Abstract
Conventional tissue engineering, cell therapy, and current medical approaches were shown to be successful in reducing mortality rate and complications caused by cardiovascular diseases (CVDs). But still they have many limitations to fully manage CVDs due to complex composition of native myocardium and microvascularization. Fabrication of fully functional construct to replace infarcted area or regeneration of progenitor cells is important to address CVDs burden. Three-dimensional (3D) printed scaffolds and 3D bioprinting technique have potential to develop fully functional heart construct that can integrate with native tissues rapidly. In this review, we presented an overview of 3D printed approaches for cardiac tissue engineering, and advances in 3D bioprinting of cardiac construct and models. We also discussed role of immune modulation to promote tissue regeneration.
Introduction
Cardiovascular diseases (CVDs) are highly prevailing worldwide diseases in terms of morbidity and mortality worldwide, especially in developed countries.Citation1 One out of seven deaths is caused by coronary artery disease and the estimated total incidences of myocardial infarction are almost 790,000 per year.Citation1–Citation3 Other than loss of native cells in heart tissues, the different pathologic conditions such as atresia, regurgitation, and stenosis also affect the heart valves.Citation4–Citation6 Cardiac arrest or heart attack is caused by problem in electrical impulse or blood arteries that supply oxygen and nutrients to heart tissues.Citation7 Almost 1 billion cardiomyocytes (CMs) are lost during heart attack.Citation8 There is no auto-regeneration or repair process for lost cells, rather the heart forms a scar tissue that blocks the electrical signal and spontaneous contraction. This situation increases the risk of heart failure. Acute myocardial infarction (MI) or end-stage ischemic heart failure is currently managed by cell therapy, coronary artery bypass grafting, left ventricular assist device, and finally heart transplantation.Citation9–Citation12 But there is lack of organ donors as compared to demand and also organ transplantation is not always successful due to associated complications of immune rejection.Citation13 Among other treatment options available, direct injection of cells to heal MI showed some success to regain muscle function; however, most of the cells after injection were reported to be deadCitation14–Citation16 (). These injected cells also failed to develop early vascularization and extracellular matrix (ECM), which is important for exchange of materials in tissue repair process. Due to lack of three-dimensional (3D) flexible biomimetic ECM and the supply of nutrients, the survival duration of the injected cells is low. Therefore, 90% cells die as a result of lack of nutrients supply and exposure to free radicals and inflammatory cytokines present in the surrounding tissueCitation17,Citation18 (). In cell therapy, bone marrow stem cells are isolated from bone marrow based on density gradient centrifugation.Citation19 The resulting product is defined as bone marrow mononuclear cells (BMMNCs), which include bone marrow hematopoietic stem cells, bone marrow mesenchymal stem cells, and committed cells in the various stages of differentiation. The majority of the clinical trials with BMMNCs reported a modest improvement of left ventricular function and cardiac perfusion by enhanced microvascularization.Citation14,Citation20–Citation22 Tissue engineering of a large-size cardiac construct is very challenging due to its complex nature (). It is tough to mimic the hierarchical property of native myocardium to develop dynamic cardiac tissue with continuous contraction and relaxation.Citation23,Citation24 Cardiac tissues are composed of ECM proteins and different cells aligned in a very special order to maintain their spontaneous contraction to pump blood. Keeping in view these challenges there is need of a special tissue engineering technique that can present a scaffold with natural ECM and can hold the CMs and promote their regeneration.
Figure 1 Cell therapy to treat MI, isolation, injection, repair, and apoptosis.
Abbreviations: HGF, human growth factor; MI, myocardial infarction; MSC, mesenchymal stem cell; VEGF, vascular endothelial growth factor.
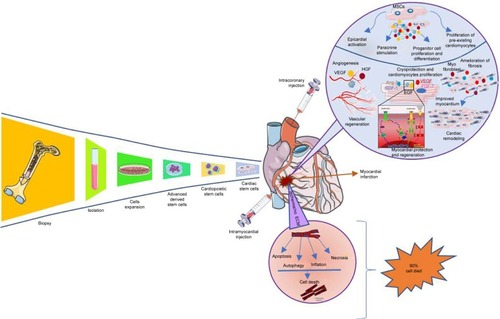
Tissue engineering is a multidisciplinary approach, which combines materials engineering, life sciences, and computer modeling to produce functional scaffolds and artificial tissues construct for biomedical applications.Citation17,Citation25,Citation26 Tissue engineering has potential to address all key challenges of cardiac tissue engineering.Citation27 In this technique a scaffold with ECM nature was used to support repair or regeneration process. Tissue engineering techniques used mainly three types of materials for cardiac tissue engineering, and those are natural (gelatin, collagen, alginate, chitosan, fibrin, and hyaluronic acid),Citation28–Citation30 synthetics [poly (glycolic acid), polycaprolactone (PCL), poly (lactic acid), and poly D,L-lactic-co-glycolic acid (PLGA)],Citation28,Citation31–Citation33 and hybrid (combination of synthetics, natural, and metallic).Citation34 These materials are used to fabricate 3D scaffolds to mimic the native ECM and present similar morphology to cell as they exit from the original organ. An ideal scaffold for cardiac tissue engineering should be biocompatible, biodegradable, and have enough mechanical strength and electrical conductance to perform dynamic functions of the heart.Citation35,Citation36
Over a course of time, 3D scaffold-based cell culture technique is proven better as compared to 2D conventional cell culture techniques, commonly used in academic and industrial labs.Citation37,Citation38 In 2D cell culture, cellular morphology is different from native tissue due to extra surface tension and one side cell adhesion in cell culture flask or dish.Citation39 Also, monolayer cell culture or single-cell culture cannot sufficiently explain the clinically relevant heart disease models like MI and heart failure.Citation40 Furthermore, culture of CMs within 3D scaffold showed CM alignment and physiology.Citation41 Embryonic stem cell (ESC)-derived CMs showed increased expression for contractile functionally related genes, extended sarcomeres, and higher conduction velocities within 3D scaffolds as compared to 2D monolayers.Citation42 Therefore, 3D tissue engineering strategies can only help in finding the optimum solution for cardiac tissue engineering. Various techniques and designs have been applied to manipulate cell physiology by targeting substrate, topography, material stimulation by electrical or mechanical signal, and coculture.Citation43 In 3D tissue engineering techniques, we can use mechanical strain and shear stress, alter calcium dynamics, electric impulse and temperature can simultaneously direct cell alignment, synchronization, differentiation, and maturations of cells to CMs.Citation37,Citation44 Further, 3D scaffolds can support coculture or mixture of cell culture, as cardiac tissue engineering required different cells to mimic functions of myocardium. Additionally, it has been observed that CMs culture with non-CM cells supported the regeneration and growth of tissue-engineered construct.Citation45 In some cases, only CMs failed to develop tissue construct when they were cultured alone, as endothelial cells (ECs) are important for vascularization of constructs to match nutrients demand. Coculture of CMs and ECs in 3D scaffolds resulted in functionalized cardiac tissue construct with increased proliferation, amelioration, physiology, and viability of CMs.Citation46 These functional cardiac constructs as a result of 3D scaffold-based tissue engineering can also act as an alternative for in vivo drug screening as existing animal models of drug screening does not match exactly with human physiology.Citation47 Furthermore, human immune system is much complex as compared to animals’ use as disease models for drug screening. Therefore, during drug development when drug is screened through 2D cell culture assays and animals’ disease models, screening results are prone to false positive or false negative. In the past decade, more than 20% drugs are withdrawn from market due to cardiac cytotoxicity including antihistamines (terfenadine and astemizole), benfluorex, sibutramine, prokinetic agents (cisapride), spasmolytics (terodiline), dexfenfluramine and pergolide, antipsychotics (thioridazine), and quinolone antibiotics (grepafloxacine).Citation47,Citation48 Among 3D scaffold fabrication techniques, hydrogels, nanofibers, and decellularization have been widely used for tissue engineering of different organsCitation49–Citation57 (). But these techniques are successful for only avascular organs; however, development of artificial construct for highly vascularized organ like heart is very challenging.Citation58 Branched blood arteries and capillaries offer additional complexity to fabricate functional cardiovascular organoids. Second challenge in engineering cardiac organoids is CM’s limited self-renewal ability. Also, fabricating aligned and thick cardiovascular tissue is complicated as it requires microvascular network for exchange of materials and oxygen supply. Additionally, synchronization of synthesized tissue constructs with native tissue and execution of spontaneous contraction of construct is itself a challengeCitation24,Citation59 (). Due to these challenges, until now, idea of fully functional heart construction by using only conventional tissue engineering technique has not been achieved yet. Recently, 3D printing technique provided an alternative to make this possible, as 3D printing technique can develop a heterogeneous 3D scaffold with strong mechanical strength and with all required characteristics of an ideal scaffold for cardiac tissue engineering.Citation44,Citation60 With the advancement of 3D printing, scaffolds can now be fabricated with native ECM morphology and accuracy.Citation61 AutoCAD (computer-aided design) has made it possible to design whole 3D structure like an organ and then print it with biocompatible materials to get a 3D scaffold of organ shape.Citation62 To develop an artificial organ or muscle patch, this scaffold must be seeded with tissue-specific cells.Citation61,Citation63 This technique enables us to use conventional 3D scaffolds (hydrogels) mix with cells (bio ink) and perform precise 3D bioprinting for organs by using different approachesCitation63–Citation66 (, ). In most recent strategies of 3D printing, organs are being printed directly by using spheroid without biomaterial scaffold involvement to avoid immune reaction.Citation37,Citation67,Citation68 In this review, we discussed different 3D printed approaches for cardiac tissue engineering, microfluidics-based heart-on-a-chip technologies, scaffold free 3D bioprinting to develop an in vitro cardiac model for drug screening,Citation69 and immune modulations for cardiac tissue engineering.
