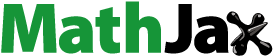
Abstract
Cancer has become one of the primary causes of death worldwide. Current cancer-therapy schemes are progressing relatively slowly in terms of reducing mortality, prolonging survival, time and enhancing cure rate, owing to the enormous obstacles of cancer pathophysiology. Therefore, specific diagnosis and therapy for malignant tumors are becoming more and more crucial and urgent, especially for early cancer diagnosis and cancer-targeted therapy. Derived theranostics that combine several functions into one “package” could further overcome undesirable differences in biodistribution and selectivity between distinct imaging and therapeutic agents. In this article, we discuss a chief clinical diagnosis tool – MRI – focusing on recent progress in magnetic agents or systems in multifunctional polymer nanoassemblies for combing cancer theranostics. We describe abundant polymeric MRI-contrast agents integrated with chemotherapy, gene therapy, thermotherapy, and radiotherapy, as well as other developing directions.
Introduction
Along with the aging and growth of the world population, cancer is the primary cause of death in economically developed countries and the secondary one in developing countries.Citation1 In 2017, 1,688,780 new cancer cases and 600,920 cancer deaths were estimated in the USA.Citation1 A significant proportion of the worldwide cancer issues could be prevented by popularizing cancer knowledge and implementing programs for tobacco and alcohol control, vaccination (for liver and cervical cancers), routine detection and treatment, and promoting physical activity and healthier dietary patterns by public health campaigns.Citation2 As a key link, the early diagnosis and radical therapy of malignant tumors can obviously improve the survival and even healing probability of cancer patients. Particularly, many novel materials and technologies have been applied to greatly promote therapeutic efficacy for cancer in recent years.
In 2002, the term “theranostic” first appeared, being defined as the multimodal combination of therapy and diagnostic imaging by Funkhouser.Citation3 It means that therapeutic drugs and imaging agents are packaged at the same dose and transported at the same time. Such a combination of several features into one package may overcome undesirable differences in biodistribution and selectivity existing between distinct imaging and therapeutic agents.Citation4 For example, chemotherapeutic drugs are considered for cancer treatment. Most of these routine anticancer drugs suffer from poor pharmacokinetics, inappropriate biodistribution, growing multidrug resistance, and widespread toxicity in vivo to a variety of healthy tissue. They are generally unable to accumulate well at the pathological site or cleared out from the circulation easily and rapidly. Consequently, many agents that are highly effective in vitro often appear not only relatively ineffective but also toxic when administered in vivo.Citation5,Citation6 However, after these drugs are integrated into one theranostic system, clearly their visualization by diagnostic imaging is very helpful in real-time gains in important information on diseased tissue, delivery kinetics, and drug efficacy to tune the drug-dosage and treatment protocols. In contrast to adopting a traditional “One size fits all” approach, a “Two or more is better than one” philosophy integrated with multifunctional theranostic systems can work out more individualized solutions to improve curative effect significantly according to patients’ real-time condition.Citation4,Citation5,Citation7 Characteristic features are detailed in . These incorporations of contrast agents and pharmacologically active agents into one nanomedicine may allow biodistribution and accumulation to be visualized noninvasively to monitor drug release and response in real time for combined theranostics.
Figure 1 Schematic representation of applications of nanotheranostics and image-guided drug delivery to improve curative effect.
Notes: Reprinted with permission from Lammers T, Kiessling F, Hennink WE, Storm G. Nanotheranostics and image-guided drug delivery current concepts and future direction. Mol Pharm. 2010;7:1899–1912.Citation5 Copyright 2010, American Chemical Society.
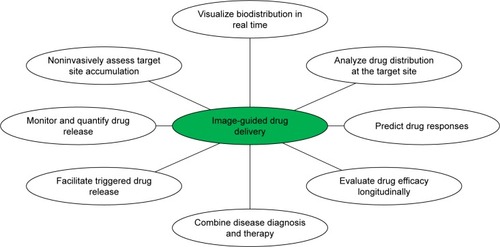
As such, in cancer treatment, therapy and diagnosis are usually integrated into one term: “cancer theranostics”. After significant advances over the past several decades, current diagnostic and therapeutic approaches still rely predominantly on invasive (ie, random biopsies and surgery) and crude, aspecific techniques, such as irradiation and chemotherapeutic agents. From a clinical perspective, cancer is almost uniformly fatal.Citation8 Therefore, there is still an urgent need to improve the susceptibility, accuracy, and specificity of cancer theranostics.
In this review, we focus on various magnetic polymeric nanoassemblies to display the nature of bifunctional or multifunctional MRI-contrast agents from the perspective of their theranostic types, including classification, imaging principles, and specific strategies for high signal sensitivity. Particularly, we introduce magnetic polymeric nanoassemblies into MRI-integrated cancer theranostics combined with chemotherapy, gene therapy, thermotherapy, and radiotherapy, and systematically display their recent development, as shown in .
Polymeric nanoassemblies in cancer theranostics
In 1975, a rational model for pharmacologically active polymers was proposed by Ringsdorf.Citation9 The Ringsdorf model is primarily aimed at covalently bound polymer–drug conjugates, and consists of three components: a polymeric solubilizer, a drug usually bound to a polymer backbone via a linker, and a targeting moiety to guide a drug-delivery system to a determined target. The enhanced permeability and retention effect, defined as the greater permeability of tumor vessels for macromolecules than normal vessels and the impaired clearance of these macromolecules from the interstitial space of the tumor (contributing to longer retention of these molecules), will greatly facilitate polymer–drug conjugates accumulated within desired physiological regions.Citation10
The drugs mentioned are not limited to just chemical drug molecules, and the composites of polymer–drug conjugates from interactions among drugs and polymer chains can also be regarded as “drugs”. Various drug–polymer hybrids, including polymeric conjugates, composites, dendrimers, hydrogel, microspheres, microcapsules, microbubbles, nanospheres, micelles, vesicles, nanorods, and nanowafers, have been used as theranostic polymer agents for cancer management,Citation10–Citation14 as summarized in .
Figure 3 Nanoscale drug carriers used as theranostic polymer agents for cancer management.
Notes: (A) Liposomes, (B) polymeric micelles, (C) polymer–drug conjugate, (D) dendrimer, (E) nanoemulsion, (F) mesoporous silica nanoparticles, and (G) iron oxide nanoparticles. Reprinted from Biochem Pharmacol, 83, Hu CM, Zhang L, Nanoparticle-based combination therapy toward overcoming drug resistance in cancer, 1104–1111.Citation11 Copyright 2012, with permission from Elsevier.
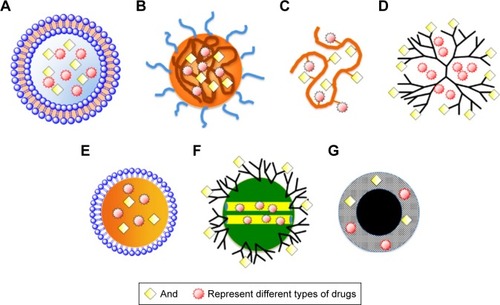
MRI-contrast agents
From a clinical point of view, MRI is one of the most powerful and noninvasive imaging tools for disease diagnosis, widely used in neurological, musculoskeletal, cardiovascular, and oncological imaging. MRI has the advantages of no radiation harm, high sensitivity to blood flow, and yielding great contrast between different soft tissues. Magnetic nanoparticles (MNPs) represent a promising nanomaterial for targeted therapy and imaging.Citation15 MRI is an effective clinical imaging tool for the theranostics of cancer.
Based on the principle of nuclear MR spectroscopy, MRI images are generated by spatially encoding the nuclear MR signal coming from nuclei (eg, protons) presented in the object and through the application of time-varying, linear magnetic field gradients.Citation16 However, MRI contrast is often insufficient in clinical practice. A number of contrast agents have been introduced to enhance image contrast between normal and diseased tissue by increasing the relaxation rates of water protons in tissue in which the agent accumulates, thus being helpful for radiologists to make a more precise diagnosis on the status of organ function or blood flow.Citation17
Classification of MRI-contrast agents
The image contrast in MRI is typically classified by its sensitivity to three tissue parameters: proton density, longitudinal (or spin–lattice) relaxation time (T1), and transverse (or spin–spin) relaxation time (T2). Currently available MRI-contrast agents can be divided into two classes: T1 agents and T2 agents. T1 agents generally generate positive image contrast by increasing longitudinal relaxation rates (1/T1) of surrounding water protons in tissue more than transverse relaxation rates (1/T2). With conventional pulse sequences, this dominant T1-lowering effect gives rise to increased signal intensity and predominance on T1-weighted images. Commonly, Mn2+ (LumenHance) and Gd3+ (Magnevist enteral and Gadolite) are used as metal centers of the most common T1 agents. Recently, however, Wei et alCitation18 designed and developed a gadolinium (Gd)-free T1-contrast agent with exceedingly small zwitterion-coated superparamagnetic iron oxide NPs (IONPs). Similarly, T2 agents generally generate negative image contrast by selectively increasing the 1/T2 of tissue to decrease in signal intensity and be predominant on T2-weighted images. Some common T1/T2 agents are summarized in , including Gd-DTPA-BMA (OmniScan), Gd-DTPA (Magnevist), Gd-HPDO3A (ProHance), Gd-DO3A–butrol (Gadovist), Mn-DPDP (Teslascan), and Gd-DOTA (Dotarem), Fe3O4 NPs, MnFe2O4 NPs, FeCo NPs, and FePt NPs.
