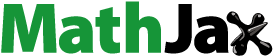
Abstract
Background
Biodegradable polyurethanes have found widespread use in soft tissue engineering due to their suitable mechanical properties and biocompatibility.
Methods
In this study, polyurethane samples were synthesized from polycaprolactone, hexamethylene diisocyanate, and a copolymer of 1,4-butanediol as a chain extender. Polyurethane scaffolds were fabricated by a combination of liquid–liquid phase separation and salt leaching techniques. The effect of the NCO:OH ratio on porosity content and pore morphology was investigated.
Results
Scanning electron micrographs demonstrated that the scaffolds had a regular distribution of interconnected pores, with pore diameters of 50–300 μm, and porosities of 64%–83%. It was observed that, by increasing the NCO:OH ratio, the average pore size, compressive strength, and compressive modulus increased. L929 fibroblast and chondrocytes were cultured on the scaffolds, and all samples exhibited suitable cell attachment and growth, with a high level of biocompatibility.
Conclusion
These biodegradable polyurethane scaffolds demonstrate potential for soft tissue engineering applications.
Introduction
Polyurethanes are considered to be excellent biomaterials due to their suitable mechanical properties and good biocompatibility.Citation1–Citation5 Resistant polyurethanes have been used as long-term implant materials, including catheters,Citation6 artificial heart valves,Citation7 wound dressings, angioplasty balloons, ventricular assist devices, and pacing lead insulation.Citation8 Recently, investigators have designed biodegradable polyurethanes for applications such as artificial skin,Citation9 bone graft substitutes,Citation10 drug delivery systems,Citation11 and porous scaffolds for regenerating damaged tissues.Citation12 The biodegradability and biocompatibility of the polyurethanes are determined by their composition and preparation. Citation13 Polyurethanes containing ester or ether groups are vulnerable to degradation through hydrolysis in vivo.Citation14
Typically, aliphatic diisocyanate is employed for applications where degradation is desired because its ultimate degradation products are more likely to be nontoxic. Hexamethylenediisocyanate (HMDI), due to its linear structure, is the most widely used isocyanate in the preparation of biodegradable polyurethanes.Citation15–Citation17 Polyester polyols, such as polycaprolactone, are amongst the biocompatible and biodegradable polymers used in the synthesis of polyurethanes. The biodegradability of polyurethanes is due to the ester bonds in the polymer structure.Citation18–Citation20
Tissue engineering offers an alternative approach to improve, repair, or replace damaged human tissue. It utilizes a combination of progenitor or mature cells to initiate natural tissue repair and regeneration. This process takes place on or within a biomaterial scaffold, and can be done with and without appropriate growth factors.Citation21
Briefly, a biomaterial scaffold suitable for use in tissue engineering should be biodegradable and have nontoxic degradation products, be highly porous with an interconnected pore structure, have suitable physical and mechanical properties, and be biocompatible and suitable for cell attachment, proliferation, and differentiation.Citation22
Various methods have been investigated for the preparation of porous scaffolds, including electrospinning,Citation23 solvent casting/particulate leaching,Citation24 phase inversion,Citation25 freeze-drying,Citation26 and thermally-induced phase separation.Citation27 The solvent casting/salt leaching method has the advantage of controlling pore size by manipulating the size of the salt particulate. Techniques like freeze-drying often allow the fabrication of porous scaffolds with a high compressive modulus. Researchers have used combinations of these techniques to obtain desirable porosity ratios and pore dimensions.Citation25–Citation27 In this study, biodegradable linear polyurethanes were prepared using polycaprolactone, HMDI, and 1,4-butanediol/HMDI/1,4-butanediol (BDO/HMDI/BDO) copolymers. Scaffolds with interconnected porosity were prepared using a combination of salt leaching and freeze-drying methods. The mechanical, morphological, and physical properties of the samples were determined.
The objective of this study was to fabricate a novel polyurethane scaffold by salt leaching and liquid–liquid phase separation. Effects of the NCO:OH ratio on the mechanical, morphological, and physical properties of the scaffolds were also investigated.
