and homogeneously distributed pores. The pore size of the scaffolds was in the range 50–250 μm. The result obtained in this research revealed that the porosity and pore average size decreased and compressive modulus increased with nFHA percentage. Considering morphological, physical, and mechanical properties, the scaffold with a higher ratio of nFHA has suitable potential use in tissue regeneration.
Introduction
It is estimated that more than 500,000 bone graft procedures are performed per year in the United States alone.Citation1 According to the high frequency of bone-grafting, the quantity of donor tissue is limited.Citation1,Citation2 These limitations have resulted in significant attention to alternative approaches such as bone tissue engineering. Bone tissue-engineered grafts require three main elements: 3-dimensional porous scaffolds, progenitor or mature cells, and appropriate growth factors.Citation3
Biodegradable polymers are widely used as porous scaffold in bone tissue engineering. Citation4 Biodegradable polyurethane is one of the most biocompatible materials used as temporary extracellular matrices in bone tissue engineering scaffolds.Citation5,Citation6 However, the major concern associated with biodegradable polyurethane is the lack of bioactive groups, which limits their applications.Citation6 One solution is blending the polyurethanes with bioactive ceramic particles such as tricalcium phosphate or hydroxyapatite (HA).Citation6–Citation10 A combination of ceramic and polyurethane could improve bioactivity and enhance the mechanical properties of porous scaffolds.Citation11
HA (Ca10(PO4)6(OH)2) has attracted much interest as an implant material for teeth and bones due to the similarity of its crystallography and chemical composition to that of human hard tissues.Citation12,Citation13 In most cases, the controlled solubility of HA is an important factor, since it induces bioactivity, osteoconductivity, and therapeutic effects. On the other hand, pure fluorapatite (FA) with a chemical formula, Ca10(PO4)6(F)2 is thought to have a much lower solubility than HA due to greater chemical and structural stability.Citation14 Moreover, the FHA forms (Ca10(PO4)6(OH,F)2) solid solutions with HA by the replacement of OH− by F−. Hence, modulation of the extent of fluoride substitution provides an effective way of controlling the solubility of the apatite. In addition, F− enhances the mineralization and crystallization of calcium phosphate compounds during the bone regeneration.Citation15,Citation16 Also, FHA would facilitate joining between the implants and bone tissue because of its chemical similarity to the calcium phosphate parts in the bone tissue, and its ability to form a strong chemical bond with bone. In addition, FHA keeps comparable biocompatibility and bioactivity with pure HA in terms of the tissue and cell response.Citation17,Citation18
The HA powder has been synthesized over the past years using different techniques, such as precipitation, hydrothermal, and sol-gel methods.Citation19 Among these, the sol-gel technique offers many advantages over other techniques, such as high chemical homogeneity, fine grain structure, and low crystallization temperature, and by being both an economical and technically simple procedure to perform.Citation20,Citation21
The objective of this study is to fabricate novel polyester urethane (PU)/nano fluor-hydroxyapatite (nFHA) porous composites processed by solid–liquid phase separation. The nFHA particles were dispersed uniformly in the polyurethane matrix to improve the mechanical properties and bioactivity. The miscibility, morphology, water uptake, and chemical and mechanical properties of the composite samples were investigated.
Material and methods
Materials
1,6-Hexamethylene diisocyanate (HMDI) and 1,4-dioxane were purchased from Merck (Darmstadt, Germany). Polycaprolactone (PCL, Mn = 2,000; Sigma. Aldrich St. Louis, MO) and 1,4-butanediol (BDO; Merck) were dried under vacuum for 48 hours to remove the residual water. Calcium nitrate (Ca (NO3)2,4H2O; Merck), ammonium fluoride (NH4F; Merck), and triethyl phosphite (TEP, (C2H5O)3P; Merck) were used for preparation of nFHA particles.