Table 1 Scaffolds/materials used in 3D printing of cardiac and microvascular structures
Figure 3 Comparison of conventional and modern 3D printed scaffold-based tissue engineering techniques: (A) decellularization, (B) hydrogels, (C) nanofibers, (D) spheroids and hydrogel hybrid bioprinting, (E) 3D scaffold printing, and (F) 3D printed microfluidics chip.
Abbreviation: 3D, three-dimensional.
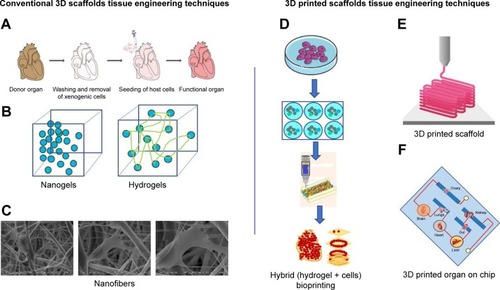
Figure 4 Process of 3D bioprinting, (A) steps of 3D bioprinting, (B) pre-scaffold fabrication bioprinting, (C) simultaneous hybrid 3D bioprinting.
Abbreviations: 3D, three-dimensional; CAD, computer-aided design; PCL, polycaprolactone; hdECM, heart decellularized-extracellular matrix; cdECM, cartilage decellularized-extracellular matrix; adECM, adipose decellularized-extracellular matrix.
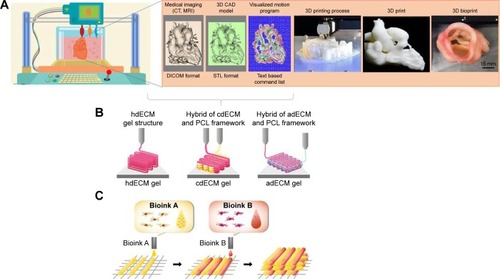
3D printing cardiac tissue engineering
3D printing is a technique in which materials are combined in 3D shape through computer-controlled process.Citation63 There are different methods to complete this 3D printing process based on technology, cost, speed, resolution, and limitationsCitation70 (). In case of 3D bioprinting, only a few of them have been used, primarily due to the restrictions made when working with biologic materials.Citation71 3D printing processes have two main categories: scaffold-based printing and scaffold-free printing.Citation72 Various approaches have been used for scaffold-based bioprinting: first fabrication of 3D scaffold by biomaterial(s) and the cell seeding; preprinting of scaffold and then cell layers printing or simultaneous printing of biomaterials and cells. In second scaffold-free category, bioink consists of individual cells or tissue spheroids are used for direct printing on substrate.Citation73 3D printing-based applications of tissue engineering in combination with stem cell technology have the potential to address the shortage of donor organs for transplantation and provide patient-specific tissue replacement. However, tissue vascularization and rapid vascular integration in vivo remain as two pervasive and long-standing obstacles. Here we will discuss few modern 3D printing approaches for cardiac tissue engineering.
Figure 5 3D bioprinting technology and its types.
Abbreviations: 3D, three-dimensional; UV, ultraviolet.
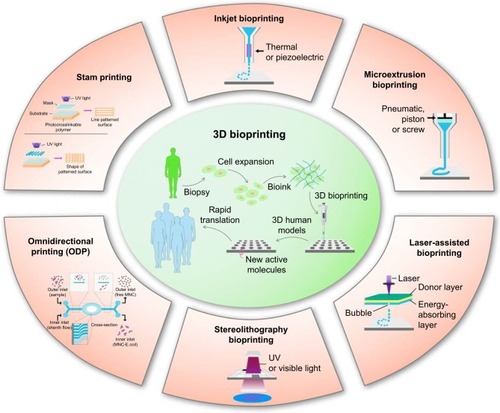
3D printed scaffold tissue engineering
One of the essential traits of an ideal scaffold is that it should have native ECM texture to support repair and regeneration process. 3D printing has offered a precise control to fabricate scaffold with native ECM morphology and through this it has prompted a tremendous excitement in tissue engineering.Citation61 3D printed scaffolds have defined geometric dimensions with fine 3D groove pattern composed of hybrid and heterogeneous materials to mimic the natural ECM morphology. Surface of 3D scaffold can also be patterned with channels to mimic additional function of endothelial network.Citation74 These 3D patterns, grooves, and channels are key characteristics and they influence cell’s migration, morphology, phenotype, and physiology. This phenomenon is called as topologic or surface guidance and it is important for stem cell differentiation into adipogenic, osteogenic, neurogenic, myogenic, and cardiomyogenic phenotype.Citation75 To get control over cell physiology and limit their differentiation, composition and pattern of ECM are important to know and then replicate those key features with computer-aided software and design 3D printed scaffold with fine resolution. Until now a fine resolution of ≤1 µm has been achieved to construct adjacent narrow channels.Citation64,Citation76 These narrow patterns also influence cell adhesions. For example in a recent study, a 3D printed scaffold seeded with CMs demonstrated synchronized movement just after 1 day of cell culture, which shows that individual cell adhesion with scaffold inside the microchannels was quick and interchannel coupling was also established quickly.Citation60 Another study was conducted by using a novel multiphoton-excited 3D printing technique to print ECM-based scaffold with high resolution. They cultured 5×104 cells composed of human induced pluripotent cell-derived cardiomyocytes (hiPSC-CMs), smooth muscle cells (SMCs), and ECs (in a 2:1:1 ratio) over this 3D scaffold to develop human cardiomyocyte patches (hCMP). The hCMP appeared soon after 24 hours of cell seeding and expressed the cardiac protein markers, calcium transients, and synchronized natural heart beating. The authors also used this scaffold to treat animal model of MI, and scaffold-free hCMP were used as control. This 3D printed scaffold-based hCMP showed improvement in heart physiology, infarct size, cell viability, endothelial density, and cell proliferation as compared to control.Citation77 Recently, PCL and PCL carbon nanotube were used to develop 3D printed composite scaffolds and test its biocompatibility to support cardiac tissue engineering.Citation78 Similarly, PLGA were used to construct 3D scaffold for tissue engineering applicationsCitation79 ().
In a latest technique of 3D scaffold-based printing, a bioink composed of cells and desired biomaterials is directly used to print a scaffold in the shape of organ to develop full or partial organ (). Recently, Zhang et al used the composite bioink consisting of ECs and microfibrous hydrogel in 3D printing to fabricate myocardium. During direct 3D printing, gravity and difference in density helped ECs to align themselves along the periphery of microfibers and form a symmetrical layer of confluent endothelium. Further, endothelium was overlayered by CMs with controlled anisotropy to develop a functional myocardium. This myocardium expressed the rhythmic beating. The authors also used this myocardium model with microfluidics-based perfusion bioreactor to develop endothelialized-myocardium-on-a-chip model for drug screening. It has shown that CMs derived from iPSCs can be used for developing endothelialized human myocardium.Citation64 Recently the 3D sacrificial molding-based 3D bioprinting was introduced, and in this technique a rigid 3D lattice of sugar filaments was embedded with cells patterned over hydrogels. On contact with aqueous phase, sugar filaments subsequently dissolved and formed a microchannel network to support microvascularization. Though this is a simple method to introduce hallow channel network in hydrogels, hydrogel does not support lumen network when extensive remodeling of ECM happened with cell seeding. For instance, macroscopic, tissue contraction of heart muscle would be virtually impossible to establish without tight cell–cell connections at physiologic cell density.Citation80 In another similar study, microchannels were fabricated in a 3D printed gelatin hydrogel cross-linked with an enzyme mTgase. These microchannels were used to align hMSCs and increase their differentiation into CMs. These microchannels also increased the CM functionality and orientation.Citation81 To 3D print fully functional cardiac tissue, a hybrid hydrogel system composed of sacrificial hydrogel, cell-laden hydrogel, and PCL was developed. They used these hydrogels along with 10×106 cells/mL as a bioink to print a 3D cardiac construct at 18°C. Separate dispensing modules were used for each type of hydrogel and PCL for 3D printing. Thus, the obtained cardiac tissue constructs showed a spontaneous synchronized beating in culture, showing potential for in vitro cardiac tissue development and maturation. Fully functional cardiac tissue development was confirmed by immunostaining of α-actinin and connexin 43. They also tested these bioprinted cardiac tissues for in vitro drug screening studies and their response was phenomenal and comparable to native cardiac tissues.Citation44 Similarly, albumin electrospun nanofibers were 3D laser patterned to develop grooves on its surface to align CMs. The authors seeded these patterned groves with ECs to form closed lumens in another layer of 3D patterned nanofiber. They also fabricated 3D cage-like pattern to attach PLGA microparticulate systems for sustained release of VEGF to promote vascularization, or dexamethasone, an anti-inflammatory agent. They grew these layers separately until they glued them with ECM-based biologic glue to form thick 3D cardiac patches and implanted them in rat and their coupling was observed.Citation82 Maiullari et al used a bioink composed of alginate and PEG-fibrinogen (PF) hydrogel and human umbilical vein endothelial cells (HUVECs) and induced pluripotent cell-derived cardiomyocytes (iPSC-CMs) to develop functional construct in hydrogel strands produced through 3D printed microfluidics channels. They developed a functional cardiac construct with a high orientation index imposed by the different defined geometries and blood vessel-like shapes generated by HUVECs. They also demonstrated its in vivo grafting and integration of the engineered cardiac tissue with host’s vasculature.Citation83 All these scaffold-based 3D printed heart constructs’ success depends on their electrical coupling with the native cardiac tissues, and in the future such 3D printed scaffold should have electrical conductance properties to facilitate the cell bundle extension to establish that connection.
