Abstract
Background
Cationic solid lipid nanoparticles (SLNs) have been given considerable attention for therapeutic nucleic acid delivery owing to their advantages over viral and other nanoparticle delivery systems. However, poor delivery efficiency and complex formulations hinder the clinical translation of SLNs.
Aim
The aim of this study was to formulate and characterize SLNs incorporating the cholesterol derivative cholesteryl oleate to produce SLN–nucleic acid complexes with reduced cytotoxicity and more efficient cellular uptake.
Methods
Five cholesteryl oleate-containing formulations were prepared. Laser diffraction and laser Doppler microelectrophoresis were used to evaluate particle size and zeta potential, respectively. Nanoparticle morphology was analyzed using electron microscopy. Cytotoxicity and cellular uptake of lipoplexes were evaluated using flow cytometry and fluorescence microscopy. The gene inhibition capacity of the lipoplexes was assessed using siRNAs to block constitutive luciferase expression.
Results
We obtained nanoparticles with a mean diameter of approximately 150–200 nm in size and zeta potential values of 25–40 mV. SLN formulations with intermediate concentrations of cholesteryl oleate exhibited good stability and spherical structures with no aggregation. No cell toxicity of any reference SLN was observed. Finally, cellular uptake experiments with DNA-and RNA-SLNs were performed to select one reference with superior transient transfection efficiency that significantly decreased gene activity upon siRNA complexation.
Conclusion
The results indicate that cholesteryl oleate-loaded SLNs are a safe and effective platform for nonviral nucleic acid delivery.
Introduction
The development of efficient delivery systems is a critical factor for successful gene therapy.Citation1 Numerous studies have described the use of vectors, such as retroviruses and adenoviruses, for delivering and expressing genes.Citation2–Citation4 While this approach often results in high expression efficiencies, it has several disadvantages, including marked immunogenicity, insertional mutagenesis, DNA package size limit, nonspecific effects, and potential hazards to laboratory personnel (comprehensive reviews are given in the studies by Collins and Thrasher,Citation5 van der Loo and Wright,Citation6 and Chira et alCitation7). Alternatively, mechanical transfection methods, such as direct microinjection and the “gene gun,” or physical transfection methods, such as electroporation, sonoporation, or laser irradiation, represent direct methods for delivering genetic material into cells.Citation8 Some of these methods have demonstrated their potential to directly transfer nucleic acids into cells and achieve good transgene expression. However, the instability of the nucleic acid and its rapid degradation, together with the associated toxicity, are still present.Citation8 In recent decades, nanoparticle-mediated therapeutic nucleic acid delivery has been gaining considerable attention. Compared with viral systems, nanoparticles are less immunogenic and oncogenic and have no potential for virus recombination, all of which translates to improved safety.Citation9 Nanoparticles are relatively easy to prepare and can incorporate nucleic acids with little toxicity.Citation10 However, the transfection efficiency is low and a major concern in nanoparticle-mediated targeted therapy.Citation11 While considerable effort is needed to study the biological interactions of nanoparticles with cells and their constituent proteins,Citation12 it has been widely observed that transfection efficiency is dependent on the specific properties of the nanoparticle, ie, its formulation.Citation13 Nanoparticle diameter, shape, surface charge, and components influence cellular uptake and toxicity.Citation14–Citation17 Hence, the research and development of novel nanoparticle formulations able to enter cells with high efficiency have been a priority for the clinical success of nanoparticle delivery systems.Citation11,Citation18
Depending on the manufacturing process and the physicochemical properties, nanoparticles fall into different categories, ie, inorganic and organic nanoparticles. Among the first, a good example is gold nanoparticles, which can be used as cancer immunotherapy carriers or for diagnostic applications because they can be functionalized to detect low levels of specific targets.Citation19–Citation22 Organic nanoparticles have shown tremendous potential for broad clinical applications and gene therapy applications.Citation23–Citation25 As an example, several formulations of polymeric nanoparticles containing poly(lactic-co-glycolic acid) (PLGA), cyclodextrin, and chitosan have been developed for cancer therapy,Citation26,Citation27 and pH-sensitive polymers, which exhibit improved drug delivery to tumors, have shown increased antitumor activity with decreased side effects.Citation28 The fact that many nanoparticle-based approaches have been approved for clinical use serves as a proof of concept.Citation29,Citation30 However, manufacturing processes, costs, toxicity, and transfection efficiency continue to hinder the widespread use of nanotechnology for clinical purposes in humans.Citation1
Cationic solid lipid nanoparticles (SLNs) represent one of the most promising nanoparticle-based methods for gene therapy.Citation31–Citation35 SLNs were developed to overcome the limitations of polymeric nanoparticles and other lipid-based nanoparticles.Citation36 They are based on the use of biocompatible lipids to produce a surface charge, which allows DNA and RNA binding and facilitates good biocompatibility and low cytotoxicity.Citation10,Citation37,Citation38 SLNs are capable of transfecting nucleic acids into cells in vitro and in vivo,Citation10,Citation35 thus supporting their research and development for potential effective gene therapy strategies. SLNs can also be formulated for drug delivery and targeting through antibody-mediated, cationic lipid, and pH-sensitive lipid attachments.Citation33 SLNs can be obtained using several methods with the advantage of avoiding organic solvent incorporation.Citation39,Citation40 Although SLNs have a remarkably wide range of properties and applications, they present some limitations and difficulties that need to be overcome before their translation into the clinic. Poor delivery efficiency, uncontrollable nanoparticle transport inside the body, and problems related to realizing some manufacturing processes at a large scale to meet Good Manufacturing Practice (GMP) standards are limitations that explain the small number of these products on the market.Citation41 Enhanced SLN formulations need to be engineered to overcome these obstacles and produce effective nanoparticle systems for the successful intracellular delivery of nucleic acids and the improvement in nanomedicine development and translation.