Synthesis of nFHA powder
A mixture of FHA nanoparticles containing 50 wt% HA and 50 wt% FA was synthesized via the sol-gel method. Calcium nitrate, TEP, and ammonium fluoride (NH4F) were used as Ca, P, and F precursors, respectively. To prepare FHA nanopowders, TEP was first hydrolyzed in ethanol with a small amount of distilled water. The molar ratio of OH to P was kept at 1/6. An appropriate amount of the ammonium fluoride powder was then added directly to TEP solution and stirred for 24 hours. To achieve a stoichiometric ratio of Ca to P equal to 1.67, the appropriate amounts of TEP solution were added dropwise to the calcium nitrate solution. This solution was stirred for 1 hour and aged at 25°C for 24 hours and afterward at 40°C for 72 hours.Citation22 The mixture was dried in a vacuum oven at 80°C. Finally, the resulting powder samples were heated at 550°C for 1 hour in air.
Synthesis of polyurethane
The polyurethane was obtained in a 2-step process. In the first step, the PCL and HMDI were mixed together, and the mixture was reacted at 80°C for 4 hours in a glass reactor under nitrogen atmosphere to produce a viscous prepolymer. A stoichiometry ratio of HMDI to PCL equal to 6:1 was taken into account. The excess of HMDI was distilled under reduced pressure at 80°C in the vacuum oven. In the second step, the prepolymer was reacted with BDO at 80°C for 30 minutes under nitrogen atmosphere. The polymer was immersed in distilled water. The resulting polymer was precipitated in 80% ethanol solution at 37°C for 48 hours to remove unreacted monomers. Finally, the polymer was dried under vacuum at 40°C for 24 hours.
Fabrication of PU/nFHA composite foams
Pure polyurethane (sample control) and nanocomposite foams containing 5, 10, and 20 wt% nFHA were prepared by solid–liquid phase separation and subsequent freezedrying. A solution of PU in dioxane with a volume ratio of 3% (w/v) was prepared first. After homogeneous mixing of the solution for 24 h, appropriate amounts of nFHA powders were added into the polyurethane solution. The mixture was stirred for 30 minutes and was rapidly cooled down to −5°C afterwards. The samples were freeze-dried under vacuum (0.1 mbar) for solvent sublimation. The porous samples were dried finally at room temperature in a vacuum oven at 40°C until reaching a constant weight.
Characterization of samples
Fourier transform infrared (FTIR) spectrometer (Spectrum One; BOMEM- MB100-SERIES, USA) was used to characterize chemical structure of the samples. Phase compositions of nFHA were studied with X-ray diffraction (XRD-D4; SIEMENS, Bruker Co, Germany) with CuKα radiation (wavelength = 1.54056 Å).
The size distribution and morphology of pure polyurethane foam, and PU/nFHA composite foam were characterized with transmission electron microscopy (TEM) using a Philips CM200 FEG (Field Emission Gun; Philips, Best, The Netherlands) transmission electron microscope operated at 200 kV.
The pore structure and morphology of polyurethane and PU/nFHA composite scaffolds were studied using scanning electron microscopy (SEM, LEO 440I; UK). The pore size of the porous samples was measured from the high-resolution SEM micrographs. Average pore size was calculated by measuring the size of 25 pores using the image analysis software.Citation23
The compression tests were performed using an Instron tester model 1195 (Instron Corp, High Wycombe, UK). The cylindrical specimens, taken from scaffolds were examined at a crosshead speed of 2 mm/min at 23±2°C. All the given values are means of 6 measurements.
Porosity of foam samples
A liquid displacement method was used to measure the porosity of foam samples. Ethanol was used as the liquid because it did not induce any reaction or shrinkage, and it penetrated easily into the pores. A measured weight sample (W) was immersed in a cylinder tube containing a known volume of ethanol (V1). The cylinder was placed in a vacuum to force the ethanol to penetrate entirely the pores. The total volume of ethanol containing the sample was recorded as V2. The ethanol saturated scaffold was removed from the tube, and the residual ethanol volume was recorded as V3. The relative porosity of the open pores in the scaffold ɛ was expressed as reported by ZhangCitation24 and Hsu et alCitation25 (see following equation).Citation26
Water uptake experiments
All foam samples obtained in the study swelled in distilled water and were kept for 192 hours at 37°C in an incubator. Ratio of mass sample to water volume was 1:30. At the end of each predetermined time, the samples were removed from the water, rinsed with distilled water, and finally weighed after wiping gently with filter paper to determine the water uptake. For each experiment, three replicates were used. The percentage of water uptake was calculated according to the following equation:
where, m1 standard = dry sample; m2 = wet sample.Citation27
Results and discussion
Characterization of nFHA powder
The results of FTIR and XRD revealed that a partial substitution of the OH− in HA by F− occurred. The results of FTIR and XRD revealed that a partial substitution of the OH− in HA by F− occurred and the nanoparticles were composed of both HA and FA phases (). A considerable degree of crystallinity (78%) was deduced from XRD patterns.