Figure 4 (A) Chemical structure of representative T1 agents (left) and transmission electron microscopy images of exemplary T2 agents (right). (B) T1 relaxation (left) and T2 relaxation (right). Mz(t), longitudinal magnetization; Mz(0), longitudinal magnetization of original equilibrium state; Mxy(t), magnetization in the xy-plane; Mxy(0), magnetization in xy-plane of original equilibrium state; , relaxation time of pure water; [M], concentration of contrast agent; r1/r2, relaxivity. *Gd-DTPA (Magnevist) is a commercial MRI contrast agent like OmniScan™.
Note: Reproduced from Khemtong C, Kessinger CW, Gao J. Polymeric nanomedicine for cancer MR imaging and drug delivery. Chem Commun (Camb). 2009:3497–3510.Citation19 Copyright 2009, with permission of The Royal Society of Chemistry.
Abbreviation: Gd, gadolinium.
![Figure 4 (A) Chemical structure of representative T1 agents (left) and transmission electron microscopy images of exemplary T2 agents (right). (B) T1 relaxation (left) and T2 relaxation (right). Mz(t), longitudinal magnetization; Mz(0), longitudinal magnetization of original equilibrium state; Mxy(t), magnetization in the xy-plane; Mxy(0), magnetization in xy-plane of original equilibrium state; T1,H2O/T2,H2O, relaxation time of pure water; [M], concentration of contrast agent; r1/r2, relaxivity. *Gd-DTPA (Magnevist) is a commercial MRI contrast agent like OmniScan™.Note: Reproduced from Khemtong C, Kessinger CW, Gao J. Polymeric nanomedicine for cancer MR imaging and drug delivery. Chem Commun (Camb). 2009:3497–3510.Citation19 Copyright 2009, with permission of The Royal Society of Chemistry.Abbreviation: Gd, gadolinium.](/cms/asset/394c51c8-8ee2-4c55-a615-b01f55ef110d/dijn_a_12194079_f0004_c.jpg)
Furthermore, the mechanisms of T1-weighted imaging and T2-weighted imaging (γ-Fe2O3 and Fe3O4 [Lumirem, GastroMark, and Abdoscan] as central moiety of the most common T2 agents) are shown in .Citation19–Citation22 Briefly, MRI is the reflection of an atomic magnetic moment after introducing a magnetic pulse. In the absence of an external magnetic field, the proton has a magnetic momentum (ω0) that deflects from the central axis, as shows. With application of an external magnetic field to the proton, the direction of magnetization will align to the field direction and revert to its original state when the magnetic field disappears, and signals from this process will be collected and changed into MRI images. The process of the system recovered from the excitation state to original state is defined as the relaxation state. In general, the magnetization (Mz) of z-axis () is called longitudinal relaxation or T1 relaxation, and the magnetization (Mxy) of the xy-plane () is called transverse relaxation or T2 relaxation, with reciprocals of T1/T2 relaxation defined as r1/r2 relaxivity. In biomedicine diagnosis, results are visual output as weighted images, such as T1- and T2-weighted images. Signal-intensity formulae of T1- and T2-weighted images are shown in . According to the formulae and the curves between magnetization and relaxation time, we can know that the T1-weighted image will be brighter if T1 relaxation is short enough, and the T2-weighted image will be darker if T2 relaxation is short enough.
Recently, significant progress has been achieved in the development of MRI-contrast systems. This greatly facilitates differentiation of cancerous tissue from surrounding normal tissue by using magnetic fields and radio waves, which might make the early diagnosis of cancer with high accuracy possible.Citation8,Citation17,Citation18,Citation23 The introduction of various polymer carriers has been carried out to develop crude MRI-contrast agents into multifunctional vector integrated with therapeutic (even diagnostic) approaches for advanced cancer theranostics.
How to increase signal sensitivity
In terms of Solomon–Bloembergen–Morgan theory, T1-relaxation enhancement depends on four key parameters: hydration number (q; number of labile water molecules coordinated with the metal), residence time (τM; lifetime of coordinated water molecules at the metal site), rotational correlation time (τR; tumbling motion of the complex), and relaxation characteristics of the unpaired electrons of the metal ion (T 24 iE). The relationship between T1 relaxivity (r1IS) and inner-sphere water is described by EquationEquation 1(1) :Citation25
For T2 agents (eg, superparamagnetic Fe3O4 NPs), the spin–spin relaxivity (R2; 1/T2) represents the degree of T2-contrast effect, namely that higher R2 corresponds with greater contrast effect. At present, the relationship between NP characteristics and R2 of the proton could be described by:Citation28–Citation31
Fe3O4 NPs are a typical example of a contrast-enhanced agent for T2-weighted imaging. On the basis of a quantum-mechanical outer-sphere theory, the T2 relaxivity of Fe3O4 NPs in solution can be given by:
Considering that Ms is the maximum induced magnetic moment obtained in the magnetic field, R2 is strongly related to γ, coating materials, and the magnetization of NPs is dependent on size, composition, and magnetocrystalline phase, referring to EquationEquations 2(2) –Equation4
(4) .Citation31,Citation33,Citation34 Efforts to improve the efficiency of T2 agents can be generally rooted in nanocrystal size and compositionCitation34 and hydrodynamic size.Citation35–Citation37 Compared to traditional colloidal T2 agents, such as Feridex, Combidex, and Resovist, emerging superparamagnetic oxide NPs with high crystallinity, uniform size, and excellent morphology can generate higher magnetization and further greater T2-reducing capability. On the other hand, while superparamagnetic NPs are gathered together into small clusters, R2 can also be improved immensely.Citation35,Citation36,Citation38
R2 is proportional to the size of the nanocluster, although the exact reason behind this phenomenon is still unclear.Citation35 Therefore, the regulation of magnetic nanocrystals’ self-assembly may become another available approach to enhance contrast effect greatly in T1-weighted imaging.
MRI-combined chemotherapy
Although limited in efficacy, cancer chemotherapy has remained one of the most common treatment modalities for many malignant tumors since the early 1950s.Citation5–Citation7,Citation39 In the case of most anticancer drugs, the lack of specificity toward malignant tissues tends to present a large volume of distribution and then cause significant toxicity and side effects, along with poor solubility in body fluid or blood. Moreover, their low molecular weight makes them easy to be rapidly cleared from the circulation for intravenous administration and not to accumulate well at the pathological site.Citation5,Citation6 Therefore, in order to develop cancer therapy, two important approaches must be addressed: developing novel probes with high accuracy and precision for early diagnosis, and further enhancing pharmacological specificity or targeting during drug metabolism.Citation7 Nowadays, multifunctionalized drug-delivery systems have made the integration of MRI and chemotherapy into one “device” come true.
T1 agents
As described earlier, Gd3+ is the metal center of the most common T1 agents, and many studies on nanocarriers loading Gd and anticancer drugs as T1-enhanced contrast agents have been reported recently. Vinh et alCitation40 prepared MRI-detectable polymeric micelles containing Gd-diethylenetriaminepentaacetic acid (DTPA) and the platinum anticancer drug dichloro(1,2-diaminocyclohexane)platinum(II) (DACHPt). The longitudinal T1 relaxivity (r1) of Gd-DTPA/DACHPt-loaded micelles greatly increased to 80.7 mmol·L−1·s−1, which provided strong and specific tumor-contrast enhancement of T1-weighted MRI in N1S1 tumor-bearing Sprague Dawley rats. Not only that, this micelle induced high levels (92.4%±3.4%) of tumor apoptosis at 3 days postinjection, but also tumor size and growth in vivo were significantly suppressed without severe adverse reactions (). Likewise, Ma et alCitation41 constructed renal-clearable NPs consisting of Gd-DTPA and the water-insoluble anticancer drug camptothecin for MRI and chemotherapy. Its r1 of 4.66 mM−1·s−1 showed significant positive contrast enhancement in nude mice bearing LoVo tumors. Slightly different to this nanosystem, Tong et alCitation42 developed a functionalized polymer of 1,4,7,10-tetraazacyclododecane-1,4,7,10-tetraacetic acid–polyethylene glycol (PEG)-block-polyacrylamide-co-acrylonitrile to form temperature-responsive micelles coloading doxorubicin (Dox) and Gd ions (Gd3+). The micelles exhibited noticeable accelerated Dox release along with rising temperature, and enhanced MRI in BEL7402 cells with high proton relaxivity (25.88 mM−1·s−1).