The use of the cholesterol derivative cholesteryl oleate in SLN formulations has not been reported. In this study, we proposed the use of cholesteryl oleate to obtain an optimized formula that maintains the nanoparticle structure, morphology, and nucleic acid binding efficiency while improving both cytotoxicity and transfection to induce potent biological activity. We used a simple fabrication method with inexpensive reagents, thus incentivizing the eventual scaled-up production of SLNs.
Materials and methods
SLN production
The following materials were used to synthesize the nanoparticles: poloxamer 188 (Sigma-Aldrich Co., St Louis, MO, USA), octadecylamine (Acros Organics, Geel, Belgium), stearic acid (EMD Millipore, Billerica, MA, USA), cholesteryl oleate (Alfa Aesar, Karlsruhe, Germany), and ultrapure water (EMD Millipore). The SLNs were produced using the hot microemulsification method, as previously described.Citation42 Briefly, all components were melted, and the matrix lipid was poured with stirring onto the cationic lipid, water, and surfactant solution to form a hot emulsion. The emulsion was dispersed into cooled water at 2°C–3°C (ratio 1:5) under stirring to form the SLNs. The final microemulsion was centrifuged at 15,000 rpm (approximately 19,000× g) and filtered. These nanoparticles were lyophilized to preserve their properties and to improve the stability. The amount of the matricial lipid (cholesteryl oleate + stearic acid) varied depending on the formulation (). All assays except for the electronic microscopy studies were performed with lyophilized SLNs (L-3 Telstar; Telstar, Terrassa, Spain) using trehalose (5% w/v) as a cryoprotectant.
Table 1 Composition of the engineered nanoparticles
Plasmid constructs and siRNAs
The major histocompatibility complex (MHC) reporter luciferase plasmid has a minimal fos promoter element and three copies of the MHC class I κB element and has been previously described.Citation43 The sequence of the Cy3-labeled siRNA was as follows: 5′-CUACAACAGCCACAACGE-3′ (siEGFP).Citation44 The sequence of the siRNA targeting luciferase (siLUC) was as follows: 5′-UUGUAUUCAGCCCAUAGC-3′.Citation45
Determination of SLN particle size
SLN suspensions were used for particle size determination by laser diffraction according to the Mie theory on a Mastersizer 2000 (Malvern Instruments, Malvern, UK). All samples were measured in triplicate using a sufficient amount of sample to obtain an appropriate obscuration percentage. A Fourier transform was applied to the measured diffracted angles to obtain the particle size data using specialized software, and the mean value was calculated in nanometers.
Determination of SLN surface charge (zeta potential)
A Zetasizer Nano Z (Malvern Instruments) was used to determine the surface charge of the SLNs by laser Doppler microelectrophoresis. Samples were stabilized at 25°C prior to measurement. The electrophoretic mobility between electrodes connected to the cell containing the sample was converted to zeta potential values using specific software. Results are expressed in mV.
Morphological analysis of SLNs
The surface and content homogeneity of the SLNs were analyzed by transmission electron microscopy (TEM) using a Spirit 120 kV microscope (FEI Company, Hillsboro, OR, USA).
Cell culture and transfection assays
HEK293T cells were obtained from the American Type Culture Collection (ATCC, Manassas, VA, USA) and grown and maintained as previously described.Citation46 For the viability assays, HEK293T cells were grown in 35 mm plates (Fisher Scientific, Madrid, Spain) to approximately 60%–70% confluence. Because the concentration of octadecylamine varied according to the performance of the manufacturing process, we measured its concentration using a Varian 212 liquid chromatography mass spectrometry (LC-MS) system, and the test volumes were selected based on the concentration of octadecylamine present in the samples to ensure that the amount of octadecylamine and the octadecylamine:cholesteryl oleate ratio remained constant. Accordingly, SLNs were added to the cell cultures using 23.4 µL of reference Citation12, 14.2 µL of reference Citation13, 5 µL of reference Citation14, 4.25 µL of reference Citation15, and 11.6 µL of reference Citation16 (). After 24 and 48 h, cells were harvested and processed for cytotoxicity using flow cytometry analysis. For all the biological assays implicating nucleic acids, the complexes were prepared by mixing SLNs with the appropriate amount of plasmid DNA (pDNA) or siRNA as given in the following sections. The mixture was kept at room temperature for 40–45 min to allow the complexes to form before transfection. For the luciferase assays, HEK293T cells were grown in 35 mm plates to approximately 60%–70% confluence, and then the medium was changed to a medium without serum or antibiotics. Lipoplexes were prepared by mixing 1,000 ng of the MHC reporter luciferase plasmid, 100 ng of Renilla plasmid, and the amounts of SLNs derived from the viability assay as mentioned earlier. The mixtures were added to the cells, and after 4–6 h, 200 µL of serum was added to each well. The cells were harvested approximately 48 h after transfection and processed for luciferase activity using the dual-luciferase reporter assay (Promega Corporation, Fitchburg, WI, USA) protocol following the manufacturer’s instructions. For the transfection of Cy3-labeled siRNA, HEK293T cells were grown in 35 mm plates (Falcon) to approximately 60%–70% confluence, and the medium was changed to a medium without serum or antibiotics. Lipoplexes were prepared by mixing 60 or 120 nM of Cy3-labeled siRNA and 13.5 µL of reference Citation14 SLNs. As a transfection control, 60 nM of Cy3-labeled siRNA was transfected into cells using Lipofectamine 2000 according to the manufacturer’s protocols. Then, the cells were transfected with the lipoplexes. Cells were harvested at different times after transfection and processed for flow cytometry and confocal microscopy analysis. For the transfection of siLUC, we used the TZM-bl cell line. TZM-bl is a HeLa cell line that contains integrated copies of the luciferase and B-galactosidase genes under the control of the HIV-1 promoter (NIH AIDS Reagent Program, Germantown, MD, USA).Citation47 Cells were grown in 35 mm plates to approximately 60%–70% confluence, and the medium was changed to a medium without serum or antibiotics. Lipoplexes were prepared by mixing 120 nM of siLUC and different amounts of reference Citation14 SLNs. Cells were transfected with the lipoplex mixtures, and after 4–6 h, 200 µL of serum was added to each well.