Figure 1 Infrared spectra of samples: A) FHA; B) PU–0% FHA; C) PU–5% FHA; D) PU–10% FHA; E) PU–20% FHA.
Abbreviations: FHA, fluor-hydroxyapatite; PU, polyester urethane.
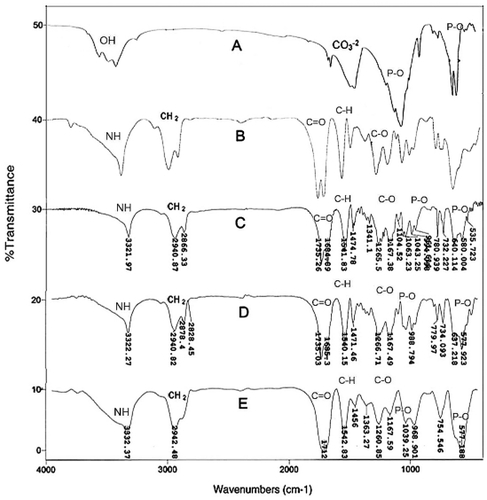
Figure 2 XRD pattern A) and EDX B) of FHA nanoparticles.
Abbreviations: XRD, X-ray diffraction; EDX, energy dispersive X-ray spectroscopy; FHA, fluor-hydroxyapatite.
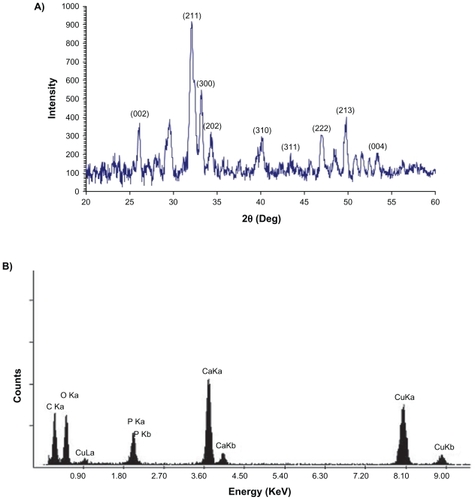
A Ca/P ratio of around 1.65 was estimated from energy dispersive X-ray spectroscopy (EDX) through TEM analysis (). The particle size of powders evaluated by TEM was less than 100 nm (). In accordance with the results of XRD experiments, the diffraction pattern presented in revealed that the FHA nanoparticles were almost crystalline.
Figure 3 Bright field TEM image of FHA nanoparticles.
Abbreviations: FHA, fluor-hydroxyapatite; TEM, transmission electron microscopy.
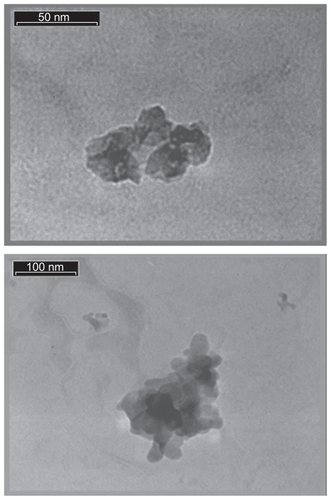
Figure 4 Diffraction pattern A) and Crystalline plane B) of FHA nanoparticles taken by TEM.
Abbreviations: FHA, fluor-hydroxyapatite; TEM, transmission electron microscopy.