Methods and materials
Polycaprolactone (Mn = 2000 g/mol, Sigma, St Louis, MO) was dried under vacuum for 48 hours, and 1,4-butanediol (Merck, Darmstadt, Germany) and dimethylsulfoxide (Merck) were dried for 24 hours. HMDI (Merck) was distilled under reduced nitrogen pressure prior to use. NaCl, 1,4-dioxane, and dimethyl formamide were used as received from the supplier (Merck).
Prepolymer and polyurethane synthesis
All reactions were done under nitrogen atmosphere in a glass reactor equipped with a mechanical stirrer. The nitrogen gas, prior to entering the reactor, was dried by passage through concentrated sulfuric acid. The polyurethane was synthesized using a two-step polymerization method (). The prepolymers were produced by reacting of polycaprolactone with HMDI for 4 hours at 80°C. The excess of HMDI was later distilled off under reduced pressure (0.03 mbar) at 80°C. The stoichiometry of the chemical reaction was 2:1, 4:1, and 6:1 of HMDI: polycaprolactone. The chain extender copolymer (BDO/HMDI/BDO) was prepared by mixing the HMDI with an excess amount of 1,4-butanediol at 80°C. The excess of 1,4-butanediol was later removed by washing with acetone. The BDO/HMDI/BDO was dissolved in dimethylsulfoxide and added to the prepolymer at 80°C for 30 minutes. The resulting samples from 2:1, 4:1, and 6:1 of HMDI:polycaprolactone were named Polyurethane A1 (PUA1), Polyurethane A2 (PUA2), and Polyurethane A3 (PUA3), respectively. The polyurethane product was washed with water and dried under vacuum at 40°C. The polyurethane films were prepared by solvent casting of polyurethane solution in dimethylformamide (5% w/v).
Scaffold production
Polyurethane scaffolds were created by a combination of phase separation and salt leaching techniques. Polyurethane was dissolved in dioxane at a concentration of 20% (w/v), and distilled water 5% (w/w) was added as a nonsolvent. Pores were created by mixing the solution with 2 g of NaCl crystals (varying in size from 50 to 355 μm) per gram of polymer. The polyurethane/salt mixture was poured into several moulds. The filled moulds were rapidly cooled to −20°C. Subsequently, the moulds were freeze-dried under vacuum (0.1 mbar) from −30 to +5°C for 57 hours. The samples were washed for 10 hours in water to remove the salt crystals. The polymer samples were later dried in a vacuum oven for 24 hours at 40°C in order to avoid degradation ().
Table 1 Mechanical properties of the polyurethane scaffolds
Material characterization
Fourier transform infrared spectroscopy
Infrared data were obtained on KBr slices with a spectrophotometer (Spectrum One, MB100 series; BOMEM, Minneapolis, MN). Approximately 30 scans were taken for each sample.
Mechanical properties
The compressive strength of the polyurethane scaffolds was tested using an Instron materials testing machine (Model 1195; Instron Corporation, Norwood, MA) at 23 ± 2°C and relative humidity of 50%. The sample dimensions were 12 mm × 12 mm × 2 mm and the cross head speed was 2 mm/min with a 1000 N load of cells.
Scaffold porosity
The porosities of the polyurethane foams were studied using a liquid displacement method similar to the procedure reported by Zhang and MaCitation28 and Hsu et al.Citation29 Ethanol was used as the displacement liquid for this procedure because it penetrated easily into the pores of the polyurethane scaffold. A dry scaffold was placed in a graduated cylinder filled with a predetermined volume (V1) of ethanol, and this cylinder was then placed in a vacuum for 20 minutes to enable penetration of ethanol into the scaffold pores. The total volume of ethanol containing the sample was recorded as V2. The scaffold was taken out of the graduated cylinder, and the residual ethanol volume was recorded as V3. The amount of open pores in the scaffold (P) was calculated according to the following equation:
where (V2–V3) = total volume of the scaffold and (V1–V3) = volume of ethanol retained in the sample. Three specimens of each sample were used for the porosity measurements and the results were averaged.