Cell proliferation
Cell proliferation was detected using the MTT reagent (Hoffman-La Roche Ltd., Basel, Switzerland). Briefly, 10 µL of MTT was added to the plate and incubated for 4 h at 37°C. The purple precipitate of the cells was treated with solubilization buffer, as indicated by the manufacturer, to dissolve the crystals. The absorbance at 570 nm was measured with an enzyme-linked immunosorbent assay (ELISA) reader (Molecular Devices LLC, Sunnyvale, CA, USA) at different time points after treatment.
Flow cytometry
Cytometry was performed on a FACSCalibur (BD Biosciences, San Jose, CA, USA), and the results were analyzed using FlowJo software. The cutoff values were established using unstained HEK293T cells to subtract the autofluorescence signal of the cells. At this setting, only 0.5%–1% of the cells were over the 100 level for the Cy3 fluorescence channel.
Confocal microscopy
Confocal microscopy studies were performed using a Leica SP5 spectral confocal laser scanning microscope (Leica Microsystems, Wetzlar, Germany). Images were analyzed and digitally processed for presentation using LAS AF v2.3.6 software (Leica Microsystems) and Adobe Photoshop CS3 extended v10.0 software, respectively.
Statistical analyses
Statistical analyses were performed using Graph Pad Prism 5.0 (GraphPad Software, Inc., La Jolla, CA, USA) with one-way analysis of variance (ANOVA) followed by Tukey’s posttest analysis to describe significant differences between groups. The P-values are represented by asterisks (**P = 0.001–0.01 and ***P < 0.001). The absence of an asterisk indicates that the change relative to the control is not statistically significant.
Results
Preparation and characterization of SLNs
The particle size results from the initial characterization of the different SLN references 12–16 are shown in . We obtained an important population of particles approximately 150–200 nm in size, confirming the presence of nanoparticles. References 12–14 contained homogeneous material with almost no aggregates present (). We observed an increased amount of aggregation in reference Citation15, but nanoparticles were also synthesized (). Reference Citation16, which was synthesized with 100% cholesteryl oleate, contained numerous aggregates (). These results showed that references 12–14 were the best suitable to form lipoplexes for transfection purposes. Concerning zeta potential, there were no differences among the different formulations (). All the references had zeta potential values from 25 to 40 mV, which indicated a potentially good capacity for nucleic acid binding.
Table 2 SLN zeta potentials
Figure 1 Particle size distribution of reference Citation12 (A), reference Citation13 (B), reference Citation14 (C), reference Citation15 (D), and reference Citation16 (E) measured by laser diffraction.
Notes: Composition of the engineered nanoparticles – reference Citation12: 400 mg stearic acid, 100 mg cholesteryl oleate, 600 mg octadecylamine, 100 mg poloxamer 188; reference Citation13: 300 mg stearic acid, 200 mg cholesteryl oleate, 600 mg octadecylamine, 100 mg poloxamer 188; reference Citation14: 200 mg stearic acid, 300 mg cholesteryl oleate, 600 mg octadecylamine, 100 mg poloxamer 188; reference Citation15: 100 mg stearic acid, 400 mg cholesteryl oleate, 600 mg octadecylamine, 100 mg poloxamer 188; reference Citation16: 0 mg stearic acid, 500 mg cholesteryl oleate, 600 mg octadecylamine, 100 mg poloxamer 188.
Abbreviation: d, diameter.
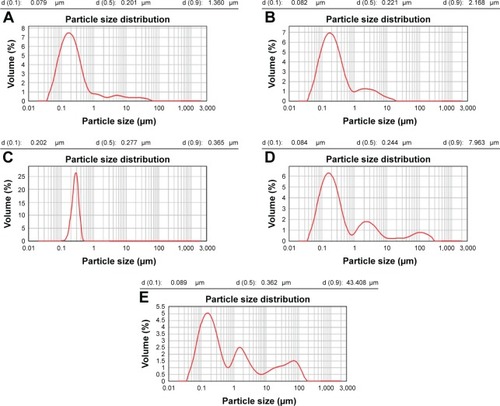
Images of the SLNs were acquired by TEM as shown in . We observed the presence of nanostructures in all the formulations. However, the homogeneity and quality of these particles were substantially different in each formulation. For references 12–14, the images showed a high proportion of nanoparticles without any amorphous structures. In contrast, images of references 15 and 16 showed less spherical nanoparticles. In reference Citation16, we also observed many aggregates using a large field of view (data not shown). For reference Citation16, it was difficult to find images containing nanoparticles, further suggesting that the presence of 100% cholesteryl oleate in the formula yields nanoparticles that become unstable. These results are consistent with the laser diffraction data and confirm the suitability of references 12–14 for further investigation.