Figure 5 (A) Preparation of Gd-DTPA/DACHPt-loaded micelles by self-assembly. (B) Tumors, histopathology, and tumor apoptosis. Macroscopic images of an N1S1 hepatic tumor (a–c). Microscopic analysis of H&E-stained tumor sections (showed high levels of tumor apoptosis) and observations from TUNEL, DAPI, and merged images (d, e).
Note: Reprinted from Vinh NQ, Naka S, Cabral H, et al. MRI-detectable polymeric micelles incorporating platinum anticancer drugs enhance survival in an advanced hepatocellular carcinoma model. Int J Nanomedicine. 2015;10:4137–4147.Citation40 Copyright 2015. Dove Medical Press.
Abbreviations: Gd, gadolinium; DTPA, diethylenetriaminepentaacetic acid; DACHPt, dichloro(1,2-diaminocyclohexane)platinum(II); TUNEL, terminal deoxynucleotidyl transferase deoxyuridine triphosphate nick-end labeling; DAPI, 4′,6-diamidino-2-phenylindole. Citation1
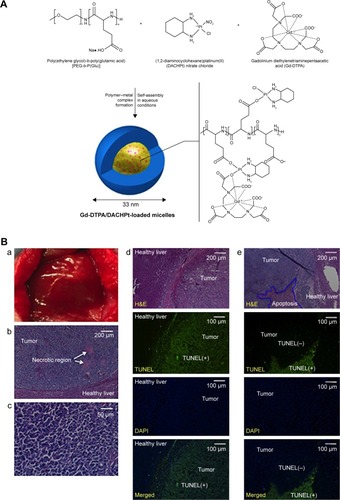
Both magnetic manganese oxide (MnO2) and manganese ions are another category of common T1 agents, and have been studied deeply. For example, Abbasi et alCitation43 prepared polymeric theranostic NPs encapsulating docetaxel (Dtx), fluorescent dye, and MnO2 NPs. The novel polymeric theranostic NPs not only sustained in vitro release of Dtx, enhanced energy-dependent cellular uptake, and extended cytoplasmic retention in MDA-MB-231 cells but also enhanced r1 (2.4 mM−1·s−1) to achieve contrast enhancement of T1-weighted images in a xeno-graft orthotopic human breast-tumor model. Similarly, Hao et alCitation44 constructed multifunctional poly(lactic-co-glycolic acid) (PLGA) NPs containing hematoporphyrin monomethyl ether and MnO2 as T1 agents as well as a drug carrier. Dong et alCitation45 encapsulated manganese ions with indocyanine green and Dox in theranostic polydopamine NPs with integrated chemotherapy, photothermal therapy (PTT), and MRI. In summary, the theranostic system that combined T1-weighted MRI and chemotherapy showed positive contrast enhancement and inhibition efficacy on tumor cells simultaneously.
T2 agents
As the simplest solution, a class of effective theranostic nanoplatforms is developing directly based on the core of MNPs. Asadi et alCitation46 built pH-sensitive and random copolymer-modified superparamagnetic IONPs (SPIONs) as a promising nanocarrier for MRI and Dox delivery. Both excellent cytocompatibility and cellular uptake in DU145 cells were apparent in this integrated system. It displayed acid-accelerated drug release and MRI-contrast enhancement (r2 =134.4 mM−1·s−1). Likewise, Liang et alCitation47 designed functionalized SPION with a shell of PEGylated Dox. The nanocarriers significantly prolonged the half-life of Dox in blood circulation, improved uptake of HT29 cells under the magnetic field, and strengthened the r2:r1 ratio and T2-weighted signals in an in vivo MRI study of rats. Malekzadeh et alCitation48 developed a multifunctional quercetin-loaded nanosystem with a core of SPIONs developed through layer-by-layer self-assembly of poly(citric acid) dendrimer, PEG, and folic acid. The total release time of quercetin was prolonged, and excellent cellular uptake and cytotoxicity to HeLa cells and MDA cells were very evident. Of course, the nanovector also led to appreciable negative contrast enhancement in T2-weighted MRI.
Feng et alCitation49 chose hollow, mesoporous, Dox-loaded, SPION-entrapped, PEGylated copper sulfide (CuS) NPs to construct smart controllable on-demand cargo delivery (). The metal nanohybrid possessed elevated photothermal conversion efficiency (42.12%) for enhanced PTT effect and controlled on-demand drug release upon exposure to a near-infrared (NIR) stimulus for high tumor cytotoxicity. Both in vitro and in vivo experiments revealed remarkable antitumor therapeutic efficacy with the synergistic combination of MRI, photodynamic therapy, PTT, and chemotherapy under NIR irradiation. Similarly, Ren et alCitation50 constructed a novel paclitaxel-loaded nanocomposite that encapsulated cysteine-functionalized SPIONs, CuS, and paclitaxel into BSA aggregation. These nanoagents can not only absorbed NIR light and transformed it into heat effectively to trigger drug release within the tumor site but also brought sharp T2-weighted contrast enhancement on a HeLa tumor-bearing mouse. Moreover, An et alCitation51 utilized glycopolymer-modified magnetic mesoporous silica NPs for liver cancer treatment. The significant recognition ability of HepG2 cells for this nanocarrier greatly facilitated intracellular uptake, controlled drug release, HepG2-cell cytotoxicity, and the contrast effect of T2-weighted MRI in livers of nude mice.
Figure 6 Synthesis of the drug-delivery system (HMCuS/Dox@IONP-PEG) for combining MRI with chemophototherapy.
Note: Reprinted from Acta Biomater, 49, Feng Q, Zhang Y, Zhang W, et al, Programmed near-infrared light-responsive drug delivery system for combined magnetic tumor-targeting magnetic resonance imaging and chemo-phototherapy, 402–413.Citation49 Copyright 2017, with permission from Elsevier.
Abbreviations: Dox, doxorubicin; HM, hollow mesoporous; NPs, nanoparticles; IO, iron oxide; NIR, near-infrared; PTT, phototherapy; PDT, photodynamic therapy; PEG, polyethylene glycol; IV, intravenous; ROS, reactive oxygen species.
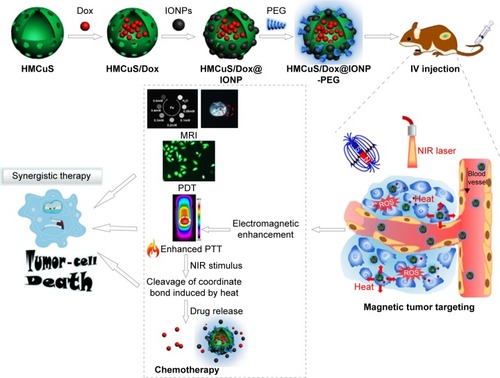
More often, all kinds of polymeric self-assemblies are chosen as appropriate delivery system for MNPs and anti-tumor drugs. Du et alCitation52 constructed temperature-sensitive, folate-targeted, Dtx-loaded magnetic PEGylated liposomes containing fullerene (C60)-decorated and PEGylated SPIONs. The multifunctional liposomes achieved the integration of MRI, photothermal tumor ablation, and fullerene radiofrequency-triggered drug release. In vitro and in vivo experiments indicated its high stability, temperature-adjusted release rate, and resulting high efficiency of inhibition on MCF7 cells under 13.56 MHz radiofrequency. In addition, these liposomes gave much brighter T2-weighted images in female C57 mice bearing B16F10 tumors (). In the same way, Wang et alCitation53 reported on reduction-responsive PEGylated lipid vesicles coloading SPIONs and Dox. The release rate of Dox from these novel vesicles was up to 68.9% in 36 hours in the reduction environment. Cellular uptake, antitumor effect (IC50=1.39 μg·mL−1), and T2-weighted MRI capability toward HeLa cells were enhanced signifi-cantly. Other reduction-sensitive theranostic nanocarriers based on amphiphilic disulfide-linked dextran-g-poly(N-ω-carbobenzyloxy-l-lysine) (Dex-g-SS-PZLL) were studied by Yang et al.Citation54 Dox–SPION-coloaded Dex-g-SS-PZLL micelles also exhibited reduction-sensitive properties and led to rapid Dox release, higher toxicity to HepG2 cancer cells, and high MRI T2-weighted imaging sensitivity (r2=261.3 mM−1·s−1).