In vitro cell culture
Fibroblast culture
The cell culture reaction for the prepared films was evaluated by in vitro cell culture testing. Mouse L929 fibroblasts and human dermal fibroblasts isolated from neonatal foreskin (Iran Pasteur Institute, Tehran, Iran) were used in this study and cultured in RPMI containing fetal calf serum 10%, penicillin 100 μg/mL, and streptomycin 100 μg/mL (Sigma). A suspension of 1.8 × 105 cells/mL was prepared before seeding. Duplicate specimens for each scaffold sample were sterilized in 70% ethanol and washed in culture medium before the cell culture procedure. The samples were placed on a multiwall polystyrene plate with 5 mL of cell suspension and maintained for 48 ± 1 hours in a CO2-controlled incubator at 37°C. One sample was retained as a negative control. After incubation, all samples were washed with phosphate-buffered saline solution. The cells were fixed with glutaraldehyde 2.5% and dehydrated in graded ethanol (60%, 70%, 80%, and 95%). The cells were observed using Nikon light microscopy (Tokyo, Japan).Citation30
Chondrocyte culture and isolation
The scaffold samples were washed with deionized water and sterilized in ethylene oxide gas. Chondrocytes were obtained from the metacarpophangeal joints of 6–9-month-old calves. The samples were put in the bottom of a 24 well-plate, and 0.1 mL of cell medium suspension at a concentration of 4 × 106 cells/mL was added to observe cell attachment. After 2 hours of incubation at 37°C and 5% CO2, the medium (Dulbecco’s Modified Eagle Medium, 6%v/v fetal calf serum) was replaced with 2 mL of new medium, and the plate was put into the incubator again, under the same conditions.Citation31,Citation32 After 3 days of culture, the cells were stained with Trypan Blue. The samples were examined under an optical microscope, and photographs were taken and analyzed.
Cell proliferation assay
The cell proliferation assay was done according to a previously published method.Citation33 The extraction process for the scaffold samples was done according to ISO 10993-5, whereby 1 mL of RPMI culture medium was added to each sample surface area within a range of 4 ± 0.5 cm2. After 7 and 14 days, the media samples were removed for use in the cell proliferation assay. A specified amount of culture medium was retained under the same conditions as for the negative control. The proliferation rate of the human fibroblasts exposed to the sample extracts was measured using the 3-(4,5-dimethylthiazol-2-yl)-2,5-diphenyltetrazoliumbromide (MTT) assay (Sigma). The fibroblasts were plated onto a 96-well microtiter plate at 1 × 104 cells/well. After 24 hours, the culture medium of each well was removed and replaced with 90 μL of extract plus 10 μL of fetal calf serum. The medium was eliminated over the next 24 hours, and 100 μL of a MTT 0.5 mg/mL solution was added to each well, followed by incubation for 5 hours at 37°C. Purple formazan crystals were dissolved by addition of isopropanol 100 μL (Sigma) per well. The plates were then incubated at 37°C for 15 minutes prior to absorbance measurements. The optical density was recorded on a multiwell microplate reader (ICN, Birsfelden, Switzerland) at 545 nm, and normalized to the control optical density.
Scanning electron microscopy of fibroblasts
The morphology and pore structure of the porous scaffolds were examined using scanning electron microscopy (440I; LEO, Cambridge, UK). The samples were sputter-coated with gold (approximately 50 nm) under vacuum. Scanning electron microscopy was carried out at 15 kV. The morphology of human fibroblasts cultured on the samples was also examined by scanning electron microscopy. Cells (5 × 103) were seeded onto the surface of the specimens in a six-well culture plate and incubated using 5% CO2 at 37°C for 7 days. At the end of the culture, the cells were fixed with Karnovsky fixation solution (paraformaldehyde 2 g, 25% glutaraldehyde solution 10 mL, and 0.2 M cacodylate buffer 20 mL, pH 7.4) for 24 hours. Samples were dehydrated in graded alcohols (10%, 30%, 50%, 70%, 80%, 85%, 90%, 95%, and 100%), each for 10 minutes, sputter-coated with gold, and viewed.