Figure 2 TEM images of reference Citation12 (A), reference Citation13 (B), reference Citation14 (C), reference Citation15 (D), and reference Citation16 (E).
Notes: Scale bars are in µm. Composition of the engineered nanoparticles – reference Citation12: 400 mg stearic acid, 100 mg cholesteryl oleate, 600 mg octadecylamine, 100 mg poloxamer 188; reference Citation13: 300 mg stearic acid, 200 mg cholesteryl oleate, 600 mg octadecylamine, 100 mg poloxamer 188; reference Citation14: 200 mg stearic acid, 300 mg cholesteryl oleate, 600 mg octadecylamine, 100 mg poloxamer 188; reference Citation15: 100 mg stearic acid, 400 mg cholesteryl oleate, 600 mg octadecylamine, 100 mg poloxamer 188; reference Citation16: 0 mg stearic acid, 500 mg cholesteryl oleate, 600 mg octadecylamine, 100 mg poloxamer 188.
Abbreviation: TEM, transmission electron microscopy.
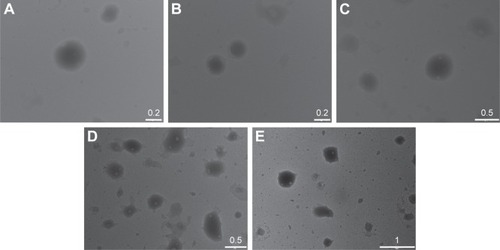
Cytotoxicity
An early and crucial stage in determining the efficacy of nanoparticles is toxicity testing. To determine the effect of the SLNs on cellular viability, a fixed quantity of nanoparticles from each reference was incubated with HEK293T cells for 48 h. We assessed cell viability by counting the number of viable cells using flow cytometry analysis. Variability in the nanoparticle concentration among the samples was minimized by quantifying octadecylamine via LC-MS. No toxic effects of any SLN reference were observed (). Moreover, the viability of SLN-containing cells was similar to that of untreated cells after the incubation time (). These results show that the modified SLNs are not harmful to human cells cultured in vitro. The cytotoxicity against HEK293T cells was also studied using the MTT colorimetric cell proliferation assay. Time-dependent cytotoxic effects of SLNs from reference Citation14 (selected based on their physicochemical characteristics and good performance as given in the following sections) were observed as shown in . While initial cytotoxicity was observed at 24 h after treatment, the cells recovered quickly and showed almost normal viability at later treatment time points ().
Figure 3 (A) Cell viability assays in HEK293T cells with references 12–16 at 24 and 48 h. Data are from two independent experiments (mean ± SEM). (B) MTT assay of HEK293T cells at different time points following treatment with reference Citation14. Data are from one representative experiment performed in quadruplicate.
Notes: Composition of the engineered nanoparticles – reference Citation12: 400 mg stearic acid, 100 mg cholesteryl oleate, 600 mg octadecylamine, 100 mg poloxamer 188; reference Citation13: 300 mg stearic acid, 200 mg cholesteryl oleate, 600 mg octadecylamine, 100 mg poloxamer 188; reference Citation14: 200 mg stearic acid, 300 mg cholesteryl oleate, 600 mg octadecylamine, 100 mg poloxamer 188; reference Citation15: 100 mg stearic acid, 400 mg cholesteryl oleate, 600 mg octadecylamine, 100 mg poloxamer 188; reference Citation16: 0 mg stearic acid, 500 mg cholesteryl oleate, 600 mg octadecylamine, 100 mg poloxamer 188.
Abbreviations: Ctrl, control; H2O2, hydrogen peroxide; SEM, standard error of the mean.
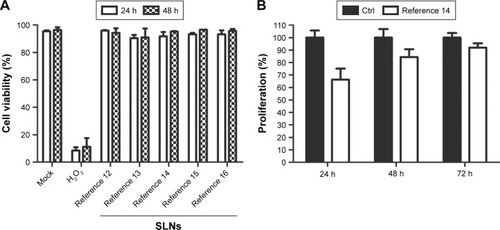
Cellular uptake and biological activity
The cellular uptake of nanoparticles and their capability to generate a biological response can be tested by the luciferase reporter assay, where pDNA driving the expression of the luciferase gene is transfected into cells. Using this assay, we tested the cellular uptake and bioactivity of two different luciferase reporter plasmids (MHC and Renilla) using references 12–16 () in the same quantities employed in the viability assays. While we observed a dose–response effect in the luciferase activity upon transfection with SLNs from references 12 to 14, the enzymatic activity decreased upon transfection with SLNs from references 15 and 16. These results correlate with the physicochemical parameters of the SLNs and suggest that aggregation and poor stability are detrimental to transfection efficiency and bioactivity. Reference Citation14 resulted in potent luciferase activity (), which is consistent with the previously determined size and morphology data of this formulation (our results) and with the binding capacity of these nanoparticles.Citation10,Citation42 We concluded that our cholesteryl oleate-based SLNs can be internalized in cells and generate a biological response. Therefore, these SLNs provided protection against nuclease activity, enabling cellular uptake, intracellular nucleic acid release and RNA transcription in the nucleus, transport to the cytoplasm, and translation into protein, enabling bioactivity. Reference Citation14 showed good physicochemical parameters and superior transfection efficiency and was therefore suitable for further experimentation.
Figure 4 MHC (1000 ng) (A) and Renilla (100 ng) (B) plasmids were transfected into HEK293T via SLNs (references 12–16).