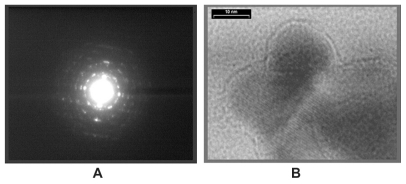
Crystalline planes of a nanoparticle can be observed in a very high resolution TEM image (). The crystallite size assessed directly from TEM imaging and estimated from XRD patterns using Scherrer equation were about 20–50 nm and 13 nm, respectively.
FTIR analysis
The FTIR spectra of PU and nFHA/PU composite foam samples with different nFHA contents are shown in . The absorption band at 3550 cm−1 is related to the vibration bending of hydroxyl (–OH) of nFHA (). The characteristic absorption band around 3322 cm−1 corresponds to hydrogen-bonded NH groups, and the sharp absorption bands related to asymmetric and symmetric –CH2 groups are observed at 2940 and 2866 cm−1, respectively. In addition, the strong bands at 1735 and 1684 cm−1 belong to the free and hydrogen-bonded –C=O groups of PU.Citation28,Citation29 The bands in the range 1040–1220 cm−1 are related to vibration of C–O and C–O–C groups.Citation7,Citation28,Citation29 Disappearance of the isocyanate group’s peak (2270 cm−1) revealed the fact that HMDI reacted well, and polymerization has been completed throughout the synthesis. Various modes of –CH2 vibrations are manifested in the range 1260–1465 cm−1.
In the synthesis of PU, the ratio of NCO to OH was 6:1. The NCO to OH ratio higher than 1.0 may result in the cross linking of polyurethane during the formation of allophanates and biurets. However, obvious bands owing to allophanates and biurets were not observed probably because the bands were weak or overlapped with other absorption bands.Citation30
The peaks at 1460 and 1420 cm−1 are related to carbonic group (CO3−2) in nFHA, and the absorption bands at 567, 1037, and 1091 cm−1 belong to phosphate groups (PO4−3), which shift to 580, 1039, and 1104 cm−1 (), to 578, 1039, and 1102 cm−1 (), and to 577, 1039, and 1102 cm−1 (), respectively.Citation31,Citation32
There are some molecular interactions between polyurethane and nFHA in the FTIR spectra. The hydroxyl group from FHA is thought to be able to easily link with the carboxyl or amino groups from PU.Citation6 Disappearance of the peaks at 3550 cm−1 corresponding to –OH groups in nFHA might be attributed to chemical linkage between hydroxyl groups of nFHA and polyurethane groups.Citation31 This disappearance could be affected by small amounts of nFHA (0–20 [w/w%]) in composite samples and the substitution of 50% OH− by F− in FHA nanoparticles. Also, it could be mentioned that polyurethane has linked with nFHA by hydrogen bonding.Citation31
SEM observation and porosity
The microstructures of pure and nFHA/PU scaffold shown in revealed the presence of well-distributed macropores throughout the scaffolds and some micropores within the pore walls. The size, morphology, and interconnectivity of pores determine the ability of the scaffolds to the nutrient diffusion, metabolic product delivery, cell attachment, tissue ingrowth, and angiogenesis. With the increase of mineral content, porosity and pore average size decreased, and wall thickness increased slightly.Citation33 It seems that the closed pores are formed in the samples containing higher nFHA content. Moreover, the SEM images showed that nFHA powder homogeneously dispersed in the pore wall as well as in the pore surfaces. The porosity of pure PU and PU–20% nFHA samples were approximately 88% and 80%, respectively. The pores were regular, uniformly distributed, and interconnected with average pore sizes ranging 50–250 μm, which are suitable scaffold architecture for application in bone tissue engineering.Citation11,Citation34
Mechanical properties
The mechanical properties of the PU and PU/nFHA composite foams are summarized in .