Results and discussion
Fourier transform infrared spectroscopic analysis
shows the Fourier transform infrared spectrum obtained from the polyurethane samples. The absorption band at 3323 cm−1 corresponds to NH stretching. The sharp peaks at 2859 cm−1 and 2938 cm−1 are associated with −CH2 stretching, while other modes of −CH2 vibrations are identified by the bands at 1464, 1418, 1364, and 1294 cm−1. In addition, the absorption band at 1734 cm−1 is associated with a C=O group in polyurethane. The group of NH vibrations is identified by the bands at 1541 cm−1.
The band at 1702 cm−1 is assigned to hydrogen bonding between N-H and C=O groups in the hard segment and the ester or ester-oxygen groups of the soft segments of urethane linkage. The band at 1720 cm−1 belongs to nonhydrogen-bonded carbonyl groups (). However, on increasing the NCO:OH ratios from 2:1 to 6:1, the intensity of the hydrogen-bonded C=O band compared with the nonhydrogen-bonded C=O band was increased.Citation26–Citation34
Mechanical properties and porosity
The compressive modulus is an important parameter in tissue formation and thus in the repair of tissue lesions. By changing the ratio of NCO:OH, the content of the soft segment in polyurethane can be controlled. The mechanical properties of the porous polyurethane scaffolds are shown in . It can be seen that by increasing the hard segment in the polyurethane scaffold, the compressive strength and compressive modulus also increases. These scaffolds show a compressive strength in the range of 0.58–0.93 MPa and a compressive modulus in the range of 0.82–1.23 MPa (). It was observed that the less porous scaffolds had better properties.
Increasing the NCO:OH ratio in polyurethanes improves their mechanical properties by increasing the uniformity and inter connectivity of the pores. The highest percentage of pores (over 74%) was observed for the polyurethane A1 scaffold. The average pore size for the sample was about 50–300 μm, indicating that the cells could easily penetrate the pores of the scaffold.
Scanning electron microscopy
The size, distribution, and inter connectivity of the pores determine the capacity of the scaffold to enable cell attachment and growth. Scanning electron photo micrographs of the samples are shown in . These images demonstrate that the scaffolds have porous structures, with pore sizes ranging from 50 μm to 300 μm, which is within the appropriate range for tissue engineering. As can be clearly seen, the pores are rather large, the cell walls are thick, and the pore structure is interconnected. Therefore, cells can penetrate into the pores following their growth on the scaffold surface. Pores were regular, uniformly distributed, and interconnected. Pore content ranged from 64% to 83%, and the NCO:OH ratio decreased at the same time (). Due to inter connectivity of the pores, the cells could penetrate into the scaffold.
Figure 2 Typical scanning electron microscopy images of polyurethane scaffolds. (A) PUA1; (B) PUA2; (C) PUA3 and (D) SEM of human fibroblasts on polyurethane scaffold after 7 days culture on PUA3.
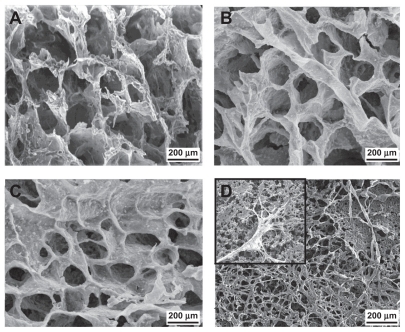
shows electron microscopy images of human fibroblast cells cultured on the polyurethane A3 scaffold. It can be seen that by day 7, the cells were well grown and spread throughout the polyurethane scaffold. Moreover, fibroblast cell migration into the porous scaffold could be observed in some areas.
Cell culture
As already reported,Citation27 cell adhesion onto a material surface can be arbitrarily classified as a two-step mechanistic process. The first stage is controlled by a complex combination of physicochemical interactions including hydrophobic, coulombic, and Vander Waals forces between the cell membrane and the material surface. This process might be termed “passive adhesion.” The second stage might be considered as “active adhesion,” because of the participation of metabolic cellular processes. Attached cells are well-known for changing their shapes and expending metabolic energy in order to stabilize the interface between their membranes and the underlying materials. This could occur by both physicochemical and biological mechanisms.