Figure 7 (A) Design of functional liposomes with RF-triggered drug release and RF targeted thermochemotherapy using the nanocomposite. (B) In vivo T2-weighted magnetic resonance imaging of multifunctional liposomes with a magnet (left to right: control group and at 1, 4, and 7 hours after injection, respectively).
Note: Reproduced from Du B, Han S, Li H, et al. Multi-functional liposomes showing radiofrequency-triggered release and magnetic resonance imaging for tumor multi-mechanism therapy. Nanoscale. 2015;7:5411–5426.Citation52 Copyright 2015, with permission from the Royal Society of Chemistry.
Abbreviations: Dtx, docetaxel; IONPs, iron oxide nanoparticles; C60, fullerene; PEG, polyethylene glycol; DPPC, dipalmitoylphosphatidylcholine; DSPE, distearoylphosphati-dylethanolamine; RF, radiofrequency.
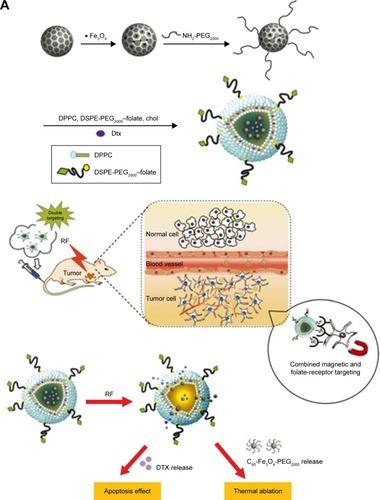
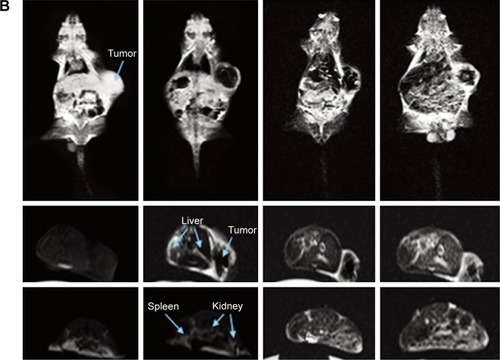
Li et alCitation55 further developed folate-conjugated and PEGylated PLGA NPs with a loaded anticancer drug (sorafenib) and SPIONs for liver cancer therapeutics. This nanocarrier also exhibited sustained drug release, enhanced cellular uptake in cancer cells, effectively suppressed tumor-cell proliferation, and enhanced MRI efficiency. They also studied the colony-forming ability of BEL7402 cells to confirm superior antitumor efficacy. Mosafer et alCitation56 also applied PLGA NPs as the framework to develop AS1411 aptamer-targeted nanoassemblies coloading SPIONs and Dox against murine C26 colon carcinoma cells. This nanoplatform had slow Dox release over 20 days and prominent cellular uptake and cytotoxicity. These characteristics contributed to improved anti-colon cancer efficacy and contrast effect of T2-weighted MRI in vivo. Jaidev et alCitation57 prepared multifunctional PLGA nanospheres containing fluorescent SPIONs and gemcitabine with a surface bonded with HER2 antibody for pancreatic cancer therapy and imaging. Apparently, this theranostic nanosystem achieved sustained release of gemcitabine for 11 days, great inhibition of tumor cells, and significant tumor regression (86%±3%) in male severe combined immunodeficient mice. Moreover, high r2 relaxivity (773 mM−1·s−1) and a specific absorption rate (183 W·g−1) were determined, which are beneficial to MRI-contrast enhancement and thermotherapy, respectively.
Situ et alCitation58 synthesized A54 peptide-functionalized PLGA-grafted dextran (A54-DexPLGA) for the formation of A54 homing peptide-functionalized micelles with Dox and SPIONs. The micelles revealed good sustained release and MRI-contrast enhancement similarly to other nanocarriers. Distinctively, specific targeting to BEL7402 cells caused greater antitumor activity in BEL7402 cells (IC50=0.56 μg mL−1) and tumor-inhibition rate of micelle treatments (72.53%) in orthotopic implantation hepatoma models. Lin et alCitation59 also studied a multifunctional nano-composite based on stearic acid chain-grafted amphiphilic dextran (Dex-g-SA) and carbohydrate and manganese-doped IONPs (Mn-SPIONs) for Dox delivery and MRI. The Mn-SPION-Dox-loaded Dex-g-SA micelles showed low IC50 of 1.2 μmol·L−1 against MCF7 cells and 15 μmol·L−1 against MCF-7/Adr cells. Naturally, it also had good uptake of MCF7/Adr cells and obvious MRI-signal darkening under clinical 3T MRI scanning.
A rather more complex case is multiside groups or multiblock polymers being employed as framework materials. Du et alCitation60 developed N-succinyl-N′-4-(2-nitrobenzyloxy)-succinyl-chitosan micelles loading C60, SPIONs, Dtx and upconversion nanophosphors. The micelles regulated controlled drug release on demand by NIR light to improve photodynamic therapy and ROS generation in tumor cells effectively for tumor inhibition. Meanwhile, this novel nano-composite was also employed for noninvasive deep MRI and upconversion fluorescence imaging. Another example is a multifunctional PEG-PCL nanocarrier with encapsulated SPIONs and busulfan.Citation61 The theranostic NPs showed sustained drug release over 10 hours and great contrast enhancement in T2-weighted MRI with high r2 (103.3 Fe mM−1·s−1). In addition, VivoTag 680XL fluorochrome was also labeled to the nanocarriers to observe dynamic biodistribution in vivo and uptake in different organs by fluorescence imaging in real time. Similarly, Lee et alCitation62 proposed a multifunctional theranostic micelle of cationic PDMA-b-PCL and PEG-PCL for loading 7-ethyl-10-hydroxycamptothecin (SN38), ultrasmall SPIONs, and siRNA. This micellar system improved the biostability of the loaded drug and siRNA, prolonged drug release and circulation time, and provided effective T2-weighted MRI capability. Furthermore, it also efficiently silenced VEGF expression in tumor cells.
More complex and advanced magnetite NPs have also been wrapped inside polymeric nanosystems, such as magnetite nanoclusters (MNCs). Wu et alCitation63 designed a charge-switchable nanohybrid (HNP-DA) comprising of MNCs, a paclitaxel-loaded core of amphiphilic polymer (pluronic F127), and a hydrophilic polymeric shell of stearoyl-polyethylenimine-2,3-dimethylmalefic anhydride. The surface charge conversion of HNP-DA from negative to positive obviously enhanced uptake efficiency in cancer cells. In vitro studies of HepG2 cells demonstrated that this nanocarrier exhibited very high cytotoxicity at pH 6.5 (,15% cell viability). In addition, the r2 of the HNP-DA was improved to 142.68 mM−1·s−1. Likewise, Hayashi et alCitation64 synthesized folic acid and PEG-decorated NPs with a core of MNCs and shell of Dox-containing polymers. These core–shells realized controlled Dox release for continuous chemotherapy over a long time and enhanced r2 to 357 mM−1·s−1. Further under alternating a magnetic field, the core–shells induced heat generation to accelerate drug release within the tumors for outstanding therapeutic efficacy ().
Figure 8 (A) Synthesis of magnetic resonance imaging-guided magnetic thermochemotherapy core–shell agents. (B) Photography and T2-weighted magnetic resonance imaging of mice subjected to core–shell injection. (C) Thermal images of mice with and without magnetic field or core–shell injection under influence of atomic force microscopy (AMF). The white circles indicate abdominal tumor sites.
Note: Reprinted from Hayashi K, Sato Y, Sakamoto W, Yogo T. Theranostic nanoparticles for MRI-guided thermochemotherapy: “tight” clustering of magnetic nanoparticles boosts relaxivity and heat-generation power. ACS Biomater Sci Eng. 2017;3:95–105.Citation64 Copyright 2017, with permission from the American Chemical Society.
Abbreviations: Dox, doxorubicin; PEG, polyethylene glycol; FA, folic acid; Mag, magnetic.