Notes: Data are from three independent experiments performed in triplicate (mean ± SEM). ***P < 0.001. MHC, plasmid containing a minimal fos promoter and three copies of the MHC class I κB element. Composition of the engineered nanoparticles – reference Citation12: 400 mg stearic acid, 100 mg cholesteryl oleate, 600 mg octadecylamine, 100 mg poloxamer 188; reference Citation13: 300 mg stearic acid, 200 mg cholesteryl oleate, 600 mg octadecylamine, 100 mg poloxamer 188; reference Citation14: 200 mg stearic acid, 300 mg cholesteryl oleate, 600 mg octadecylamine, 100 mg poloxamer 188; reference Citation15: 100 mg stearic acid, 400 mg cholesteryl oleate, 600 mg octadecylamine, 100 mg poloxamer 188; reference Citation16: 0 mg stearic acid, 500 mg cholesteryl oleate, 600 mg octadecylamine, 100 mg poloxamer 188.
Abbreviations: MHC, major histocompatibility complex; SEM, standard error of the mean; SLNs, solid lipid nanoparticles.
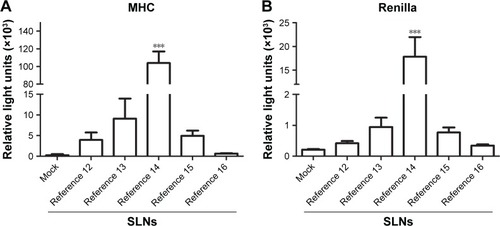
A more relevant model for RNA interference (RNAi) applications is the siRNA-mediated targeting of endogenous gene products rather than the transient transfection of plasmid-based genes. Therefore, we generated a synthetic siRNA against the luciferase gene to be used in subsequent experiments. First, we used flow cytometry to evaluate the cellular uptake of SLN-Cy3-labeled siRNA complexes using reference Citation14 in HEK293T cells. SLN complexation was performed with 60 and 120 nM Cy3-labeled siRNA. After the quantification analysis, the transfection efficiency of the SLN-Cy3-labeled siRNA complexes was approximately 40%–45% (). However, the SLNs elicited increased signal intensity compared with Lipofectamine 2000 (). Interestingly, we observed an increase in signal intensity when using larger quantities of SLN-Cy3-labeled siRNA complexes (). Given that both siRNA concentrations exhibited similar transfection efficiency, these data suggest that more fluorescent siRNA is introduced into each individual cell, which may give rise to an increased biological response.
Figure 5 The cell transfection percentage (A) and the mean signal intensity (B) of complexes formed with 60 and 120 nM siEGFPCy3 with reference Citation14 measured by flow cytometry.
Notes: Data are from two independent experiments (mean ± SEM). *P = 0.01–0.05, **P = 0.001–0.01, and ***P < 0.001. siEGFPCy3, small interfering RNA against the enhanced green fluorescent protein labeled with cyanine dye 3. Composition of reference Citation14: 200 mg stearic acid, 300 mg cholesteryl oleate, 600 mg octadecylamine, 100 mg poloxamer 188.
Abbreviations: Ctrl, control; SEM, standard error of the mean.
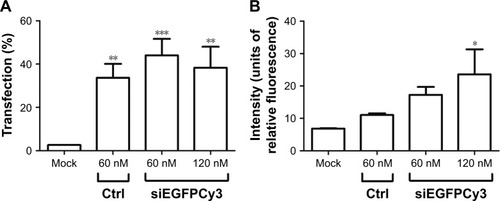
The transfection efficiency data were further supported by the confocal microscopy results. We observed a lack of fluorescence when the Cy3-labeled siRNA alone was added to the cells (). Many large fluorescent dots were observed in the cytoplasm and in the nucleus at 1 h post-transfection of the Cy3-labeled siRNA using Lipofectamine 2000 (). Importantly, the addition of the SLN-Cy3-labeled siRNA complexes resulted in similar fluorescence signals (), reflecting the efficient cell binding and intracellular uptake of the lipoplexes. While strong fluorescence signals were still observed at 2 h post-transfection, weak fluorescence was detected at 6 h post-transfection, as assessed by confocal microscopy () or flow cytometry (data not shown), thus revealing the rapid intracellular uptake of these lipoplexes. Grayscale images () showed multiple arrays of fluorescence signals widely spread throughout the cells, which may explain the loss of signal observed with image color information.
Figure 6 Confocal microscopy images of HEK293T cells transfected with Cy3-labeled siRNA alone (A), together with Lipofectamine (B), or together with reference Citation14 (C) at 1 h post-transfection.
Notes: DAPI (4′,6-damidino-2-phenylindole) labeling was used to stain the chromatin (blue). Scale bars, 25 µm. Composition of reference Citation14: 200 mg stearic acid, 300 mg cholesteryl oleate, 600 mg octadecylamine, 100 mg poloxamer 188.
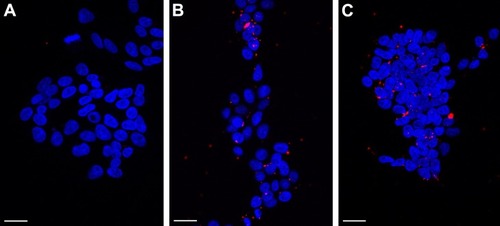
Figure 7 HEK293T cells were transfected with Cy3-labeled siRNA together with reference Citation14 and were analyzed at 1, 2, and 6 h after transfection using confocal microscopy (A and B).