shows L929 and human fibroblast attachment onto the polyurethane surfaces. The cells showed good proliferation and attachment, and covered the polyurethane surface. Their spreading pattern resembles that of spindle morphology. Apparently what takes place here is active adhesion. L929 and human fibroblasts grew on the polyurethane surfaces and spread on these samples. The cells consumed metabolic energy during this process, which is indicative of active adhesion. These observations strongly suggest that the chemical and/or physical structure of the substrate controls the degree of cell adhesion and proliferation. Our cell culture experiments showed good cell attachment in all the samples, indicating that the scaffolds had a high level of biocompatibility. A suitable cell response was obtained by the 7-day cell seeding procedure (). For the polyurethane A3 scaffold, some rounded cells were observed (). Evidence of good attachment is the flattened form of the cells, which was observed in all the samples. A particular substrate is biocompatible if the fibroblast extends more of its body and filopodia onto the surface of the substrate. For the polyurethane A1 scaffold, not only the population of cells attached to the surface but also the quality of their attachment was better than for the other samples (). More cells can be observed in than in . Therefore, it can be concluded that the addition of a soft segment increases the biocompatibility of the scaffold.
Figure 3 Cell culture of L929 on the (A) PUA1, (B) PUA2, (C) PUA3 (magnification ×100) and human dermal fibroblast on (D) PUA1, (E) PUA2, (F) PUA3 (magnification ×100).
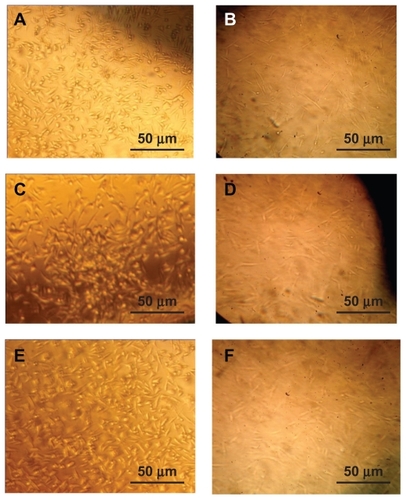
The cytotoxicity evaluation shows that the scaffolds had good tissue compatibility with human fibroblast cultures. It was observed that cells cultured in the scaffolds could partially attach, spread, and proliferate in Petri dishes containing samples, and the scaffold surfaces were also covered by fibroblasts ().
Scanning electron microscopic images of human fibroblasts on the different polyurethane scaffold surfaces after 7 days of plating are shown in . The cells adhered to and aggregated on the surface and pores of the scaffold. Fibroblasts displayed good adhesion and spread well on the scaffold samples. However, the morphology of the fibroblasts was varied. Most of the human fibroblasts on the polyurethane scaffolds had more spherical and elongated shapes, and some had a round or flat morphology. The polyurethane A1 scaffold attracted more cells than the polyurethane A2 and A3 scaffolds. The NCO:OH ratio on the polyurethane A1 scaffold was 2:1, so the soft hydrophilic segment on the polyurethane A1 scaffold was greater than in other samples. The cells were attracted to the soft segment, and the polyurethane A1 scaffold showed the best biocompatibility results, as evidenced by a higher attachment rate (). The cell morphology indicates that the polyurethane scaffold is favorable for cell culture.
Figure 4 SEM of human fibroblasts on polyurethane scaffold after 7 days in culture (A) PUA1, (B) PUA2, (C) PUA3, The cells well attached on the samples.
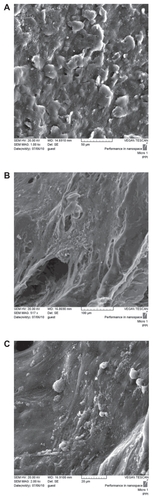
Analyzing the photographs of chondrocyte culture on the scaffolds indicate that the native phenotype and morphology of the cells was preserved (). Therefore, the polyurethane scaffold is suitable for tissue engineering. The results of chondrocyte growth on the scaffold surface indicate that the cells were able to grow without any modification in their morphology and phenotype. Chondrocytes have round shapes, and the images show that the cells attached to the scaffold had similar spherical shapes. These results indicate that biodegradable polyurethane scaffolds have adequate-biocompatibility for use in tissue repair.