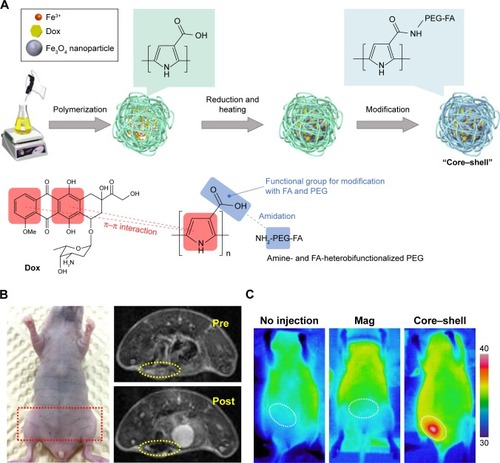
Compared with SPIONs, ferric oxide NPs (γ-Fe2O3) are another common superparamagnetic T2-weighted contrast agent. For example, Wang et alCitation65 developed an innovative tumor-specific multifunctional nanoplatform comprising γ-Fe2O3 core and chitosan shell modified with tumor-specific ligand transferrin for anti-tumor theranostics. Thorat et alCitation66 encapsulated γ-Fe2O3 developed together with Dox into bis(p-sulfonatophenyl)phenylphosphine–methoxypolyethylene glycol–thiol vesicles for consideration of both controlled drug release and enhanced MRI contrast. All results were positive, testifying that this nanocarrier could be also used for synergetic magnetochemotherapy. IONPs, the central moiety of the most common T2 agents, were studied mostly. Others, like γ-Fe2O3, magnetic mesoporous silica NPs, and magnetite nanoclusters, were also mentioned as the central moiety. These theranostic systems exhibited excellent negative contrast enhancement in T2-weighted MRI on account of the loading of T2-weighted central moieties, and revealed better results for drug delivery and antitumor efficacy.
MRI-combined gene therapy
Gene therapy that involves the delivery of therapeutic genetic material into specific target cells is regarded as a new medical approach for the treatment of tumors. In 1991, the first clinical trial of cancer gene therapy was performed in melanoma patients. To date, most strategies for cancer gene therapy can be classified into five main categories: suicide-gene therapy, rehabilitation of aberrant cell cycle, immunomodulatory gene therapy, antiangiogenesis gene therapy, and oncolytic gene therapy.Citation67–Citation69
Among many nonviral gene vectors, polyethylenimine (PEI) is one of the most effective gene-delivery vehicles studied to date. Owing to high charge density, it can effectively bind genes or oligonucleotides to form stable complexes that can be swallowed easily by cells and escape from intracellular organelles (eg, endosomes) through the proton-sponge effect.Citation70 Generally, 25 kDa branched PEI and 22 kDa linear PEI are applied as golden standards to evaluate nonviral transfection agents.Citation71 The presence of primary and secondary amine groups within PEI branches also facilitates various chemical modifications for enhanced functionality.Citation72 Hydrophobic moieties incorporated into gene-delivery systems are believed to enhance overall cell interactions and tissue permeability.
A new kind of Gd-chelated cationic poly(urethane amide) copolymer (GdCPUAs) was investigated for gene delivery and T1-weighted MRI by Gao et al.Citation73 As shown in , the Gd-chelated and plasmid DNA-loaded poly-plexes showed very high transfection efficiency in different cancer cells and significant T1-contrast enhancement in vitro (r1=11.8 mM−1·s−1). All the results testified to great potential of biodegradable Gd-chelated cationic poly(urethane amide) copolymers for tumor theranostics. A theranostic micellar delivery system for synergetic gene therapy and T2-weighted MRI was presented.Citation60 Park et alCitation74 integrated multimodal imaging (MRI and Raman imaging) with combined hyperthermia and siRNA-based therapy in multifunctional MNPs with an iron cobalt core and graphitic carbon shell. This system exhibited a higher relaxivity coefficient (r2 =392 mM−1·s−1) and delivered small interfering RNA (siRNA) to tumor cells in targeted fashion together with hyperthermia therapy.
Figure 9 (A) Design of multifunctional SN38/USPIO-loaded siRNA-PEG micelle complexes. (B) Synthesis process and intracellular therapeutic mechanism of multifunctional SN38/USPIO-loaded siRNA-PEG micelle complexes.
Note: Reprinted from Biomaterials, 86, Lee SY, Yang CY, Peng CL, et al, A theranostic micelleplex Co-delivering Sn-38 and VEGF siRNA for colorectal cancer therapy, 92–105.Citation62 Copyright 2016, with permission from Elsevier.
Abbreviations: PDMA, poly(2-[dimethylamino]ethyl methacrylate); PCL, polycaprolactone; SN38, 7-ethyl-10-hydroxycamptonthecin; USPIO, ultrasmall superparamagnetic iron oxide; PEG, polyethylene glycol.
![Figure 9 (A) Design of multifunctional SN38/USPIO-loaded siRNA-PEG micelle complexes. (B) Synthesis process and intracellular therapeutic mechanism of multifunctional SN38/USPIO-loaded siRNA-PEG micelle complexes.Note: Reprinted from Biomaterials, 86, Lee SY, Yang CY, Peng CL, et al, A theranostic micelleplex Co-delivering Sn-38 and VEGF siRNA for colorectal cancer therapy, 92–105.Citation62 Copyright 2016, with permission from Elsevier.Abbreviations: PDMA, poly(2-[dimethylamino]ethyl methacrylate); PCL, polycaprolactone; SN38, 7-ethyl-10-hydroxycamptonthecin; USPIO, ultrasmall superparamagnetic iron oxide; PEG, polyethylene glycol.](/cms/asset/7524f3e7-aadc-44e9-b337-93c993233e2c/dijn_a_12194079_f0009_c.jpg)
MRI-combined thermotherapy
As previously mentioned, the combination of early diagnosis and precise treatment is ideal for cancer therapy, especially represented by thermotherapeutic NPs.Citation75
T1 agents
T1 agents with metal centers of Gd or Mn have also been combined with thermotherapy for cancer MRI imaging and treatment. Cao et alCitation76 chose Gd (III)-chelated silica nano-spheres (Gd@SiO2) as the framework to integrate Dox and indocyanine green, and then introduced poly (diallyldimethylammonium chloride) to the surface of the magnetic core, as shown in . In vitro results revealed that Dox release accelerated significantly at pH 5 or upon NIR irradiation. This was attributed to heat weakening in the electrostatic interactions between Dox and the surface of MNPs. Meanwhile, under laser irradiation, NPs improved therapeutic efficacy by their energy conversion from light to heat. Mi et alCitation77 developed PEG-b-polyanion micelles hybrid-ized with calcium phosphate and Gd-DTPA for imaging-guided Gd neutron-capture tumor therapy. The micelles possessed high molecular relaxivity (18.4 mM−1·s−1), suitable for T1-weighted MRI, and high fatality rate to murine colon adenocarcinoma (C26) cells under thermal neutron irradiation for significantly enhanced therapeutic effects. In addition, tumor growth was effectively suppressed after thermal neutron irradiation. With enhancement of MRI and high energy conversion, these systems not only can be used for T1-weighted MRI and thermal ablation therapy but also can aggregate with chemotherapy to improve the controlled-release ability of nanocarriers.
Figure 10 Synthesis of Gd@SiO2-PDC and Gd@SiO2-Dox/ICG-PDC.
Notes: Reprinted with permisison from Cao M, Wang P, Kou Y, et al. Gadolinium (III)-chelated silica nanospheres integrating chemotherapy and photothermal therapy for cancer treatment and magnetic resonance imaging. ACS Appl Mater Interfaces. 2015;7:25014–25023.Citation76 Copyright 2015, American Chemical Society.
Abbreviations: Gd, gadolinium; Dox, doxorubicin; ICG, indocyanine green; PDC, polydiallyldimethylammonium chloride; TEOS, tetraethyl orthosilicate.
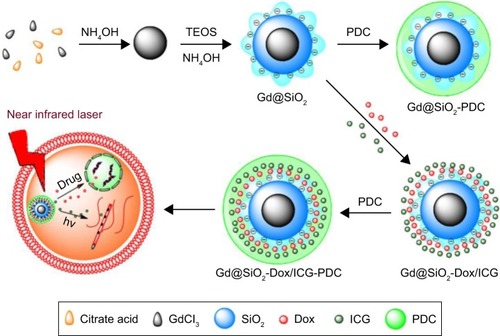
T2 agents
The integration of thermotherapy and MRI into a multifunctional nanoassembly has been achieved.Citation49,Citation50,Citation52,Citation60,Citation64,Citation78 Also, Chen et alCitation79 reported PEG-coated NPs with a core of gold NPs and a shell of ferric sulfide for combination of PTT and radiotherapy. In in vitro experiments, the cellular viability of 4T1 cancer cells was greatly inhibited after NP treatment and NIR irradiation. Under the synergistic action of thermoradiotherapy, these NPs significantly induced cell growth and death. Not only that, they also had high r2 of 213.84 mM−1·s−1, which was available for both in vitro and in vivo tumor MRI. Shah et alCitation80 also developed a magnetic core–shell NP-mediated delivery system for mitochondria-targeting proapoptotic amphipathic tail-anchoring peptide to malignant brain and metastatic breast cancer cells. This system not only can afford noninvasive MRI, but also significantly enhanced the efficacy of the peptide, due to the introduction of magnetic core–shell NP-mediated hyperthermia.