Table 1 Mechanical properties of the scaffolds
Water absorption
The water uptake ability of the scaffolds was depended on the material’s composition. shows that water uptake increased very quickly in the foam samples. The amount of absorbed water during incubation in water increases with the nFHA content. As seen in this figure, the most hydrophilic materials containing 20% nFHA absorbed the highest amount of water. The composite scaffolds absorbed a very high amount of water in the range 424%–502% after 144 hours incubation in water (). Water absorption after 6 days achieved a plateau value at the end of incubation period. The percentage of water absorption in all scaffold composites was higher than in the pure PU scaffolds during 192 hours.Citation36,Citation37
Conclusion
A novel porous scaffold based on polyurethane and nFHA was fabricated in this study. Aliphatic polyurethane was polymerized with PCL diols as soft segment and HMDI and BDO as hard segment. A higher amount of HMDI compared with PCL diols (NCO/OH = 6/1) led to an important cross linking in the PU sample. The porous composites were obtained by solid–liquid phase separation and freeze drying. The SEM images indicated that the pores were interconnected and uniformly distributed. The porous scaffolds not only have pores with sizes in the range 50–250 μm but also contain many micropores in the scaffold wall. In the FTIR spectra, it was shown that nFHA particles formed hydrogen bonds with the polyurethane. The compressive strength and compressive modulus of polyester polyurethane scaffolds increased with increasing nFHA powder ratio. When the nFHA content changed from 0 wt% to 20 wt%, the samples showed suitable mechanical properties and a high porosity of 80 to 88%. These novel nFHA/PU scaffolds have potential for bone regeneration, and may be suitable for use as a scaffold for applications in bone tissue engineering.
Acknowledgments
The authors would like to thank the Science and Research Branch of Islamic Azad University for financial support of this research. The authors wish to thank Ms Sima Eshghi for SEM studies.
Disclosure
The authors report no conflicts of interest in this work.
Referenes
- GreenwaldASBodenSDGoldbergVMKhanYLaurencinCTRosierRNBone-graft substitutes: facts, fictions, and applicationsJ Bone Joint Surg Am200183A9810311712842
- YangFWolkeJGCJansenJABiomimetic calcium phosphate coating on electrospun poly (ɛ-caprolactone) scaffolds for bone tissue engineeringChem Eng J2008137154161
- LuHHTangACheolOhSSpalazziJPDionisioKCompositional effects on the formation of a calcium phosphate layer and the response of osteoblast-like cells on polymer-bioactive glass compositesBiomaterials2005266323633415919111
- RezwanKChenQZBlakerJJBoccacciniARBiodegradable and bioactive porous polymer/inorganic composite scaffolds for bone tissue engineeringBiomaterials2006273413343116504284
- HillCMAnYHKangQKHartsockLAGogolewskiSGornaKOsteogenesis of osteoblast seeded polyurethane-hydroxyapatite scaffolds in nude MiceMacromol Symp20072539497
- HuangMNWangYLLuoYFBiodegradable and bioactive porous polyurethanes scaffolds for bone tissue engineeringJ Biomed Sci Eng200923640
- BilMRyszkowskaJRoetherJABretcanuOBoccacciniARBioactivity of polyurethane-based scaffolds coated with BioglassBiomed Mater2007229310118458441
- ChettyASteynbergTHydroxyapatite-coated polyurethane for auricular cartilage replacement: an in vitro studyJ Biomed Mater Res A200884247548217618497
- HuangXMiaoXNovel porous hydroxyapatite prepared by combining H2O foaming with PU sponge and modified with PLGA and bioactive glassJ Biomater Appl200721435137416543281
- BrovaroneCVVerneEDevelopment of glass ceramic scaffolds for bone tissue engineering: characterization, proliferation of human osteoblasts and nodule formationActa Biomater2007319920817085090
- MathieuLMMuellerTLBourbanPEPiolettiDPMullerRMansonJAEArchitecture and properties of anisotropic polymer composite scaffolds for bone tissue engineeringBiomaterials20062790591616051346
- LiuDMTroczynskiTTsengWJWater-based sol-gel synthesis of hydroxyapatite: process developmentBiomaterials2001222130