Cell proliferation results
shows the results of the cell proliferation assay for all cells in comparison with controls, and demonstrates that all the samples had cell viability of more than 90%. It seems that increasing the isocyanate groups in the polyurethane composition had no toxic effect on cell viability.
Conclusion
In this study, biodegradable polyurethane samples were synthesized from polycaprolactone and HMDI, with a copolymer of 1,4-butanediol used as a chain extender. Scaffolds were created by liquid–liquid phase separation and salt leaching techniques. Pores were regular, uniformly distributed, and interconnected. Scanning electron micrographs indicate that the samples had regular and interconnected pores, with sizes in the range of 50–300 μm. Fourier transform infrared-spectroscopy showed the formation of urethane bonds after polymerization. The compressive strength and compressive modulus of the polyurethane scaffolds increased with increasing NCO:OH ratios. These results could be explained by a higher content of hard segments than soft segments in the polyurethane copolymer. The cytotoxicity results showed appropriate cell attachment with a spindle-like spreading pattern. The fibroblasts attached firmly to the surface and pores of the scaffolds, and the polyurethane samples supported attachment and growth of the fibroblasts. The results of in vitro cell culture indicated cell growth on the scaffold surface, and that the cells were able to grow without any modification in their morphology and phenotype. Overall, the results presented here demonstrate that these polyurethane scaffolds are biocompatible.
Acknowledgment
This work was undertaken with partial financial support from the Islamic Azad University of Science and Research.
Disclosure
The authors report no conflicts of interest in this work.
References
- SpaansCJDe GrootJHBelgraverVWPenningsAJA new biomedical polyurethane with a high modulus based on 1,4-butanediisocyanate and ɛ-caprolactoneJ Mater Sci Mater Med1998967567815348920
- WetzelsGMKooleHPhotoimmobilisation of poly (N-vinylpyrrolidinone) as a means to improve haemocompatibility of polyurethane biomaterialsBiomaterials1999201879188710514064
- GornaKGogolewskiSMolecular stability, mechanical properties, surface characteristics and sterility of biodegradable polyurethanes treated with low-temperature plasmaPolym Degrad Stab200379475485
- KhanISmithNJonesEFinchDSCameronREAnalysis and evaluation of a biomedical polycarbonate urethane tested in an in vitro study and an ovine arthroplasty model, Part II: in vivo investigationBiomaterials20052663364315282141
- SimmonsAHyvarinenJOdellRALong-term in vivo biostability of poly(dimethylsiloxane)/poly(hexamethylene oxide) mixed macrodiol-based polyurethane elastomersBiomaterials2004254887490015109849
- ParkJHChoYWKwonICJeongSYBaeYHAssessment of PEO/PTMO multiblock copolymer/segmented polyurethane blends as coating materials for urinary catheters: In vitro bacterial adhesion and encrustation behaviorBiomaterials2002233991400012162332
- SisterVGIurechkoVNExperimental study of the hydrodynamics of polyurethane tricuspid heart valvesMed Tekh20066814 Russian17290919
- StokesaKCobianaKPolyether polyurethanes for implantable pacemaker leadsBiomaterials198232252317171682
- GogolewskiSPenningsAJAn artificial skin based on biodegradable mixtures of polylactides and polyurethanes for full thickness skin wound coveringMakromol Chem Rapid Commun19834675680
- GogolewskiSGornaKBiodegradable polyurethane cancellous bone graft substitutes in the treatment of iliac crest defectsJ Biomed Mater Res A200680A94101
- SivakWNPollackIFPetoudSZamboniWCZhangJBeckmanEJCatalyst-dependent drug loading of LDI–glycerol polyurethane foams leads to differing controlled release profilesActa Biomater200841263127418440884
- GogolewskiSGornaKZaczynskaECzarnyAStructure-property relations and cytotoxicity of isosorbide-based biodegradable polyurethane scaffoldsJ Biomed Mater Res A200785A456465