Additionally, dual-modal imaging, multimodal imaging combining thermotherapy, eg, T1–T2 dual-modal MRI and MRI–computed tomography (CT)–upconversion luminescence (UCL) multimodal imaging, have also been studied for cancer theranostics. The dysprosium ion (Dy3+) was researched deeply by Liu et al, due to the relatively large K-edge value and highest magnetic moment with the shortest electronic relaxation time of Dy3+. They designed an NaDyF4:50% Lu@Prussian blue nanocomposite for T1–T2-weighted MRI, CT, and PTT with the help of the Prussian blue shell.Citation81 They also developed a UCL-T2 dual-modal contrast agent with NaLuF4:Yb, Tm/NaLuF4/NaDyF4Citation82 and a lanthanide-based core−shell−shell nanocomposite NaYbF4:Tm@CaF2@NaDyF4 for upconversion MRI/CT multimodal imaging.Citation83 Liu et alCitation84 developed an Mn3O4 contrast agent capped with EDTA and BSA for efficient T1–T2 dual-modal imaging and PTT. They also reported an NIR second-window downconversion luminescence and T2 MRI-contrast agent based on ultrasmall Nd-doped NaDyF4.Citation85 These studies developed and optimized multifunctional materials for cancer theranostics and went into depth on the feasibility of multimodal imaging for cancer theranostics and imaging-guided PTT.
Magnetic fluid hyperthermiaCitation86 is also a superior method of thermotherapy used for cancer therapy, and is based on the property of the contrast agents within the magnetic field. Thorat et alCitation87 functionalized superparamagnetic La0.7Sr0.3MnO3 NPs with an oleic acid–PEG–polymeric micelle structure and loaded it with Dox. The design combined the effects of magnetic hyperthermia produced by particles under MRI and controlled drug release, and showed that cancer-cell killing was up to almost 90%. Other polymer-coated MNPs were studied by Boni et al.Citation88 They synthesized MNPs by decomposition of iron pentacarbonyl, growth with iron oleate, and further coating with polyamidoamine. The optimized MNPs (faceted-like) exhibited excellent transverse relaxivity (r2~300 mM−1·s−1) that was 2.5–3 times higher than the commercial Endorem and very high specific loss of power up to 900 W·g−1 at 262 kHz and 10 kA·m−1. This could be a suitable candidate contrast agent for simultaneous hyperthermic treatment and MRI diagnosis of cancer. In addition, Hatamie et alCitation89 also designed a novel MRI-contrast agent with graphene–cobalt nanocomposites, which exhibited superior conversion of electromagnetic energy into heat and achieved the integration of MRI and hyperthermia therapy for cancer. Liu et alCitation90 synthesized ferromagnetic Fe0.6Mn0.4O nanoflowers for T1–T2 dual-mode MRI and magnetic hyperthermia therapy. Both in vitro and in vivo results showed excellent magnetic induction-heating effects of the nanoflowers and great therapeutic effect on MCF7 breast cancer. To avoid local overheating, Herynek et alCitation91 studied novel silica-coated (La1–xSrxMnO3) ferromagnetic NPs with low Curie temperature, which induced cell apoptosis and cell death with thermoablation and MRI in cancer cells.
MRI-combined radiotherapy
Despite unavoidable organ exposure and potential cancer risk, radiotherapy is still one of the most effective treatments for malignant tumors.Citation92 Li et alCitation93 synthesized PEG-coated Au@MnS@ZnS core–shell–shell NPs for MRI-guided X-ray irradiation, as shown in . The results indicated that these NPs enhanced 4T1 cell-killing efficiency and inhibited tumor growth dramatically, owing to severe DNA damage in tumor cells under X-ray irradiation. Paramagnetic Mn2+ in the NP shell made these NPs usable as a T1-weighted MRI contrast agent to guide treatment section precisely for improving therapeutic efficacy. Similarly, Dou et alCitation94 developed a multifunctional PEG-decorated nanotheranostic with a core of Prussian blue NPs and a bonded satellite of gold NPs for synergistic MRI, CT, PTT, and radiosensitive therapy. These radial NPs combined the properties of both r1 (7.48 mM−1·s−1) and r2 (8.02 mM−1·s−1) values to achieve simultaneous T1- and T2-weighted MR contrast. After NIR and X-ray irradiation treatment, <11% of 4T1 cancer cells remained alive in vitro. Tumor growth in vivo was entirely suppressed, and the death of 4T1-xenograft tumor-bearing mice was also remarkably delayed under synergetic treatments. Novel trimodal theranostic NPs consisting of silica nanospheres, polysiloxane shells, and Bi/Gd-DOTA chelate-grafted outside surfaces were prepared by Detappe et al.Citation95 These NPs showed great MRI- and CT-contrast enhancement in vivo. They also attained outstanding improvement in tumor-growth delay followed by radiation in the subcutaneous xenograft solid-tumor model.
Figure 11 PEG-coated Au@MnS@ZnS core-shell-shell NPs for MRI-guided X-ray irradiation.
Notes: (A) Design of Au@MnS@ZnS NPs. (B) Transmission electron microscopy of Au@MnS@ZnS NPs. (C) Elemental mapping (Au, Mn, and Zn) of an Au@MnS@ZnS NP. According to the parameter of TEM and the rod of the figures, we can know that (B) was observed at 400× magnification. (C) was observed at 400× magnification. Reprinted with permission from Li M, Zhao Q, Yi X, et al. Au@MnS@ZnS core/shell/shell nanoparticles for magnetic resonance imaging and enhanced cancer radiation therapy. ACS Appl Mater Interfaces. 2016;8:9557–9564.Citation93 Copyright 2016, American Chemical Society.
Abbreviations: AuNPs, gold nanoparticles; C18PMH-PEG, polyethylene glycol-grafted poly(maleic anhydride-alt-1-octadecene).
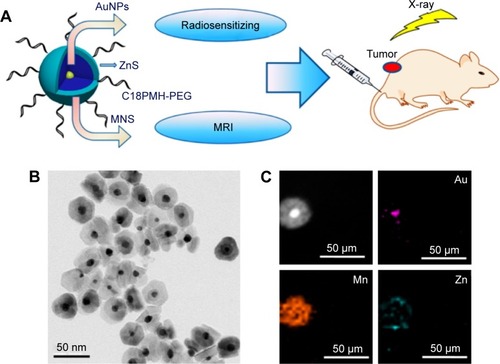
Conclusion
Some problems, including functional integrity, harmony, and mutual promotion, still exist, although cancer-specific theranostic agents or systems have made great progress. In future, several key points may be focused on in the design of more advanced multifunctional devices with enhanced imaging accuracy and therapeutic efficiency for cancer treatment:
MRI contrast agent with high imaging sensitivity. SPIONs’ magnetic susceptibility is relevant to their physical/chemical properties (eg, crystal form, size, surface property, aggregation density, and morphology), and Gd complexes depend on local concentration and interaction with water molecules. In addition, some doped rare-metal elements can also greatly improve contrast effects.
Tumor-targeting moiety with high specificity. Currently, specific peptides, antibodies, and aptamers with universal single tumor targeting have been studied. Their high tumor specificity would favor therapeutic agents or systems accumulated effectively within the cancer region.
Appropriate carriers. Besides size, both the morphology and surface properties of delivery systems can affect their stability, drug-release rate, cell uptake, and circulation time in vivo. Substrate material is crucial to achieve low toxicity, high stability, high loading efficiency, and controlled release. Particularly, drug-controlled release best possessed tumor-specific responsiveness due to the conversion from passivity to initiative. Now, amphiphilic, biodegradable, and biocompatible polymers have become the first choice.
Novel magnetic polymer nanoassemblies are constantly emerging, but almost none can enter clinical trials, let them alone improve theranostic effects or decrease costs. It seems only right to find a better solution with appropriate balance between structure and function to develop simple but effective integration for cancer theranostics.