Notes: Grayscale images are shown in part B. Scale bars, 25 μm. Composition of reference Citation14: 200 mg stearic acid, 300 mg cholesteryl oleate, 600 mg octadecylamine, 100 mg poloxamer 188.
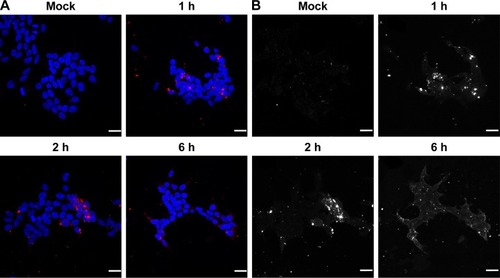
To confirm that the SLNs could deliver and release siRNA molecules into cells to result in bioactivity, we performed transfection experiments using TZM-bl cells, which carry integrated copies of the HIV-1 long terminal repeat driving expression of the luciferase gene. Targeting luciferase expression with SLN-siLUC complexes using different quantities of reference Citation14 resulted in an approximately 35% reduction in luciferase activity after 48 h compared with the control (). This downregulation of endogenous luciferase expression validated the use of SLNs incorporating cholesteryl oleate in their formulation as promising tools for gene targeting.
Figure 8 Activity of siLUC upon transfection via reference Citation14 SLNs in TZM-bl cells.
Notes: Data are from five experiments performed in duplicate (mean ± SEM). **P = 0.001–0.01 and ***P < 0.001. Composition of reference Citation14: 200 mg stearic acid, 300 mg cholesteryl oleate, 600 mg octadecylamine, 100 mg poloxamer 188.
Abbreviations: SEM, standard error of the mean; siLUC, siRNA targeting luciferase; SLNs, solid lipid nanoparticles.
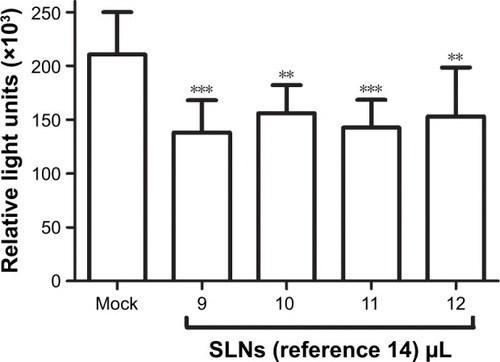
Discussion
Identification of new formulations and cationic compounds for therapeutic use is of fundamental importance for achieving therapeutic nucleic acid transfer. This objective must be attained with consideration for the requirements of cost-optimized large-scale production. The purpose of this study was to formulate and characterize SLNs incorporating the cholesterol derivative cholesteryl oleate to achieve SLN–nucleic acid complexes with more efficient cellular uptake and reduced cytotoxicity. The steroid lipid cholesterol is an essential component of animal cell membranes and has multiple functions. We reasoned that incorporating cholesterol or cholesterol derivatives in the lipoplex formulation might increase the transfection efficiency and biocompatibility of the resulting SLNs. Previous studies have shown that lipid mixtures with cholesterol or cholesterol derivatives, which were generally synthesized using complicated protocols, exhibited enhanced transfection.Citation48–Citation51 Cholesterol may improve transfection efficiency by promoting fusion of the lipoplex with the cell membrane.Citation52 We decided to test cholesteryl oleate because of its low melting point (44°C–47°C versus 148°C for cholesterol), which was compatible with our protocols.Citation42 In this study, we have shown that cholesteryl oleate can be conveniently formulated in SLNs through a simple and uncomplicated protocol. The resulting vectors showed good performance in delivering DNA or siRNA into cells without cytotoxicity and with good transfection efficiency.
We compared in detail the properties of a series of SLNs with increasing quantities of cholesteryl oleate in its formulation. While all SLNs formed suspensions in aqueous media, the stability of the nanoparticles was decreased with high cholesteryl oleate concentrations (references 15 and 16). Accordingly, electron microscopy studies revealed nearly spherical structures in those SLN formulations with low and intermediate concentrations of cholesteryl oleate and less spherical structures with abundant aggregation in those formulations with higher concentrations of cholesteryl oleate. While the toxicity assessment results of the formulations containing any cholesteryl oleate concentration were similar to that observed for the control, the transfection efficiency of each compound differed according to the formulation. In the transient transfection experiments using firefly and Renilla luciferase expression vectors, reference Citation14 displayed robust, dose–response luciferase activation much higher than that observed for the other references. Therefore, this reference was selected for further studies using siRNA. Cytometry and confocal microscopy characterizations showed the ability of reference Citation14 to deliver siRNA molecules into cells. We observed massive and rapid uptake, as indicated by many large siRNA-labeled fluorescent dots throughout the cytoplasm at 1 h post-transfection, with a loss of signal at longer times post-transfection. Semiquantitative cytometry analysis showed approximately 45% cell incorporation, which is a remarkable transfection efficiency for a non-functionalized nanoparticle-based delivery method.Citation53 In addition to enhanced cellular uptake levels, siRNA delivery requires stabilization and protection against degradation by nucleases, which can impair the biological response. Targeting a chromosome-integrated luciferase gene, we observed an ~35% reduction in enzymatic activity, which demonstrates that upon SLN complexation, siRNA is protected against degradation, internalized by cells, and released intracellularly, ultimately leading to bioactivity. In this study, we used HEK293T and HeLa cells to establish experimental conditions for nucleic acid delivery into cells using SLNs formulated with cholesteryl oleate. Future experiments will test our formulation in hard-to-transfect primary and cell lines to deliver nucleic acids to appropriate targets in order to interfere with relevant gene expression programs.