- GunatillakePMayadunneRAdhikariRRecent developments in biodegradable synthetic polymersBiotechnol Annu Rev20061230134717045198
- DarbyRTKaplanAMFungal susceptibility of polyurethanesAppl Microbiol19681690090516349806
- TuominenJKylmäJKapanenAVenelampiOItävaaraMSeppäläJBiodegradation of lactic acid based polymers under controlled composting conditions and evaluation of the ecotoxicological impactBiomacromolecules2002344545512005513
- GoronaKGogolewskiSNovel biodegradable polyurethanes for medical applicationsAmerican Society of Testing and Materials20003957
- LambaNWoodhouseKCooperSPolyurethanes in Biomedical ApplicationsNew YorkCRC Press1998
- GoronaKGogolewskiSBiodegradable polyurethanes for implants. II. In-vitro degradation and calcification of materials from poly(ɛ-caprolactone)-poly(ethylene oxide) diols and various chain extendersJ Biomed Mater Res20026059260611948518
- WalinskaKIwanAGornaKGogolewskiSThe use of long-chain plant polyprenols as a means to modify the biological properties of new biodegradable polyurethane scaffolds for tissue engineering: A pilot studyJ Mater Sci Mater Med20081912913517587148
- GoronaKGogolewskiSIn vitro degradation of novel medical biodegradable aliphatic polyurethanes based on ɛ-caprolactone and Pluronics with various hydrophilicitiesPolym Degrad Stab200275113122
- YangXBWebbDBlakerJEvaluation of human bone marrow stromal cell growth on biodegradable polymer/bioglass compositesBiochem Biophys Res Commun20063421098110716516859
- SpaansCJBelgraverVWRienstraOde GrootJHVethRPPenningsAJSolvent-free fabrication of micro-porous polyurethane amide and polyurethane-urea scaffolds for repair and replacement of the knee-joint meniscusBiomaterials2000212453246011055293
- RockwoodDNWoodhouseKAFromsteinJDChaseDBRaboltJFCharacterization of biodegradable polyurethane microfibers for tissue engineeringJ Biomater Sci Polym Ed20071874375817623555
- FromsteinJDWoodhouseKAElastomeric biodegradable polyurethane blends for soft tissue applicationsJ Biomater Sci Polym Ed20021339140612160300
- SavarinoLBaldiniNGrecoMThe performance of poly-ɛ-caprolactone scaffolds in a rabbit femur model with and without autologous stromal cells and BMP4Biomaterials2007283101310917412415
- GerçekITigliRSGümüsdereliogluMA novel scaffold based on formation and agglomeration of PCL microbeads by freeze-dryingJBiomed Mater Res A2008861012102218067167
- SaadBMatterSCiardelliGInteractions of osteoblasts and macrophages with biodegradable and highly porous polyesterurethane foam and its degradation productsJ Biomed Mater Res1996323553668897140
- ZhangRYMaPXPoly(a-hydroxyl acids)/hydroxyapatite porous composites for bone-tissue engineering. I. Preparation and morphologyJ Biomed Mater Res19994444645510397949
- HsuYYGresserJDTrantoloDJLyonsCMGangadharamPRWiseDLEffect of polymer foam morphology and density on kinetics of in vitro controlled release of isoniazid from compressed foam matricesJ Biomed Mater Res1997351071169104703
- KhorasaniMTMirzadehHBHK cells behaviour on laser treated polydimethylsiloxane surfaceColloids Surf B Biointerfaces200435677115261058
- KarbasiSMirzadehHOrangFUrbanJPGA comparison between cell viability of chondrocytes on a biodegradable polyester urethane scaffold and alginate beads in different oxygen tension and pHIran Polym J200514823830
- YangLKoromSWederWTissue-engineered cartilage generated for human trachea using Degrapol scaffoldEur J Cardiothorac Surg20032420120712895608
- BonakdarSHojjati EmamiSShokrgozarMAPreparation and characterization of polyvinyl alcohol hydrogels crosslinked by biodegradable polyurethane for tissue engineering of cartilageMater Sci Eng C Mater Biol Appl201030636643
- KurimotoYTakedaMKoizumiAYamauchiSDoiSTamuraYMechanical properties of polyurethane films prepared from liquefied wood with polymeric MDIBioresour Technol200074151157