Cell types
There are various types of cell used in 3D printed scaffolds or 3D bioprinting for cardiac tissue engineering. Mainly they are classified as nonhuman cells and human cells. They are either used as in single form or in mixture form. Rat myoblast cells have been shown to differentiate into functioning cardiac tissue in a variety of experiments.Citation84 Among human cells, so far bone marrow–or adipose-derived stem cells, ESCs, and skeletal myoblast and resident cardiac stem cells are commonly used for cardiac tissue engineering application.Citation12,Citation32,Citation42,Citation85–Citation87 With the advancement of stem cell biology, progenitor cells of different sources have also been now used in regenerative medicine for cardiac repair. Regenerative medicine and stem cell engineering are emerging as a promising solution to treat CVDs.Citation88–Citation90 ESCs are considered ideal for cardiac tissue engineering prior to their pluripotency and self-renewal. They are isolated from human blastocyst and cultured in vitro for the first time in 1998.Citation91 Stem cell advancement resolved this issue of cell demand by generating iPSCs from somatic cells of patients. These cells can generate unlimited numbers of different types of cells including functional CMs just like ESCs. Somatic cells are reprogrammed into iPSCs through addition of transcription factors kfl-4, Myc c, Oct 4, and Sox 2.Citation92 When these iPSCs are further provided with transcription factors Flk1, Isl1, or Nkx2.5 they differentiate into cardiac progenitor cells, which are further used in cardiac tissue engineering.Citation93 Furthermore, ESCs and iPSCs can also be differentiated into CMs and vascular cells through Wnt/Catenin signaling pathway. Wnt/Catenin signaling pathway can be activated by blocking glycogen synthase kinase 3 before the differentiation of ESCs and iPSCs.Citation94,Citation95 As these iPSCs will be derived from the somatic cells of the patient to be treated, they do not face immune problems. Thus, iPSCs are considered an important source to produce the autologous CMs needed to develop synthetic cardiac tissue construct.Citation36,Citation96,Citation97 There are different protocols that have been developed to differentiate ESCs and iPSCs into CMs and are widely applied in tissue engineering to repair MI. However, immaturity of stem cell-derived CMs, due to incomplete maturation,Citation98 remains a major obstacle, and promoting CM maturation is important in order to achieve the final goal of cardiac regeneration.Citation99 Chong et al observed in a nonhuman primate model of myocardial ischemia-reperfusion that treatment with human embryonic stem cell–derived cardiomyocytes (hESC-CMs) led to significant remuscularization, albeit with nonfatal ventricular arrhythmias, due to incomplete maturation of hESC-CMs.Citation100 Recently mouse somatic cells were programmed into pluripotent stem cells and further differentiated into electrophysiologic functional mature CMs expressing cardiac markers with the potential to treat MI. In terms of human cells,Citation101 hCMPCs and hiPSC-CMs are popular choices for 3D bioprinting.Citation102–Citation104 These cells demonstrated genetic profiles and protein expression of native myocardium when bioprinted in the methods described above.
Microfluidics-based 3D cardiac tissue engineering
As discussed previously, one of the vital barriers in heart tissue engineering is the supply of oxygen and nutrients to thick cardiac tissue (>100–200 µm) (). Therefore, developing a perusable microvascular network, which mimics the natural vascular network of arteries, is a fundamental requirement to treat ischemic diseases. Previously, efforts were made to develop microvascular structures by stimulation of angiogenesis in vivo, by implantation of ECs, or by re-endothelialization of decellularized organs (). But all these previous methods have shown their own limitations. Most recent development to resolve this issue is microfluidics devices, which mimic the complex microvascular tissue engineering and demonstrated the physiologic function of heart on the chip.Citation64 Microfluidics devices involve microfabrication of the device through computer-aided designing, and electrical and mechanical control of fluid controls with 3D coating of biomaterials.Citation105 Microfluidics devices like organ-on-a-chip and lab-on-a-chip could be a potential technique to implement key features of functional tissue units at the microscale and nanoscale levels. These systems presented the platform to observe a real-time effect of biochemical, mechanical, and electrical stimulations on new heart tissue constructs, which are key factors to improve tissue functions.Citation25 As the functions of cardiac muscles are mainly determined by the 3D arrangement of their muscles’ fibers and their perfect contractions in response to electrical impulse, microfluidics devices are one such approach to mimic such complicated arrangements of cardiac tissues in vitro to study the pathophysiologic nature of CMs and drug screening for cardiac toxicity evaluation.
A group of scientists used the microfluidics-based system to study the physiology of cardiac ventricle contractions under physical and electrical stimulation. To mimic the laminar anisotropic nature of cardiac ventricle wall, they fabricated 2D muscular thin films (MTFs), engineered by culturing anisotropic muscular tissue on top of fibronectin-patterned flexible elastomeric cantilevers. They monitored the contractile pattern of MTFs and compared it with sarcomere organization of the cardiac ventricle wall. They concluded that a high degree of 2D arrangements results in higher systolic and diastolic status. In addition to this, they controlled the fluid flow through a platinum pacemaker to analyze more thoroughly contractility tests and study MTF response to electrical impulse. Further, they also used their system for drug screening applications. They successfully demonstrated that CMs can produce relevant contractile forces in measurable range when cells are grown and molded in a 2D structure and under electrical impulse.Citation106 Similarly, Kitamori group demonstrated artificial heart beating on chip through microfluidics by developing a bio-micro-actuator cultured with CMs to bend polydimethylsiloxane (PDMS) micropillars. They also developed a heart-on-a-chip pump, by using mechanical forces produced by CMs that aligned the cell sheet to pump fluids through microfluidic channels.Citation107
To mimic the physiologic functions and protein expression of adult heart tissues, Sheehy et al fabricated an in vitro model of heart-on-the-chip. They seeded this chip with CMs and they showed that anisotropic engineered myocardium expressed a similar degree of global sarcomere alignment, contractile stress output, and inotropic concentration response to the adrenergic agonist isoproterenol. This engineered myocardium also expressed the myofibril-related gene expression similar to muscle fibers isolated from the ventricular tissue of adult rat.Citation108
In vitro 3D microfluidics functional heart models for drug screening
The common strategy to treat CVDs is to use therapeutic interventions that mitigate symptoms; however, these treatments are not sufficient for full recovery and patients are left only with transplant option. To improve medical care options, development of new drugs must be considered. Drug discovery is a complex and time-consuming process involving significant basic research and preclinical evaluation before testing in patients for drug cytotoxicity and clinical efficacy.Citation109 In conventional drug screening approaches, to evaluate a drug for its ADME (absorption, distribution, metabolism, elimination) and cytotoxicity, animal models are being used as disease models (). Preclinical studies conducted on animal models often fail in human trials and lead to huge economic loss along with adverse effect on human lives. Drug-induced cardiotoxicity is one of the major issues for drug industry working on new therapeutics research for CVDs.Citation110 Over the last 15 years, almost 20% of drugs were called back from market due to their cardiotoxicity issues. In addition to them, many of the drugs showed unwanted lethal effects during clinical trials after they passed animals trials. Approximate cost for one drug to pass through clinical trials is around 3–4 billion USD. This indicates that the failure of one drug causes a huge economic loss to drug manufacturing company or funding agency. In the last two decades, almost 50 drugs have been called back from market due to cardiotoxicity.
Figure 6 Conventional vs 3D microfluidics-based drug screening model for cardiotoxicity testing.
Abbreviation: 3D, three-dimensional.
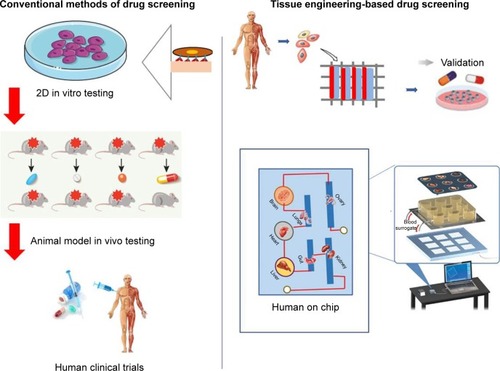
The main reason behind this huge economic loss and time loss is the lack of proper in vitro and in vivo drug testing assays to evaluate drug toxicity, which mimics the exact microenvironment with natural human body environment. Animal-based in vivo models have many ethical concerns, require time-consuming procedures, are expensive, and require sophisticated animal housing. Most important results with animal models are not reliable because physiology of human organ and its chemical interaction with the human body is different from the animal’s body. Though 2D-based static cell culture dishes used for in vitro drug screening assays are physiologically relevant, modeling vascular system specifically for primary perfusion and pharmacokinetic interface as ECs cultured in the absence of hemodynamic stresses (stretch, shear, and pressure) does not mimic the natural physiology of organ or tissues. Therefore, such assay models are not able to predict natural human body response to the drugs.