Conclusion
Nanoparticles can facilitate the intracellular delivery of siRNA, and many studies have shown that siRNA can have a significant therapeutic effect on disease, especially tumors.Citation54 However, the use of these nanoparticle carriers still has disadvantages in terms of transfection efficiency, immunogenicity, and toxicity, all of which limit their therapeutic applications. The experiments reported in this study demonstrate that the use of cholesteryl oleate in SLNs provides an avenue for the development of highly efficient and nontoxic delivery carriers for therapeutic applications.
Acknowledgments
We are grateful to many colleagues for their helpful suggestions, critical discussions, and comments. We thank José Alcamí and Mayte Coiras (National Microbiology Center, Majadahonda, Madrid, Spain) for the TZM-bl cells. This work was supported by grants from the Spanish Ministry of Economy and Competitiveness (grant number BFU2014-54660-R) and the Andalusian Government (Excellence Project BIO-2515/2012) to CS and from the Spanish Ministry of Economy and Competitiveness (grant number BFU2016-79699-P) to CHM. Support from the European Region Development Fund (ERDF [FEDER]) is also acknowledged. MSP was supported by a fellowship from the Spanish Ministry of Education (FPU Program [Training Program for Academic Staff]).
Disclosure
The authors report no conflicts of interest in this work.
References
- WilhelmSTavaresAJDaiQAnalysis of nanoparticle delivery to tumoursNat Rev Mater20161112
- Hacein-Bey-AbinaSLe DeistFCarlierFSustained correction of X-linked severe combined immunodeficiency by ex vivo gene therapyN Engl J Med2002346161185119311961146
- WestphalMYla-HerttualaSMartinJASPECT Study GroupAdenovirus-mediated gene therapy with sitimagene ceradenovec followed by intravenous ganciclovir for patients with operable high-grade glioma (ASPECT): a randomised, open-label, phase 3 trialLancet Oncol201314982383323850491
- CicaleseMPFerruaFCastagnaroLUpdate on the safety and efficacy of retroviral gene therapy for immunodeficiency due to adenosine deaminase deficiencyBlood20161281455427129325
- CollinsMThrasherAGene therapy: progress and predictionsProc Biol Sci201528218212014300326702034
- van der LooJCWrightJFProgress and challenges in viral vector manufacturingHum Mol Genet201625R1R42R5226519140
- ChiraSJacksonCSOpreaIProgresses towards safe and efficient gene therapy vectorsOncotarget2015631306753070326362400
- Mehier-HumbertSGuyRHPhysical methods for gene transfer: improving the kinetics of gene delivery into cellsAdv Drug Deliv Rev200557573375315757758
- YinHKanastyRLEltoukhyAAVegasAJDorkinJRAndersonDGNon-viral vectors for gene-based therapyNat Rev Genet201415854155525022906
- FabregasAPrieto-SanchezSSune-PouMImproved formulation of cationic solid lipid nanoparticles displays cellular uptake and biological activity of nucleic acidsInt J Pharm20175161–2394427840160
- Al-DosariMSGaoXNonviral gene delivery: principle, limitations, and recent progressAAPS J200911467168119834816
- DengJGaoCRecent advances in interactions of designed nanoparticles and cells with respect to cellular uptake, intracellular fate, degradation and cytotoxicityNanotechnology2016274141200227609340
- BashaGNovobrantsevaTIRosinNInfluence of cationic lipid composition on gene silencing properties of lipid nanoparticle formulations of siRNA in antigen-presenting cellsMol Ther201119122186220021971424
- ChithraniBDChanWCElucidating the mechanism of cellular uptake and removal of protein-coated gold nanoparticles of different sizes and shapesNano Lett2007761542155017465586
- GrattonSERoppPAPohlhausPDThe effect of particle design on cellular internalization pathwaysProc Natl Acad Sci U S A200810533116131161818697944
- MaranoFHussainSRodrigues-LimaFBaeza-SquibanABolandSNanoparticles: molecular targets and cell signallingArch Toxicol201185773374120502881
- RauchJKolchWLaurentSMahmoudiMBig signals from small particles: regulation of cell signaling pathways by nanoparticlesChem Rev201311353391340623428231
- WangYHuangLComposite nanoparticles for gene deliveryAdv Genet20148811113725409605
- AnselmoACMitragotriSA review of clinical translation of inorganic nanoparticlesAAPS J20151751041105425956384
- AlmeidaJPFigueroaERDrezekRAGold nanoparticle mediated cancer immunotherapyNanomedicine201410350351424103304
- MieszawskaAJMulderWJFayadZACormodeDPMultifunctional gold nanoparticles for diagnosis and therapy of diseaseMol Pharm201310383184723360440
- HuangHCBaruaSSharmaGDeySKRegeKInorganic nanoparticles for cancer imaging and therapyJ Control Release2011155334435721723891
- GozuacikDYagci-AcarHFAkkocYKosarADogan-EkiciAIEkiciSAnticancer use of nanoparticles as nucleic acid carriersJ Biomed Nanotechnol20141091751178325992440
- KanastyRDorkinJRVegasAAndersonDDelivery materials for siRNA therapeuticsNat Mater2013121196797724150415
- WhiteheadKALangerRAndersonDGKnocking down barriers: advances in siRNA deliveryNat Rev Drug Discov20098212913819180106