Acknowledgments
This work was financially supported by Guangdong Provincial Key Laboratory of Optical Information Materials and Technology (grant 2017B030301007), the Special Fund on Public Interest Research and Capacity Building in Guangdong Province (2015A020211024), the Natural Science Foundation of China (No. 51773069), the Guangdong Innovative Research Team Program (2013C102), MOE International Laboratory for Optical Information Technologies, and the 111 Project.
Disclosure
The authors report no conflicts of interest in this work.
References
- SiegelRLMillerKDJemalACancer statistics, 2017CA Cancer J Clin20176773028055103
- JemalABrayFCenterMMFerlayJWardEFormanDGlobal cancer statisticsCA Cancer J Clin201161699021296855
- FunkhouserJReinventing pharma: the theranostic revolutionCurr Drug Discov200221719
- KelkarSSReinekeTMTheranostics: combining imaging and therapyBioconjug Chem2011221879190321830812
- LammersTKiesslingFHenninkWEStormGNanotheranostics and image-guided drug delivery current concepts and future directionMol Pharm201071899191220822168
- XinYHuangQTangJQNanoscale drug delivery for targeted chemotherapyCancer Lett2016379243127235607
- YongKTRoyISwihartMTPrasadPNMultifunctional nanoparticles as biocompatible targeted probes for human cancer diagnosis and therapyJ Mater Chem2009194655467220305738
- BharaliDJMousaSAEmerging nanomedicines for early cancer detection and improved treatment: current perspective and future promisePharmacol Ther201012832433520705093
- RingsdorfHStructure and properties of pharmacologically active polymersJ Polym Sci Symp197551135153
- BarretoJAO’MalleyWKubeilMGrahamBStephanHSpicciaLNanomaterials: applications in cancer imaging and therapyAdv Mater201123H18H4021433100
- HuCMZhangLNanoparticle-based combination therapy toward overcoming drug resistance in cancerBiochem Pharmacol2012831104111122285912
- WolinskyJBColsonYLGrinstaffMWLocal drug delivery strategies for cancer treatment: gels, nanoparticles, polymeric films, rods, and wafersJ Control Release2012159142622154931
- LiechtyWBPeppasNAExpert opinion: responsive polymer nanoparticles in cancer therapyEur J Pharm Biopharm20128024124621888972
- LarsonNGhandehariHPolymeric conjugates for drug deliveryChem Mater20122484085322707853
- WankhedeMBourasAKaluzovaMHadjipanayisCGMagnetic nanoparticles: an emerging technology for malignant brain tumor imaging and therapyExpert Rev Clin Pharmacol2012517318622390560
- MånssonSBjørnerudAPhysical principles of medical imaging by nuclear magnetic resonanceTóthEMerbachAEThe Chemistry of Contrast Agents in Medical Magnetic Resonance ImagingChichester, UKWiley2001144
- YanGPZhuoRXResearch progress of magnetic resonance imaging contrast agentsChin Sci Bulll20014612331237
- WeiHBrunsOTKaulMGExceedingly small iron oxide nanoparticles as positive MRI contrast agentsProc Natl Acad Sci U S A20171142325233028193901
- KhemtongCKessingerCWGaoJPolymeric nanomedicine for cancer MR imaging and drug deliveryChem Commun (Camb)20093497351019521593
- PengYKTsangSCChouPTChemical design of nanoprobes for T1-weighted magnetic resonance imagingMater Today201619336348
- YanGPRobinsonLHoggPMagnetic resonance imaging contrast agents: overview and perspectivesRadiography200713e5e19
- GeraldesCFLaurentSClassification and basic properties of contrast agents for magnetic resonance imagingContrast Media Mol Imaging2009412319156706
- FassLImaging and cancer: a reviewMol Oncol2008211515219383333
- BloembergenNMorganLOProton relaxation times in paramagnetic solutions: effects of electron spin relaxationJ Chem Phys196134842850
- CaravanPStrategies for increasing the sensitivity of gadolinium based MRI contrast agentsChem Soc Rev20063551252316729145
- VillarazaAJBumbABrechbielMWMacromolecules, dendrimers, and nanomaterials in magnetic resonance imaging: the interplay between size, function, and pharmacokineticsChem Rev20101102921295920067234
- AiHLayer-by-layer capsules for magnetic resonance imaging and drug deliveryAdv Drug Deliv Rev20116377278821554908
- SeymourHKoenigKETheory of 1/T1 and 1/T2 NMRD profiles of solutions of magnetic nanoparticlesMagn Reson Med1995342272337476082
- RodneyABrooksFMGillisPOn T2-shortening by weakly magnetized particles: the chemical exchange modelMagn Reson Med2001451014102011378879
- GillisPMoinyFBrooksRAOn T2-shortening by strongly magnetized spheres: a partial refocusing modelMagn Reson Med20024725726311810668
- TongSHouSZhengZZhouJBaoGCoating optimization of superparamagnetic iron oxide nanoparticles for high T2 relaxivityNano Lett2010104607461320939602
- JunYWLeeJHCheonJChemical design of nanoparticle probes for high-performance magnetic resonance imagingAngew Chem Int Ed Engl2008475122513518574805
- SunSZengHRobinsonDBMonodisperse MFe2O4 (M = Fe, Co, Mn) nanoparticlesJ Am Chem Soc200412627327914709092
- LeeJHHuhYMJunYWArtificially engineered magnetic nanoparticles for ultra-sensitive molecular imagingNat Med200713959917187073
- AiHBrentCWeinbergBMagnetite-loaded polymeric micelles as ultrasensitive magnetic-resonance probesAdv Mater20051719491952
- WangZLiuGSunJSelf-assembly of magnetite nanocrystals with amphiphilic polyethylenimine: structures and applications in magnetic resonance imagingJ Nanosci Nanotechnol2009937838519441322
- LiuGWangZLuJLow molecular weight alkyl-polycation wrapped magnetite nanoparticle clusters as MRI probes for stem cell labeling and in vivo imagingBiomaterials20113252853720869767
- LuJMaSSunJManganese ferrite nanoparticle micellar nano-composites as MRI contrast agent for liver imagingBiomaterials2009302919292819230966
- WuCPHsiehCHWuYSThe emergence of drug transporter-mediated multidrug resistance to cancer chemotherapyMol Pharm201181996201121770407
- VinhNQNakaSCabralHMRI-detectable polymeric micelles incorporating platinum anticancer drugs enhance survival in an advanced hepatocellular carcinoma modelInt J Nanomedicine2015104137414726203241
- MaYMouQSunMCancer theranostic nanoparticles self-assembled from amphiphilic small molecules with equilibrium shift-induced renal clearanceTheranostics201661703171627446502
- TongGFangZHuangGGadolinium/DOTA functionalized poly(ethylene glycol)-block-poly(acrylamide-co-acrylonitrile) micelles with synergistically enhanced cellular uptake for cancer theranosticsRSC Adv201665053450542
- AbbasiAZPrasadPCaiPManganese oxide and docetaxel co-loaded fluorescent polymer nanoparticles for dual modal imaging and chemotherapy of breast cancerJ Control Release201520918619625908171
- HaoYZhangBZhengCMultifunctional nanoplatform for enhanced photodynamic cancer therapy and magnetic resonance imagingColloids Surf B Biointerfaces201715138439328029550
- DongZGongHGaoMPolydopamine nanoparticles as a versatile molecular loading platform to enable imaging-guided cancer combination therapyTheranostics201661031104227217836
- AsadiHKhoeeSDeckersRPolymer-grafted superparamagnetic iron oxide nanoparticles as a potential stable system for magnetic resonance imaging and doxorubicin deliveryRSC Adv201668396383972
- LiangPCChenYCChiangCFDoxorubicin-modified magnetic nanoparticles as a drug delivery system for magnetic resonance imaging-monitoring magnet-enhancing tumor chemotherapyInt J Nanomedicine2016112021203727274233
- MalekzadehAMRamazaniARezaeiSJTNiknejadHDesign and construction of multifunctional hyperbranched polymers coated magnetite nanoparticles for both targeting magnetic resonance imaging and cancer therapyJ Colloid Interface Sci2017490647327870961
- FengQZhangYZhangWProgrammed near-infrared light-responsive drug delivery system for combined magnetic tumor-targeting magnetic resonance imaging and chemo-phototherapyActa Biomater20174940241327890732
- RenLLiuXWangQFacile fabrication of a magnetically smart PTX-loaded Cys-Fe3O4/CuS@BSA nano-drug for imaging-guided chemo-photothermal therapyDalton Trans2017462204221328128375