Advancement in biomedical engineering has presented a new 3D microfluidic-based approach as an alternative in vitro model to resolve this complex issue of drug screening (). In addition, integration of bioengineering technologies with 3D printing technologies further extended this microfluidics platform to develop heart-on-a-chip model to mimic the biology and physiology of fully functional heart. Moreover, to replace human clinical trial, a further extended platform with assemblies of different organs on chip to demonstrate human-on-a-chip provides a system to screen drugs, and its mechanism of actions and evaluate its ADME with respect to mutual interactions of different organs. As these platforms are seeded with the human cells in ECM like natural 3D microenvironment, their results are more reliable for human applications. Microfluidics devices that demonstrate the heart model integrated with endothelial and vascular system can predict drug cardiotoxicity better than animal model. Recently, to depict such a system, cardiopatch 3D culture platform was developed to evaluate drug and disease modeling by using hiPSC-CMs. They developed this system to mimic highly mature and functional human heart (cardiopatch) with clinically active surface area of 4 × 4 cm. They set a free-floating dynamic culture with continuous nutrients supply and exchange of waste materials, and maintain cell seeding density to reflect highly accurate in vitro cardiac maturation model system. After 5 days of culture, hiPSC-CMs showed uniform sarcomere alignments showing I-bands, H-zone, and sketch of T-tubules and M bands. The cardiopatch showed spontaneous contractions, robust electromechanical coupling, and increased expression of maturation markers comparable to adult myocardium. This system can be scaled up to clinical dimensions for drug screening while preserving spatially uniform properties with high conduction velocities and contractile stresses.Citation8 In another study, researcher used PDMS-based microfluidics system to evaluate electrical and mechanical properties of heart construct. They called this system I-Wire heart-on-a-chip. In this system, I-wire assay provided them with an ability to apply control over the electrical and mechanical stimulus to 3D embryonic cardiac constructs (ECTCs) and measure their impact on the constructs. A calibrated flexible probe provides strain load to the ECTC via lateral displacement, and the microscope noticed the deflections of both the probe and the ECTC.Citation60 This system helped to study the behavior of cardiac tissues under controlled mechanical and electrical stimulus. Similarly, Marsanso et al used synchronized stimulus and translated the uniaxial cyclic strain in a 3D microfluidics model to mimic the mechanical stimulus faced by CMs in the native myocardium. Their device had an array of hanging posts, which restrict cell-laden gels and a pneumatic actuation system to produce uniaxial strains on the microengineered cardiac tissues (µECTs). They evaluated the effect of physiologic cyclic uniaxial strains (10%–15%) on the maturation and physiology of uniformly arranged µECTs developed by seeding neonatal rat or hiPSC-CMs using this device. They showed that cyclic uniaxial strain induced the maturation as well as improved the contraction and synchronized beating. They also tested the human µECTs for drug screening in physiologic environment. These tissues responded well to isoprenaline (β-adrenergic stimulation) at low concentrations (1 nM) with positive chronotropic effect when compared to the previously reported response concentration limit of 100 nM and 1 µM in large- and microscale human embryonic cells, respectively. This beating analysis of hiPSC-CM constructs under controlled administration of isoprenaline showed its potential applications for drug development and cardiotoxicity studies. Indeed, the ability to generate reproducible and functional cardiac microtissues from hiPSC-CMs broadens the application horizons of this device, with the possibility to generate patient-specific myocardium-like tissues, which can model unique and relevant genetic cardiac disorders.Citation111
One of the main problems with cardiac tissue engineering using a 3D scaffold is building few millimeters thick cardiac tissue and supply of oxygen to CMs. Zhang et al reported a 3D microfluidics scaffold called AngioChip, which has extensive network of nanopore and microholes in the microfluidics channels to enhance their perfusions and intercellular communications. This AngioChip supports the assembly of parenchymal cells on a mechanically stable matrix surrounding 3D microfluidics network coated with ECs. They also demonstrated the vascularized cardiac tissues engineered using AngioChips to show drug delivery pattern clinically relevant to intravenous injection and presented that few millimeter-thick cardiac tissues can be developed in a scalable manner. Moreover, they implanted AngioChip cardiac tissues to rat hindlimb femoral vessel by direct surgical anastomosis to restore its blood perfusion.Citation112
To present the microfluidics system as a heart disease model, Horton et al presented cardiac dysfunction model by using angiotensin II (ANG II). This heart-on-a-chip model composed of arrays of MTFs can help to differentiate the healthy and abnormal tissues by tracking the deflection of thin films that results from contraction. The ANG II was used to elicit pathologic responses and they measured the cardiac function by expression of cardiac markers, calcium transients, morphologic changes, and contractile stress after and before administration of ANG II. They also compared the results with already available experimental and clinical results and were found to have comparable results. They successfully demonstrated that the in vitro disease model can be used for drug screening applications.Citation113 To evaluate the effect of microsystem’s geometry and microenvironmental conditions on the morphology and functions of cardiac cells, Kobuszewska et al developed the microfluidics devices with various shapes and different physiologic conditions (static and dynamic). They developed longitudinal, circular, and parallel microfluidic chambers made of PDMS/glass with static and dynamic fluid flow. Rat cardiomyoblasts (H9C2) were seeded on the devices for testing purpose. They concluded that dynamic fluid flow influences the cell morphology, their alignment in fluid flow directions, proliferation, and maturation when compared to static conditions. This study supports the idea that microfluidics devices with dynamic fluid flow mimicked the natural environment necessary for proper growth of cardiac cells.Citation114 Skardal et al fabricated an integrated three-tissue organ-on-a-chip platform consisting of tissues of heart, liver, and lungs to study interorgan interactions and drug response. They evaluated the pharmacokinetics of propranolol and epinephrine in clinically relevant concentrations to control the cardiac organoid beating rate. They also tested the effect of other drug molecules on this integrated organoids-on-a-chip model.Citation115 All these 3D microfluidics models confirmed their future potential to replace currently available in vitro and animal-based in vivo models for drug development applications. Lind et al had presented a 3D printed microfluidics device, which continuously monitors electronic readout of the contractile stress of multiple laminar cardiac microtissues developed in 3D printed microfluidics channels. They used this device for maturation of HSCs-derived laminar cardiac tissue and also for screening of drug to study cardiotoxicity. This 3D printed microfluidics device successfully provides a platform to observe contractile behavior of cardiac tissues and drug screening in closed controlled microenvironment.Citation116
Bioreactors and 3D microfluidics hybrid system for cardiac tissue engineering
Bioengineering has now reached to a level where it can successfully control and reproduce the actual environmental conditions for development, regeneration, and control of cells, while making possible real-time visualization into cellular and their morphogenic events. Unlike 3D tissue engineering approaches, which are not exactly similar to exact natural environment of the heart tissue, bioreactors are able to set a necessary balanced environment for biologic functions that are required for cardiac cell differentiations as well as their functional integration for myocardial tissue regeneration.Citation117 The hybrid bioreactors approach is an advanced strategy in which scientists have used the microfluidics systems for nutrients perfusion and exchange of metabolites and gases, which are difficult to get managed only by cell diffusion in a few-millimeter-thick tissues. Previous studies have successfully shown that media perfusion through microfluidics has increased the cell survival rate in a few-millimeter-thick cardiac tissues.Citation118,Citation119 Radisic and her colleagues used this concept and developed a thicker and functional cardiac tissue through microfluidics perfusion system to supply oxygen to seeded cells. They also developed a 3D bioreactor-based scaffold with multiple parallel channels similar to capillary network to enhance perfusion of cells to match the nutrients demand. They also incorporated an oxygen carrier compound perfluorocarbon (PFC) to mimic the natural oxygen carrier molecule, hemoglobin, in the cell culture medium to fulfill oxygen demand of seeded cells in scaffold. They used this model to calculate total oxygen consumption and tried to find the relation between oxygen distribution in tissues and channel physical dimensions, flow rate, concentration of PFC, and cell density.Citation120 This model provided foundation to investigate detailed insights for engineering of tissue with a specific thickness and cell density, and set the founding principles to mimic natural environment of cardiac muscles tissue engineering. In another similar study, Maidhof et al coupled electrical stimulations with microchannels perfusion bioreactors scaffolds for cardiac tissue engineering. The control of hydrodynamic shear stress due to flow of medium in microchannels and coupling of electrical stimulations enhanced the physiology of cardiac tissue construct. The author used this system to monitor the behaviors of CMs cultured in microchannels by measuring the lower thresholds limit for higher contraction amplitudes (indicative of functional contractile maturation), higher maximum capture rates (indicative of increased intracellular connections), and contractions (indicative of cellular electrical excitability).Citation118
Scaffold-free 3D bioprinting
Despite success of scaffold for tissue engineering, still scaffolds offer problems like immune reaction and degradability. In some cases, the adverse immune reaction by scaffold can be life threatening as it can damage or degenerate area under negative self-immune reaction.Citation103,Citation121 Therefore, scaffold-free 3D bioprinting has emerged as an alternative technique to fabricate artificial organ.Citation61,Citation63 In this 3D bioprinting, scaffold support is compensated through adhesion of cells. Various approaches have been introduced to achieve 3D bioprinting, which includes pellet culture, hanging drop, hydrodynamic cell trapping, spinning flask, liquid overlay, micromolding, and rotating wall vessel techniques.Citation67,Citation122–Citation126 In all these techniques, cell are spread in 3D environment and their rapid differentiation term them into a solid mass due to cell-cell adhesion. Once cells are grown in specific shape they can be placed layer by layer with the support of 3D printer and seeded with other cells robotically to develop a tissue-like form. As in a recent study, alginate microdroplets were used to develop scaffold-free construct. These alginate microdroplets were deposited on calcinated substrate layer by layer into ring-shaped hydrogel molds and seeded with ECs and smooth muscles cells (1:1) robotically and toroid-shaped tissue was developed, which showed tissue morphology, cell–cell adhesion, and maturation.Citation127 Use of scaffold or materials in bioink posed issues of biodegradability, immune reactions, and toxicities. To avoid these complications, Ong et al recently used 3D bioprinting technique for direct delivery of stem cells without addition of biomaterials. They used the mixture of hiPSC-CMs, fibroblast (FB), and ECs in the ratios CM:FB:EC =70:15:15, 70:0:30, 45:40:15 to create spheroids. They used these spheroids for 3D printing of cardiac patches. These patches started beating spontaneously within 3 days while they were still on the needle array, and the voids between the patches got fused in another two days resulting in a complete electrically integrated patch. Patches showed ventricular-like action potential waveforms and uniform alignment of cells and electrical conduction throughout the patch. As these 3D constructs lack presence of any external materials, the ECM of tissue was more natural, which supports the cell–cell interaction and electromechanical coupling.Citation104
But mostly applied technique for 3D bioprinting is tissue-spheroid-based organ bioprinting. Tissue spheroids are 3D well-organized mass of cells, when they are placed over each other in layers; due to surface tension and adhesion they fused into living material and this material reflects the exact similar properties from which the cells were isolated (). They have the advantage to keep their characteristic of native tissue even after their assembly in large structure or organoids.Citation67 Spheroids constituted by mixture of cells on their settlement differentiate into their native structures. Especially for cardiac tissue engineering, seeding of EC cells in CM spheroids showed network of microvascularization. Noguchi et al used tissue spheroids consisting of rat neonatal ventricular CMs, human dermal fibroblasts, and human coronary microartery ECs to construct as viable contractile cardiac patch.Citation128 Similarly, Ong et al used spheroids composed of hiPSC-CMs, fibroblasts, and ECs to fabricate myocardial patches.Citation104 This patch showed myocardium morphology with spontaneous contraction, ventricular-like action potential wave form, and uniform conduction band. Later, this path was grafted in vivo into a rodent model and it showed growth, development, vascularization, and synchronization with the native tissues. These 3D bioprinted constructs by using spheroid showed potential to develop fully functionalized heart or large tissue construct to treat MI.