- KuiCGeSWenjianWSynthesis of hydroxyapatite/fluoroapatite solid solution by a sol-gel methodMater Lett2001513741
- JhaLJBestSMKnowlesJCRehmanISantosJDBonfieldNPreparation and characterization of fluoride-substituted apatitesJ Mater Sci Mater Med199789118515348776
- ElliottJCStructure and Chemistry of the Apatites and Other Calcium OrthophosphatesAmsterdam, The NetherlandsElsevier Science1994
- AobaTThe effect of fluoride on apatite structure and growthCrit Rev Oral Biol Med199781361539167089
- StanGEFerreiraJMFAn algorithm for preparing bioactive fluorinated hydroxyapatite coatings by sol gel techniqueJ Optoelectron Adv Mater20079825392542
- MiaoSWengWChengKIn vitro bioactivity and osteoblast-like cell test of zinc containing fluoridated hydroxyapatite filmsJ Mater Sci Mater Med2007182101210517562136
- SlosarczykAStobierskaEPaszkiewiczZGawlickMCalcium phosphate materials prepared from precipitates with various calcium: phosphorus molar ratiosJ Am Ceram Soc1996791025392544
- LiuDMYangQTroczynskiTTsengWJStructural evolution of sol-gel- derived hydroxyapatiteBiomaterials2002231679168711922471
- WengWBaptistaJLSol-gel derived porous hydroxyapatite coatingsJ Mater Sci Mater Med1998915916315348905
- KimHWKongYMBaeCJNohYJKimHESol-gel derived fluor-hydroxyapatite biocoatings on zirconia substrateBiomaterials2004252919292614967523
- LimYMGwonHJChoiJHShinJNhoYCPreparation and biocompatibility study of gelatin/kappa-carrageenan scaffoldsMacromol Res20101812934
- ZhangRYMaPXPoly (alpha-hydroxyl acids)/hydroxyapatite porous composites for bone-tissue engineering. I. Preparation and morphologyJ Biomed Mater Res19994444645510397949
- HsuYYGresserJDTrantoloDJLyonsCMGangadharamPRWiseDLEffect of polymer foam morphology and density on kinetics of in vitro controlled release of isoniazid from compressed foam matricesJ Biomed Mater Res1997351071169104703
- GuanJFujimotoKLSacksMSWagnerWRPreparation and characterization of highly porous, biodegradable polyurethane scaffolds for soft tissue applicationsBiomaterials2005263961397115626443
- GornaKGogolewskiSIn vitro degradation of novel medical biodegradable aliphatic polyurethanes based on ɛ-caprolactone and Pluronics with various hydrophilicitiesPolym Degrad Stab200275113122
- McCarthySJMejisGFMitchellNIn-vivo degradation of polyurethanes: transmission-FTIR microscopic characterization of polyurethanes sectioned by cryomicrotomyBiomaterials199718138714099375841
- GornaKPolowinskiSGogolewskiSSynthesis and characterization of biodegradable poly (ɛ-caprolactone urethane)s. I. Effect of the polyol molecular weight, catalyst, and chain extender on the molecular and physical characteristicsJ Polym Sci A Polym Chem200240156170
- KurimotoYTakedaMDoiSTamuraYOnoHNetwork structures and thermal properties of polyurethane films prepared from liquefied woodBioresour Technol200177334011211073
- WangLLiYZuoYPorous bioactive scaffold of aliphatic polyurethane and hydroxyapatite for tissue regenerationBiomed Mater20094202500319208942
- DongZLiYZouQDegradation and biocompatibility of porous nano-hydroxyapatite/polyurethane composite scaffold for bone tissue engineeringAppl Surf Sci200925560876091
- BoissardCIRBourbanPETamiAEAliniMEglinDNanohydroxyapatite/poly(ester urethane) scaffold for bone tissue engineeringActa Biomater200953316332719442765
- PinedaLMBusingMMeinigRPGogolewskiSBone regeneration with resorbable polymeric membranes. III. Effect of poly (L-lactide) membrane pore size on the bone healing process in large defectsJ Biomed Mater Res1996313853948806065
- BainoFVerneEBrovaroneCVFeasibility, tailoring, and properties of polyurethane/bioactive glass composite scaffolds for tissue engineeringJ Mater Sci Mater Med20092021892195
- GornaKGogolewskiSPreparation, degradation, and calcification of biodegradable polyurethane foams for bone graft substitutesJ Biomed Mater Res A200367381382714613229
- MaquetVBoccacciniARPravataLNotingherIJeromeRPorous poly (a-hydroxyacid)/bioglasss composite scaffolds for bone tissue engineering. I: preparation and in vitro characterizationBiomaterials2004254185419415046908