- AnJZhangXGuoQZhaoYWuZLiCGlycopolymer modified magnetic mesoporous silica nanoparticles for MR imaging and targeted drug deliveryColloids Surf A Physicochem Eng Asp201548298108
- DuBHanSLiHMulti-functional liposomes showing radiofrequency-triggered release and magnetic resonance imaging for tumor multi-mechanism therapyNanoscale201575411542625731982
- WangSYangWDuHMultifunctional reduction-responsive SPIO&DOX-loaded PEGylated polymeric lipid vesicles for magnetic resonance imaging-guided drug deliveryNanotechnology20162716510126941226
- YangHKQiMMoLReduction-sensitive amphiphilic dextran derivatives as theranostic nanocarriers for chemotherapy and MR imagingRSC Adv20166114519114531
- LiYJDongMKongFMZhouJPFolate-decorated anticancer drug and magnetic nanoparticles encapsulated polymeric carrier for liver cancer therapeuticsInt J Pharm2015489839025888801
- MosaferJAbnousKTafaghodiMMokhtarzadehARamezaniMIn vitro and in vivo evaluation of anti-nucleolin-targeted magnetic PLGA nanoparticles loaded with doxorubicin as a theranostic agent for enhanced targeted cancer imaging and therapyEur J Pharm Biopharm2017113607428012991
- JaidevLRChellappanDRBhavsarDVMulti-functional nanoparticles as theranostic agents for the treatment and imaging of pancreatic cancerActa Biomater20174942243327890622
- SituJQWangXJZhuXLMultifunctional SPIO/DOX-loaded A54 homing peptide functionalized dextran-g-PLGA micelles for tumor therapy and MR imagingSci Rep201663591027775017
- LinBSuHJinRMultifunctional dextran micelles as drug delivery carriers and magnetic resonance imaging probesSci Bull20156012721280
- DuBHanSZhaoFA smart upconversion-based light-triggered polymer for synergetic chemo-photodynamic therapy and dual-modal MR/UCL imagingNanomedicine2016122071208027184094
- AsemHZhaoYYeFBiodistribution of biodegradable polymeric nanocarriers loaded with busulphan and designed for multimodal imagingJ Nanobiotechnol20161482
- LeeSYYangCYPengCLA theranostic micelleplex Co-delivering Sn-38 and VEGF siRNA for colorectal cancer therapyBiomaterials2016869210526896610
- WuLWuMLinXZhangXLiuXLiuJMagnetite nanocluster and paclitaxel-loaded charge-switchable nanohybrids for MR imaging and chemotherapyJ Mater Chem B20175849857
- HayashiKSatoYSakamotoWYogoTTheranostic nanoparticles for MRI-guided thermochemotherapy: “tight” clustering of magnetic nanoparticles boosts relaxivity and heat-generation powerACS Biomater Sci Eng2017395105
- WangXChangYZhangDTianBYangYWeiFTransferrin-conjugated drug/dye-co-encapsulated magnetic nanocarriers for active-targeting fluorescent/magnetic resonance imaging and anti-tumor effects in human brain tumor cellsRSC Adv20166105661105675
- ThoratNDLemineOMBoharaRAOmriKel MirLTofailSASuperparamagnetic iron oxide nanocargoes for combined cancer thermotherapy and MRI applicationsPhys Chem Chem Phys201618213312133927427175
- KangJHToitaRKatayamaYBio and nanotechnological strategies for tumor-targeted gene therapyBiotechnol Adv20102875776320541598
- CaoSCrippsAWeiMQNew strategies for cancer gene therapy: progress and opportunitiesClin Exp Pharmacol Physiol20103710811419671071
- CondeJArnoldCETianFArtziNRNAi nanomaterials targeting immune cells as an anti-tumor therapy: the missing link in cancer treatmentMater Today2016192943
- BoussifOLezoualc’hFZantaMAA versatile vector for gene and oligonucleotide transfer into cells in culture and in vivo polyethylenimineProc Natl Acad Sci U S A199592729773017638184
- BonettaLThe inside scoop-evaluating gene delivery methodsNat Methods20052878881
- ThomasMKlibanovAMEnhancing polyethylenimine’s delivery of plasmid DNA into mammalian cellsProc Natl Acad Sci U S A200299146401464512403826
- GaoXWangGShiTBiodegradable gadolinium-chelated cationic poly(urethane amide) copolymers for gene transfection and magnetic resonance imagingMater Sci Eng C Mater Biol Appl20166518118727157741
- ParkJKJungJSubramaniamPGraphite-coated magnetic nanoparticles as multimodal imaging probes and cooperative therapeutic agents for tumor cellsSmall201171647165221560243
- BayazitogluYKheradmandSTulliusTKAn overview of nanoparticle assisted laser therapyInt J Heat Mass Transf201367469486
- CaoMWangPKouYGadolinium (III)-chelated silica nanospheres integrating chemotherapy and photothermal therapy for cancer treatment and magnetic resonance imagingACS Appl Mater Interfaces20157250142502326418578
- MiPDewiNYanagieHHybrid calcium phosphate-polymeric micelles incorporating gadolinium chelates for imaging-guided gadolinium neutron capture tumor therapyACS Nano201595913592126033034
- ChenYAiKLiuJRenXJiangCLuLPolydopamine-based coordination nanocomplex for T1/T2 dual mode magnetic resonance imaging-guided chemo-photothermal synergistic therapyBiomaterials20167719820626606445
- ChenJLiMYiXSynergistic effect of thermo-radiotherapy using Au@FeS core-shell nanoparticles as multifunctional therapeutic nanoagentsPart Part Syst Charact2017341600330
- ShahBPPasqualeNDeGTanTMaJLeeKBCore-shell nanoparticle-based peptide therapeutics and combined hyperthermia for enhanced cancer cell apoptosisACS Nano201489379938725133971
- LiuYGuoQZhuXOptimization of Prussian blue coated NaDyF4:x%Lu nanocomposites for multifunctional imaging-guided photothermal therapyAdv Funct Mater20162651205130
- YuanWYangDSuQIntraperitoneal administration of biointerface-camouflaged upconversion nanoparticles for contrast enhanced imaging of pancreatic cancerAdv Funct Mater20162686318642
- LiYGuYYuanWCore–shell−shell NaYbF4:Tm@CaF2@NaDyF4 nanocomposites for upconversion/T2-weighted MRI/computed tomography lymphatic imagingACS Appl Mater Interfaces20168192081921627366965
- LiuYZhangGGuoQArtificially controlled degradable inorganic nanomaterial for cancer theranosticsBiomaterials201711220421727768974
- LiuYFanHGuoQJiangADuXZhouJUltra-small pH-responsive Nd-doped NaDyF4 nanoagents for enhanced cancer theranostic by in situ aggregationTheranostics2017742184228
- DemirerGSOkurbACKizilelSSynthesis and design of biologically inspired biocompatible iron oxide nanoparticles for biomedical applicationsJ Mater Chem B2015378317849
- ThoratNDBoharaRANoorMRDhamechaDSoulimaneTTofailSAEffective cancer theranostics with polymer encapsulated superparamagnetic nanoparticles: combined effects of magnetic hyperthermia and controlled drug releaseACS Biomater Sci Eng2017313321340
- BoniABasiniAMCapolupoLOptimized PAMAM coated magnetic nanoparticles for simultaneous hyperthermic treatment and contrast enhanced MRI diagnosisRSC Adv201774410444111
- HatamieSAhadianaMMGhiassaMAGraphene/cobalt nanocarrier for hyperthermia therapy and MRI diagnosisColloids Surf B Biointerfaces201614627127927351138
- LiuXLNgCTChandrasekharanPSynthesis of ferromagnetic Fe0.6Mn0.4O nanoflowers as a new class of magnetic theranostic platform for in vivo T1-T2 dual-mode magnetic resonance imaging and magnetic hyperthermia therapyAdv Healthc Mater201652092210427297640
- HerynekVTurnovcováKVeverkaPUsing ferromagnetic nanoparticles with low Curie temperature for magnetic resonance imaging-guided thermoablationInt J Nanomedicine2016113801381127540292
- AggarwalAHughesSPalliative radiotherapy: evolving role and policy challengesJ Cancer Policy2016102129
- LiMZhaoQYiXAu@MnS@ZnS core/shell/shell nanoparticles for magnetic resonance imaging and enhanced cancer radiation therapyACS Appl Mater Interfaces201689557956427039932
- DouYLiXYangWPb@Au core–satellite multifunctional nanotheranostics for magnetic resonance and computed tomography imaging in vivo and synergetic photothermal and radiosensitive therapyACS Appl Mater Interfaces201791263127228029033
- DetappeAThomasETibbittMWUltrasmall silica-based bismuth gadolinium nanoparticles for dual magnetic resonance–computed tomography image guided radiation therapyNano Lett2017171733174028145723