3D bioprinting of cardiovasculature constructs
Cardiac tissue engineering is directly influenced by angiogenesis or microcirculatory vessels as they are necessary for main nutrients demand and gaseous exchange. Therefore, 3D printing for cardiac tissue engineering involves multidisciplinary approaches and use of mixture of biomaterial, cells, and angiogenic factor as bioink.Citation129 For neovascularization, angiogenic factors play an essential role and can modulate their migration, activate the progenitor (endothelial progenitor) cells and maturation, and promote self-assembly.Citation39 As we discussed earlier, one of the critical challenges in large tissue construct is oxygen supply, while success of tissue construct based on vascularization to keep construct viable is by maintaining required supply of materials and oxygen. 3D bioprinting has emerged as a promising tool to address this challenge by presenting fully biomimetic tissue constructs and organs for clinical applications. The 3D bioprinted organ mimics the anatomical feature and physiology of native tissues and showed initial success with native vascularization. There are two approaches that are used for 3D printing of such vascular structure; one involves direct printing of tubular vessels and other is indirect printing of sacrifice materials (eg, gelatin or pluronic F127) mixed within hydrogel.Citation130 Once the hydrogel scaffold solidifies and shrinks, it leaves a hollow vessel structure that is later seeded with HUVEC cells.Citation130 This second method is known as microextrusion. This method is considered better as compared to direct printing due to its stable tubular structure and choice of broad range of sacrifice materials. But the vascular channels printed by this method have limitations of size and shape due to the nozzle features of 3D printer. Various studies have used this technique with a wide range of hydrogels known to respond less for cells (for example, migration, spreading, and viability) but with high mechanical stability, a factor important for cardiac tissue engineering.Citation131–Citation133 Hinton et al used native materials like fibrin and collagen, which are difficult to handle with extrusion-based system for vascular structure printing.Citation134 Maiullari et al used PF-based scaffolds for developing a 3D cardiac tissue composed of iPSC-derived CMs with a high orientation index imposed by the different defined geometries and blood vessel-like shapes generated by HUVECs and they implanted in vivo subcutaneously in NOD-SCID mice, which demonstrated the integration of the engineered cardiac tissue with host’s vasculature.Citation83
On the other hand, microvalve-based bioprinting technique makes it easy to use materials with low viscosities such as fibrinogen or gelatin (<5% w/v) for 3D printing with better resolution compared to extrusion-based techniques and enhanced cell viability.Citation131,Citation135 The stereolithographic and laser assistance in this technique helps to achieve high resolutions and channels with typical diameters of only a few micrometers to about 125 µm being attained.Citation136 But this technique did not incorporate cells from the first point of printing. To get cellularized vessel structure photocrosslinkable patterning technique is used; in this method, hydrogels are precisely deposited to form an autonomous vessel structure.Citation130,Citation136 Printing of a thick vascularized tissue (>1 cm) with these 3D bioprinting approaches has been reported, which has also shown high cell viability for 5 weeks.Citation137,Citation138 The 3D bioprinted vascular channels were mostly designed and printed as hollow tubes with a diameter ranging from a few hundred micrometersCitation139–Citation141 up to the millimeter rangeCitation61,Citation130 with their inner wall deposited by a single layer of ECs. But the double-layered vascular cell deposition is possible with specific pattern using the multicellular cylinders consisting of two different types of cells, for example human skin fibroblast and human SMCs. This dual-layer patterning is used to develop vascular structures of distinct hierarchical trees and shapes.Citation142 This layer-by-layer deposition of hydrogels and cell mixture of mouse embryonic fibroblast has been used to bioprint an aortic tissue construct.Citation143 Similarly Xiaofeng et al used composition of fibrin and microvascular ECs as bioink for the 3D printing of microvasculature.Citation144 In another study, Fukunishi et al used biodegradable scaffold with autologous cells for 3D printing of vascular conduits and planted it in the inferior vena cavae (IVC) of sheep. After 6 months of implant they explanted the graft and it showed complete dissolution of scaffold and left behind conduit with native IVC tissue morphology.Citation145 Schöneberg et al printed the in vitro blood vessel by using mechanical microvalve inkjet 3D printer. This blood vessel system possesses fibrous and collagenous matrix of normal human dermal fibroblasts (NHDFs) in the outer surface to mimic the adventitia, a human umbilical artery SMC layer to replace the media and a continuous endothelium (HUVEC) imitating the intima.Citation146 Similarly, a natural mimic artery ring was developed by seeding the human aortic smooth muscle cells (HASMCs) on 3D printed tubular fibrin hydrogel.Citation147 The alginate-gelatin solution was used by Liu et al to print artificial vessel construct by using rotating rod.Citation148 Decellularized ECM with stem cells has been reported as bioink for 3D printing of prevascularized functional cardiac construct.Citation149,Citation150 Scaffold-free 3D printing approach has also been used to develop microvasculature structure for cardiac tissue engineering as suction and deposition of multicellular spheroids on a needle array used to print tubular structure.Citation151 Approximately 500 multicellular spheroids composed of NHDFs (50%), HUVECs (40%), and HASMCs (10%) were used to develop this tubular structure by 3D printer and it is perfused for 1 week before implantation into the abdominal aortas of F344 nude rats for 5 days. This tubular graft performance was monitored by ultrasonography and histologic examinations to observe fluid flow after 2-day interval. Remodeling of the tubular tissues (enlargement of the lumen area and thinning of the wall) observed along with assembly of ECs lining the lumen was also confirmed 5 days after implantation. To develop an ideal blood vessel construct, different theoretical models were also proposed to reach native morphology and physiology of blood vessels. These theoretical models consider different parameters like nozzle speed, motor speed, extrusion speed, bioink concentration, viscosity, mechanical properties, and printer speed.Citation152 3D printings of the microvasculatures are one of the key areas of future research to address CVD burden. To explore more self-degrading materials with analogous mechanical properties, computational or mathematical modeling is required to achieve fully functional vessel construct.
Applications of 3D printing in CVDs
3D printing has played a vital role in understanding anatomy, physiology, heart diseases, cardiac masses, electrophysiology, and bioprinting. It has been used in the diagnosis and treatment of valvular heart disease. The 3D printing helps in undermining the aortic anatomy including aortic valve area, root morphology, calcium distribution, and distance to coronary arteries in aortic stenosis.Citation153–Citation155 3D printed functional models of aortic valves have been helping to understand blood flow across the aortic valve.Citation156,Citation157 The 3D models of the aorta precisely mimic the aortic anatomy and can be linked to flow loops to increase physiologic simulation.Citation158,Citation159 Deployment of transcatheter aortic valve replacement (TAVR) is also evaluated by these models to observe their alignment in the presence of severe tortuosity or concomitant mitral valve prosthesis to avoid any obstacle or complication.Citation160,Citation161 Further, TAVR choice (size of annulus, valve type) prediction of future possible leakage of paravalvular and prior planning of its percutaneous closure can be optimized.Citation153–Citation155 Similarly, mitral valve 3D printed functional and static models are used to know the etiology of mitral valve-associates diseases and understanding of blood flow under various loading conditions.Citation162–Citation164 3D printed models are also helping in planning of surgical procedures as in the case of septal myectomy of left ventricular structure.Citation165–Citation169 Such preplanning of surgery can improve postsurgery blood flow for complex repairs as in the case of Nikaidoh surgery in patients with transposition of the great arteries associated with interventricular communication and outflow tract obstruction.Citation170 They are also used in device/stent selection and their optimum positioning to avoid branch vessel obstruction.Citation171,Citation172 Moreover, Tam et al showed that the use of 3D models in procedural planning of complex abdominal aortic aneurysms led to change in endovascular approach in 20% of cases.Citation173
The 3D printed model has applications in diagnosis of heart diseases and helps in better understanding anatomical correlation in congenital heart problems, such as complex ventricular septal and double outlet right ventricles defects as compared to common imaging.Citation174,Citation175 These models for congenital hearts defects facilitate the surgical planning and they are precise as compared to surgical finding to plan optimal baffle orientation.Citation175 Through their accurate demonstration of targeted anatomy of congenital heart defects these 3D models increase the confidence level of the specialist for operative procedures, which was never attained with 3D imaging techniques.