- KuSHKimKChoiKKimSHKwonICTumor-targeting multifunctional nanoparticles for siRNA delivery: recent advances in cancer therapyAdv Healthc Mater2014381182119324577795
- WangXYangCZhangYZhenXWuWJiangXDelivery of platinum(IV) drug to subcutaneous tumor and lung metastasis using bradykinin-potentiating peptide-decorated chitosan nanoparticlesBiomaterials201435246439645324811257
- HeHChenSZhouJCyclodextrin-derived pH-responsive nanoparticles for delivery of paclitaxelBiomaterials201334215344535823591391
- MinYCasterJMEblanMJWangAZClinical translation of nanomedicineChem Rev201511519111471119026088284
- BoboDRobinsonKJIslamJThurechtKJCorrieSRNanoparticle-based medicines: a review of FDA-approved materials and clinical trials to datePharm Res201633102373238727299311
- CarrilloCSanchez-HernandezNGarcia-MontoyaEDNA delivery via cationic solid lipid nanoparticles (SLNs)Eur J Pharm Sci201349215716523454134
- MoritzMGeszke-MoritzMRecent developments in the application of polymeric nanoparticles as drug carriersAdv Clin Exp Med201524574975826768624
- RostamiEKashanianSAzandaryaniAHFaramarziHDolatabadiJEOmidfarKDrug targeting using solid lipid nanoparticlesChem Phys Lipids2014181566124717692
- YuYHKimEParkDECationic solid lipid nanoparticles for co-delivery of paclitaxel and siRNAEur J Pharm Biopharm201280226827322108492
- ApaolazaPSDel Pozo-RodriguezASolinisMAStructural recovery of the retina in a retinoschisin-deficient mouse after gene replacement therapy by solid lipid nanoparticlesBiomaterials201690404926986855
- MadanJRKhudePADuaKDevelopment and evaluation of solid lipid nanoparticles of mometasone furoate for topical deliveryInt J Pharm Investig2014426064
- Ezzati Nazhad DolatabadiJHamishehkarHEskandaniMValizadehHFormulation, characterization and cytotoxicity studies of alendronate sodium-loaded solid lipid nanoparticlesColloids Surf B Biointerfaces2014117212824607519
- DoktorovovaSKovacevicABGarciaMLSoutoEBPreclinical safety of solid lipid nanoparticles and nanostructured lipid carriers: current evidence from in vitro and in vivo evaluationEur J Pharma Biopharm2016108235252
- Geszke-MoritzMMoritzMSolid lipid nanoparticles as attractive drug vehicles: composition, properties and therapeutic strategiesMater Sci Eng C Mater Biol Appl20166898299427524099
- MullerRHMaderKGohlaSSolid lipid nanoparticles (SLN) for controlled drug delivery – a review of the state of the artEur J Pharm Biopharm200050116117710840199
- Ezzati Nazhad DolatabadiJValizadehHHamishehkarHSolid lipid nanoparticles as efficient drug and gene delivery systems: recent breakthroughsAdv Pharm Bull20155215115926236652
- FabregasASanchez-HernandezNTicoJRA new optimized formulation of cationic solid lipid nanoparticles intended for gene delivery: development, characterization and DNA binding efficiency of TCERG1 expression plasmidInt J Pharm20144731–227027924999055
- Sánchez-ÁlvarezMMontesMSánchez-HernándezNHernández-MunainCSuñéCDifferential effects of sumoylation on transcription and alternative splicing by transcription elongation regulator 1 (TCERG1)J Biol Chem201028520152201523320215116
- BecerraSMontesMHernández-MunainCSuñéCPrp40 pre-mRNA processing factor 40 homolog B (PRPF40B) associates with SF1 and U2AF65 and modulates alternative pre-mRNA splicing in vivoRNA201521343845725605964
- MosesJGoodchildARivoryLPIntended transcriptional silencing with siRNA results in gene repression through sequence-specific off-targetingRNA201016243044120026621
- Sánchez-ÁlvarezMGoldstrohmACGarcia-BlancoMASuñéCHuman transcription elongation factor CA150 localizes to splicing factor-rich nuclear speckles and assembles transcription and splicing components into complexes through its amino and carboxyl regionsMol Cell Biol200626134998501416782886
- BermejoMLopez-HuertasMRGarcia-PerezJDasatinib inhibits HIV-1 replication through the interference of SAMHD1 phosphorylation in CD4+ T cellsBiochem Pharmacol2016106304526851491
- SamadikhahHRMajidiANikkhahMHosseinkhaniSPreparation, characterization, and efficient transfection of cationic liposomes and nanomagnetic cationic liposomesInt J Nanomedicine201162275228322072865
- HuangQDOuWJChenHNovel cationic lipids possessing protonated cyclen and imidazolium salt for gene deliveryEur J Pharm Biopharm201178332633521439377
- BiswasJBajajABhattacharyaSMembranes of cationic gemini lipids based on cholesterol with hydroxyl headgroups and their interactions with DNA and phospholipidJ Phys Chem B2011115347848621192731
- IslamRUHeanJvan OtterloWAde KoningCBArbuthnotPEfficient nucleic acid transduction with lipoplexes containing novel piperazine- and polyamine-conjugated cholesterol derivativesBioorg Med Chem Lett200919110010319028097
- PozziDMarchiniCCardarelliFTransfection efficiency boost of cholesterol-containing lipoplexesBiochim Biophys Acta2012181892335234322627109
- CortesiRCampioniMRavaniLDrechslerMPinottiMEspositoECationic lipid nanosystems as carriers for nucleic acidsN Biotechnol2014311445424120492
- XinYHuangMGuoWWHuangQZhangLZJiangGNano-based delivery of RNAi in cancer therapyMol Cancer201716113428754120