Other than 3D printed applications in treatment and diagnostics of CVDs, these models are also used as educational and communication instruments. They are effective in enhancing the understanding of spatial relationship of congenital heart diseases among students.Citation176–Citation179 Students’ score and their retention rate in medical exams have also shown improvement when they receive demonstration with 3D printed models.Citation180 Further, impact of understanding among students with 3D printed model-based lessons is comparably similar to cadavers,Citation181 while cadavers involve many ethical and financial concerns.Citation182–Citation185 Bench-top functional flow cardiac models are also helping in getting basic knowledge of valvular and coronary artery hemodynamics.Citation156,Citation186 Advanced 3D printed models are setting new horizon of advanced medical education as they have reflected the native tissue properties under ultrasound as compared to real-time imaging; therefore they can be applied for echocardiography simulation.Citation187 This advanced use of 3D heart models is very helpful and relevant for teaching congenital diseases as it demonstrates full spectrum of the problem and the variations that may exist even with a single condition. 3D models provide students with opportunity to have sight and touch experience to achieve rapid understanding of anatomical defects, including complex conditions such as crisscross atrioventricular connections. Beyond the teaching of medical students and staff, 3D printed models are also helpful in communication with patients. Patients can now understand better about their disease and surgical treatments. As a result of this they feel confident and comfortable for providing informed consent and their engagement in shared decision making for operative procedures.Citation188 3D models were also used in multidisciplinary rounds in the intensive care unit to explain treatment plans in complex patients.Citation189 Through this wide range of applications 3D printing technology will help us to further understand the complications and finding new treatments for CVDs.
Foreign body reaction and immunomodulation in cardiac tissue engineering
Any implant faces foreign body immune reaction, and dealing with this reaction is important for successful synchronization and sustainability of 3D implant or tissue construct. During the first contact of the 3D scaffolds with tissue, proteins from body fluids get adsorbed on the surface of the implant. This adsorption of proteins further activates the coagulation cascade and inflammatory response to material.Citation3,Citation4 These protein layers also include ECM proteins like collagen, fibronectin, and vitronectin, and they mainly regulate the inflammatory response.Citation190 Other than adhesion receptors, danger signals also known as alarmins also activate white blood cells and enhance inflammatory response to the implant.Citation191 Alarmins are analogs of pathogen-associated molecular patterns and include heat shock proteins, high mobility group box 1 (HMGB1), ATP, and uric acid. Through these immune players soon after protein adsorption inflammatory cells mainly polymorphonuclear leukocytes (granulocytes) migrate to implant and act as the first line of defense. If this inflammatory response persists, it acts as a driving force to intensify the immune response. Monocytes that reach at the implantation site undergo a phenotypic change differentiating to macrophages and secrete chemokines like IL-8, MCP-1, and MIP-1b to spread message.Citation192 These macrophages attached over the surface of the implant start secreting phenotypically inflammatory cytokines, ROS, and degenerative enzymes and displaying high phagocytic capacity. The cytokines IL-4 and IL-13 have been identified to induce macrophage fusion on biomaterial surfaces in vivo and in vitro.Citation193 Then the phagocyte-specific phenomenon referred to as frustrated phagocytosis starts. All these immune reactions result in inflammations and in severe cases they lead to infection and there is a need to remove the implant. Therefore, scientists are trying to modulate this foreign body reaction to make implant more biocompatible by introducing less immunogenic materials.
Recent research in the understanding of body’s immunologic reactions associated with tissue repairs in MI and other tissue damages has given an impetus on the role of immunomodulation in regenerative medicine.Citation194–Citation197 There occur cascades of immune reactions during an injury in a tissue (), which slowly resolves in a week or 10 days completing its repair process.Citation197 Innate immune system initiates this process, wherein neutrophils followed by monocytes/macrophages are recruited. These are subsequently accompanied by recruitment of T cells and other immune cells. Neutrophils that are recruited first perform phagocytosis with the help of neutrophil extracellular traps. They also help in the recruitment of macrophages and monocytes, which clear off the dying neutrophils and other cellular debris, thus initiating the resolution of inflammation. Macrophages, both the resident as well as newly recruited ones, have a profound role in tissue healing by secretion of proteases, cytokines, growth factors, ECM components, and other soluble mediators promoting tissue repair, fibrosis, or regeneration. These macrophages in the later stage of tissue repair process can take up either a proinflammatory (M1) path or an anti-inflammatory (M2) path based on their cell–cell communication and their microenvironment. The M2 macrophages produce wound healing factors like arginase, ECM components, and growth factors such as VEGF-A, platelet-derived growth factor, and insulin-like growth factor (IGF). The dendritic cells regulate the tissue repair/regeneration process by controlling the recruitment of macrophages. On the other hand, certain T cells (Tregs and αγT cells) also have regulatory functions, such that they regulate the expression of few factors like arginase, anti-inflammatory cytokines like IL-10, TGF-β, amphiregulin, growth factors, and cytokines such as IGF-1, KGF-1 (fibroblast growth factor 7 [FGF-7]), KGF-2 (FGF-10), IL-22, and IL-17A, thus controlling the repair or regeneration process. Overall, it can be understood that there is a need to balance the inflammatory reaction and tissue regeneration and currently there are lots of research carried out to develop biomaterials that can modulate these immunologic reactions and help in the cardiac tissue regeneration process.Citation197,Citation198
Figure 7 Multiple immunologic reactions during tissue repair and regeneration process.
Notes: Biomaterials-based tissue-engineered scaffolds are developed to deliver molecules, which promote regenerative pathways rather than proinflammatory pathways. Reprinted from Acta Biomater, 53, Julier Z, Park AJ, Briquez PS, Martino MM, Promoting tissue regeneration by modulating the immune system, 13–28, Copyright (2017), with permission from Elsevier.Citation197
Abbreviations: ECM, extracellular matrix; IFN, interferon; IGF, insulin-like growth factor; MMP, matrix metalloproteinase; PDGF, platelet-derived growth factor; TIMP, tissue inhibitor of metalloproteinase; TNF, tumor necrosis factor; VEGF, vascular endothelial growth factor.
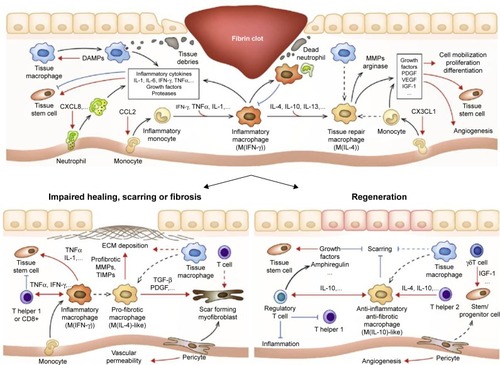
Projahn et al developed two hydrogels with different biodegradation properties, which could release two chemokines at different rates. They synthesized fast degradable hydrogel, which was tuned to release Met-CCL5 (a chemokine that inhibits neutrophil infiltration) within 24 hours of implantation, while slow degradable hydrogel releases recombinant protease-resistant CXCL12 (S4V) (a chemokine that stimulates hematopoietic stem cell recruitment thereby improving neovascularization) for 4 weeks in a controlled manner. They found that due to the temporal control of these chemokines’ release, there is a significant suppression of initial neutrophils, reduction of apoptosis, and promotion of neovascularization, which leads to an improvement in the cardiac function after MI.Citation199 In another study, Kim et al treated mesenchymal stem cells with TNFα before transplantation into MI heart in a rat model. They found that treatment of these stem cells with TNFα improved their engraftment with the tissue and enhanced the recovery of cardiac tissue after MI. They found that this might be because of the expression of BMP2 as a result of this treatment.Citation200 Chen et al devised a heparin-based coacervate delivery system for the controlled delivery of an angiogenic factor FGF2 and an anti-inflammatory cytokine, IL-10. When this coacervate system was injected into mouse MI model, they found an improvement in the long-term LV contractile function, LV myocardial elasticity, and revascularization. They also observed mitigation of LV dilation, LV fibrosis, and also chronic inflammation at the infarct site.Citation201 Thus, these are few strategies by which immunomodulation can augment the regenerative strategies that we had discussed earlier in this review.
Future directions
Despite so much advancement in 3D printing technology and its applications in cardiac tissue engineering, diagnostics, education, and treatments of CVDs, there is still space for future improvements. Areas such as advancements in image acquisition with high resolution, segmentation software, printing speed and resolution, cost, and printer types need further advancements. Future progress in printing materials and use of hybrid materials will reduce the gap between mechanical properties of scaffold and their respective demand for cardiovascular tissues. The applications of 3D printer will continue to grow in the field of bioprinting, a scaffold-free approach to get fully functional cardiac construct and blood vessels. Demand of whole organ and engineered vessels will keep growing by time with high aging population and rise in chronic illness.Citation202 In future, this 3D bioprinting technology will move toward personal-ized tissue engineering, as it can be potentially utilized in fabricating customized devices, cardiac patches, arteries and vessels, valve, and prosthesis. Already few hospitals have demonstrated patients special models of various cardiovascular pathologies by using combined technologies of hybrid material 3D printing, high–spatial resolution cardiac imaging, and image processing software.Citation168,Citation203,Citation204 Further, each university and hospital will invest in this field to print their own models and will use them as essential standard teaching modules along with conventional radiologic imaging. This notion of a personalized 3D printed cardiovascular solution is not here today, but is now clearly expected on the horizon. As diagnostic modalities, quality assurance programs will start as 3D printing is expected to be subject to more federal regulations. 3D printing will be a major advancement in setting new trends in diagnostics and therapeutics. For one, it would be expected that efforts toward direct in vivo bioprinting of biomimetic constructs to replace and enhance regeneration of compromised organ parts would eventually be realized in clinics. With enhancement of precision and optimum 3D printed product, shifting of this technology to robotic instruments for simplifying and scalable automated biofabrication along with portable bioprinters will be the focus areas of future research. To meet with the future increased demand of organs and vascular structures, fast and smart bioprinters will be required in clinics to construct reliably clinically relevant constructs according to the demand of patients as compared to today’s slow bioprinters used in laboratory for research. Similarly, brick and building concept will be also a future model of bioprinting, in which mini-tissue blocks will be fabricated and scale up by joining them to get whole organ or part of organ according to the patient demand or the other approach may employ bioprinting of macroscale vascular network in tandem with microvascularized tissue strands. Importantly, stem cell technologies will play an essential role in transferring these thoughts into reality. For example, cytograft’s lfeline vascular graft requires 6–9 months of developing time before it is ready for implantation. Most of this time is passed in culturing fibroblast sheets. The iPSC-derived SMCs have been shown to generate abundant collagen within 8–9 weeks of culture time.Citation205 This high proliferation ability of iPSCs will not reduce the bioink production time but eliminate the need for harvesting cells from patients or donors.Citation206
Conclusion
CVDs are among the leading cause of morbidity and mortality globally. Due to lack of organ donors, alternative approaches to repair MI and restore cardiac functions are essential. Tissue engineering and especially 3D scaffold and 3D printing showed significant success to develop artificial heart constructs. But still fabricating properly functional tissue construct for clinical applications depends on the availability of biomimetic materials with fine ECM feature and electrical conductance properties. To some extent supply of oxygen to artificial tissue construct is addressed by oxygen generation biomaterials. These biomaterials along with conductive elastomers are future of cardiac tissue engineering to address ischemia and spontaneous contractions issues with newly constructed cardiac tissue. Furthermore, 3D printed advanced bioreactors that can integrate electrical and mechanical stimulation will be another potential area for developing functional heart. 3D microfabrication technique is designed to engineer a highly vascularized cardiac construct on chip with controlled nutrient supply. Seeding of the 3D micropatterned chips with stem cell, growth factors, and other small biomolecules will give well cardiogenic differentiated and prevascularized functional heart constructs. 3D bioprinting using tissue spheroids is an attractive alternative as it allows customized tissue design without the use of scaffolds and avoids the problems with cell injury during the dispensing of the biomaterial. Decellularization of heart tissue can be a solution as it can create constructs with native alignments of cells. In future there is a need to integrate all conventional 3D tissue engineering techniques with modern scaffold-free 3D bioprinting to achieve the most realistic functional heart to address the burden of CVDs.
Acknowledgments
Although we are the authors of this review, we would never have been able to complete it without the great contribution of many people in the field of cardiac and vascular tissue engineering. We owe our gratitude to all those researchers who have made this review possible. We have cited as many references as permitted and apologize to the authors of those publications that we have not cited due to limitation of references. We apologize to other authors who have worked on these aspects but whom we have unintentionally overlooked. This work was supported by a grant from the Science Research Center (2015R1A5A1009701) of the National Research Foundation of Korea.
Disclosure
The authors report no conflicts of interest in this work.
References
- RothGAJohnsonCAbajobirAGlobal, regional, and national burden of cardiovascular diseases for 10 causes, 1990 to 2015J Am Coll Cardiol201770112528527533
- Writing Group MembersMozaffarianDBenjaminEJHeart disease and stroke Statistics-2016 update: a report from the American Heart AssociationCirculation20161334e38e36026673558
- TownsendNNicholsMScarboroughPRaynerMCardiovascular disease in Europe – epidemiological update 2015Eur Heart J201536402696270526306399
- CarabelloBACrawfordFAValvular heart diseaseN Engl J Med1997337132419203430
- MickellJJMathewsRAParkSCLeft atrioventricular valve atresia: clinical managementCirculation19806111231277349925
- LeoniDRelloJCardiac arrest among patients with infections: causes, clinical practice and research implicationsClin Microbiol Infect2017231073073527903458
- WilckenDELClinical physiology of the normal heartOxford Textb Med20103026192627
- ShadrinIYAllenBWQianYCardiopatch platform enables maturation and scale-up of human pluripotent stem cell-derived engineered heart tissuesNat Commun201781182529184059
- Al-AqeediRAsaadNAl-QahtaniAAcute coronary syndrome in patients with prior coronary artery bypass surgery: observations from a 20-year registry in a Middle-Eastern countryPLoS One201277e4057122815766
- TchantchaleishviliVSchubmehlHSwartzMFHallinanWMasseyHTEvolving strategies in the treatment of acute myocardial infarction-induced cardiogenic shockAnn Cardiothorac Surg20143660661125512903
- AcharyaDLoyaga-RendonRYPamboukianSVVentricular assist device in acute myocardial infarctionJ Am Coll Cardiol201667161871188027102502
- AlrefaiMTMuraliDPaulACardiac tissue engineering and regeneration using cell-based therapyStem Cells Cloning201588110125999743
- WhiteSLHirthRMahílloBThe global diffusion of organ transplantation: trends, drivers and policy implicationsBull World Health Organ2014921182683525378744
- SürderDSchwitterJMoccettiTCell-based therapy for myocardial repair in patients with acute myocardial infarction: rationale and study design of the Swiss multicenter intracoronary stem cells study in acute myocardial infarction (SWISS-AMI)Am Heart J20101601586420598973
- ZimmermannWDidieMDokerSHeart muscle engineering: an update on cardiac muscle replacement therapyCardiovasc Res200671341942916697358
- O’NeillHSGallagherLBO’SullivanJBiomaterial-enhanced cell and drug delivery: lessons learned in the cardiac field and future perspectivesAdv Mater201628275648566126840955
- DomenechMPolo-CorralesLRamirez-VickJEFreytesDOTissue engineering strategies for myocardial regeneration: acellular versus cellular scaffolds?Tissue Eng B Rev2016226438458
- WangQLWangHJLiZHMesenchymal stem cell-loaded cardiac patch promotes epicardial activation and repair of the infarcted myocardiumJ Cell Mol Med20172191751176628244640
- WollertKCMeyerGPLotzJIntracoronary autologous bone-marrow cell transfer after myocardial infarction: the boost randomised controlled clinical trialLancet2004364942914114815246726
- AssmusBSchächingerVTeupeCTransplantation of progenitor cells and regeneration enhancement in acute myocardial infarction (TOPCARE-AMI)Circulation2002106243009301712473544
- WollertKCMeyerGPLotzJIntracoronary autologous bone-marrow cell transfer after myocardial infarction: the boost randomised controlled clinical trialLancet2004364942914114815246726
- SchächingerVErbsSElsässerAImproved clinical outcome after intracoronary administration of bone-marrow-derived progenitor cells in acute myocardial infarction: final 1-year results of the REPAIR-AMI trialEur Heart J200627232775278317098754
- ThavandiranNNunesSSXiaoYRadisicMTopological and electrical control of cardiac differentiation and assemblyStem Cell Res Ther2013411423425700
- Vunjak-NovakovicGTandonNGodierAChallenges in cardiac tissue engineeringTissue Eng Part B Rev201016216918719698068
- VisoneRGilardiMMarsanoACardiac meets skeletal: what’s new in microfluidic models for muscle tissue engineeringMolecules2016219 pii: E1128
- DuanBKapetanovicEHockadayLAButcherJTThree-dimensional printed trileaflet valve conduits using biological hydrogels and human valve interstitial cellsActa Biomater20141051836184624334142
- GuyetteJPCharestJMMillsRWBioengineering human myocardium on native extracellular matrixCirc Res20161181567226503464
- LiuQTianSZhaoCPorous nanofibrous poly(L-lactic acid) scaffolds supporting cardiovascular progenitor cells for cardiac tissue engineeringActa Biomater20152610511426283164
- NistorM-TChiriacAPNitaLEVasileCVerestiucLUpon the characterization of semi-synthetic hydrogels based on poly (NIPAM) inserted onto collagen spongeCompos B Eng201243315081515
- QasimMLimDJParkHNaDNanotechnology for diagnosis and treatment of infectious diseasesJ Nanosci Nanotechnol201414107374738725942798
- ChenYZengDDingLThree-dimensional poly-(ε-caprolactone) nanofibrous scaffolds directly promote the cardiomyocyte differentiation of murine-induced pluripotent stem cells through Wnt/β-catenin signalingBMC Cell Biol2015162226335746
- LiuBHYehHYLinYCSpheroid formation and enhanced cardiomyogenic potential of adipose-derived stem cells grown on chitosanBiores Open Access201321283923514754
- PrabhakaranMPKaiDGhasemi-MobarakehLRamakrishnaSElectrospun biocomposite nanofibrous patch for cardiac tissue engineeringBiomed Mater20116505500121813957
- RavichandranRSridharRVenugopalJRGold nanoparticle loaded hybrid nanofibers for cardiogenic differentiation of stem cells for infarcted myocardium regenerationMacromol Biosci201414451552524327549
- GalperinALongTJRatnerBDDegradable, thermo-sensitive poly(N-isopropyl acrylamide)-based scaffolds with controlled porosity for tissue engineering applicationsBiomacromolecules201011102583259220836521
- XuYPatnaikSGuoXCardiac differentiation of cardiosphere-derived cells in scaffolds mimicking morphology of the cardiac extracellular matrixActa Biomater20141083449346224769114
- LaschkeMWMengerMDLife is 3d: boosting spheroid function for tissue engineeringTrends Biotechnol201735213314427634310
- OuKLHosseinkhaniHDevelopment of 3D in vitro technology for medical applicationsInt J Mol Sci20141510179381796225299693
- AmannAZwierzinaMKoeckSDevelopment of a 3D angio-genesis model to study tumour – endothelial cell interactions and the effects of anti-angiogenic drugsSci Rep201771296328592821
- van DuinenVTrietschSJJooreJVultoPHankemeierTMicrofluidic 3D cell culture: from tools to tissue modelsCurr Opin Biotechnol20153511812626094109
- HanJWuQXiaYWagnerMBXuCCell alignment induced by anisotropic electrospun fibrous scaffolds alone has limited effect on cardiomyocyte maturationStem Cell Res201616374075027131761
- ChongJJYangXdonCWHuman embryonic-stem-cell-derived cardiomyocytes regenerate non-human primate heartsNature2014510750427327724776797
- ValverdeIThree-dimensional printed cardiac models: applications in the field of medical education, cardiovascular surgery, and structural heart interventionsRev Esp Cardiol201770428229128189544
- WangZLeeSJChengHJYooJJAtalaA3D bioprinted functional and contractile cardiac tissue constructsActa Biomater201870485629452273
- KensahGRoa LaraADahlmannJMurine and human pluripotent stem cell-derived cardiac bodies form contractile myocardial tissue in vitroEur Heart J201334151134114623103664
- CaspiOLesmanABasevitchYTissue engineering of vascularized cardiac muscle from human embryonic stem cellsCirc Res2007100226327217218605
- ConantGLaiBFLLuRXZHigh-content assessment of cardiac function using heart-on-a-chip devices as drug screening modelStem Cell Rev Rep2017133335346
- MladěnkaPApplováLPatočkaJComprehensive review of cardiovascular toxicity of drugs and related agentsMed Res Rev20183841332140329315692
- SaludasLPascual-GilSPrósperFGarbayoEBlanco-PrietoMHydrogel based approaches for cardiac tissue engineeringInt J Pharm2017523245447527989830
- XuBLiYDengBChitosan hydrogel improves mesenchymal stem cell transplant survival and cardiac function following myocardial infarction in ratsExp Ther Med201713258859428352335
- KimPHChoJYMyocardial tissue engineering using electrospun nanofiber compositesBMB Rep2016491263626497579
- YuanXHeBLvZLuoSFabrication of self-assembling peptide nanofiber hydrogels for myocardial repairRSC Adv20144965380153811
- JoshiJKothapalliCRNanofibers based tissue engineering and drug delivery approaches for myocardial regenerationCurr Pharm Des201521152006202025732660
- BalashovVEfimovAAgapovaOHigh resolution 3D microscopy study of cardiomyocytes on polymer scaffold nanofibers reveals formation of unusual sheathed structureActa Biomater20186821422229288823
- MartinsAMEngGCaridadeSGElectrically conductive chitosan/carbon scaffolds for cardiac tissue engineeringBiomacromolecules201415263564324417502
- GilpinAYangYDecellularization strategies for regenerative medicine: from processing techniques to applicationsBiomed Res Int20172017113
- LuTYLinBKimJRepopulation of decellularized mouse heart with human induced pluripotent stem cell-derived cardiovascular progenitor cellsNat Commun20134111
- TaylorDAParikhRBSampaioLCBioengineering hearts: simple yet complexCurr Stem Cell Rep201731354428261549
- Vunjak-NovakovicGLuiKOTandonNChienKRBioengineering heart muscle: a paradigm for regenerative medicineAnnu Rev Biomed Eng20111324526721568715
- SidorovVYSamsonPCSidorovaTNI-Wire Heart-on-a-Chip I: three-dimensional cardiac tissue constructs for physiology and pharmacologyActa Biomater201748687827818308
- ZhuWQuXZhuJDirect 3D bioprinting of prevascularized tissue constructs with complex microarchitectureBiomaterials201712410611528192772
- ChionoVMozeticPBoffitoMPolyurethane-based scaffolds for myocardial tissue engineeringInterface Focus2014412013004524501673
- JiaWGungor-OzkerimPSZhangYSDirect 3D bioprinting of perfusable vascular constructs using a blend bioinkBiomaterials2016106586827552316
- ZhangYSArneriABersiniSBioprinting 3D microfibrous scaffolds for engineering endothelialized myocardium and heart-on-a-chipBiomaterials2016110455927710832
- YoonSParkJALeeHRInkjet–spray hybrid printing for 3D freeform fabrication of multilayered hydrogel structuresAdv Healthc Mater20187110
- JammalamadakaUTappaKRecent advances in biomaterials for 3D printing and tissue engineeringJ Funct Biomater2018922
- MeheszANBrownJHajduZScalable robotic biofabrication of tissue spheroidsBiofabrication20113202500221562365
- MironovVViscontiRPKasyanovVOrgan printing: tissue spheroids as building blocksBiomaterials200930122164217419176247
- FatimaABreast cancer copy number profiling of MammaPrint TM genes reveals association with the prognosis of breast cancer patients201720246253
- MertzLNew world of 3-D printing offers “Completely New Ways of Thinking”: Q&A with author, engineer, and 3-D printing expert Hod lipsonIEEE Pulse201341214
- OzbolatITHospodiukMCurrent advances and future perspectives in extrusion-based bioprintingBiomaterials20167632134326561931
- BakhshinejadAD’SouzaRMA brief comparison between available bio-printing methods2015 IEEE Great Lakes biomedical conference (GLBC) 1–3IEEE20152015
- OngCSFukunishiTZhangHBiomaterial-free three-dimensional bioprinting of cardiac tissue using human induced pluripotent stem cell derived cardiomyocytesSci Rep201771456628676704
- UsprechJRomeroDAAmonCHSimmonsCACombinatorial screening of 3D biomaterial properties that promote myofibrogenesis for mesenchymal stromal cell-based heart valve tissue engineeringActa Biomater201758344328532900
- BourgetJGuillemetteMVeresTAugerFAGermainLAlignment of cells and extracellular matrix within tissue-engineered substitutesAdv Biomater Sci Biomed Appl Ref2013365390
- BellAKofronMNistorVMultiphoton crosslinking for biocompatible 3D printing of type I collagenBiofabrication20157303500726335389
- GaoLKupferMEJungJPMyocardial tissue engineering with cells derived from human-induced pluripotent stem cells and a native-like, high-resolution, 3-dimensionally printed scaffoldCirc Res201712081318132528069694
- CmHMishraALinPT3D printed polycaprolactone carbon nanotube composite scaffolds for cardiac tissue engineeringMacromol Biosci20171741600250
- MironovAVGrigoryevAMKrotovaLI3D printing of PLGA scaffolds for tissue engineeringJ Biomed Mater Res A2017105110410927543196
- MirabellaATMacArthurJWChengD3D printed vascular networks direct therapeutic angiogenesis in ischemiaNat Biomed Eng2017008316
- TijoreAIrvineSASarigUContact guidance for cardiac tissue engineering using 3D bioprinted gelatin patterned hydrogelBiofabrication2017102025003
- FleischerSShapiraAFeinerRDvirTModular assembly of thick multifunctional cardiac patchesProc Natl Acad Sci U S A201711481898190328167795
- MaiullariFCostantiniMMilanMA multi-cellular 3D bio-printing approach for vascularized heart tissue engineering based on HUVECs and iPSC-derived cardiomyocytesSci Rep20188111529311619
- SiltanenAKitabayashiKLakkistoPhHGF overexpression in myoblast sheets enhances their angiogenic potential in rat chronic heart failurePLoS One201164e1916121541335
- TakahashiHOkanoTCell sheet-based tissue engineering for organizing anisotropic tissue constructs produced using microfabricated thermoresponsive substratesAdv Healthc Mater20154162388240726033874
- WangHShiJWangYPromotion of cardiac differentiation of brown adipose derived stem cells by chitosan hydrogel for repair after myocardial infarctionBiomaterials201435133986399824508080
- HamidTPrabhuSDImmunomodulation is the key to cardiac repairCirc Res2017120101530153228495983
- MajkaMSułkowskiMBadyraBMusiałekPConcise review: mesenchymal stem cells in cardiovascular regeneration: emerging research directions and clinical applicationsStem Cells Transl Med20176101859186728836732
- CaplanAICell Sources for Tissue Engineering: Mesenchymal Stem Cells Biomaterials Science: An Introduction to Materials3rd edMA, USAElsevier2013
- MichlerREStem cell therapy for heart failureMethodist Debakey Cardiovasc J20139418719424298308
- ThomsonJAItskovitz-EldorJShapiroSSEmbryonic stem cell lines derived from human blastocystsScience19982825391114511479804556
- TakahashiKYamanakaSInduction of pluripotent stem cells from mouse embryonic and adult fibroblast cultures by defined factorsCell2006126466367616904174
- KehatIKhimovichLCaspiOElectromechanical integration of cardiomyocytes derived from human embryonic stem cellsNat Biotechnol200422101282128915448703
- LianXHsiaoCWilsonGRobust cardiomyocyte differentiation from human pluripotent stem cells via temporal modulation of canonical Wnt signalingProc Natl Acad Sci U S A201210927E1848E185722645348
- MummeryCLZhangJNgESDifferentiation of human embryonic stem cells and induced pluripotent stem cells to cardiomyocytes: a methods overviewCirc Res2012111334435822821908
- CsobonyeiovaMPolakSKollerJDanisovicLInduced pluripotent stem cells and their implication for regenerative medicineCell Tissue Bank201516217118025037593
- AmabileGMeissnerAInduced pluripotent stem cells: current progress and potential for regenerative medicineTrends Mol Med2009152596819162546
- BedadaFBWheelwrightMMetzgerJMMaturation status of sarcomere structure and function in human iPSC-derived cardiac myocytesBiochim Biophys Acta Mol Cell Res20161863718291838
- GaldosFXGuoYPaigeSLCardiac regeneration: lessons from developmentCirc Res2017120694195928302741
- ChongJYangXdonCPW281 human pluripotent stem cell derived cardiomyocytes regenerate infarcted hearts of non-human primatesGlob Heart201491e315e316
- CambriaEPasqualiniFSWolintPTranslational cardiac stem cell therapy: advancing from first-generation to next-generation cell typesNPJ Regen Med2017211729302353
- GaetaniRFeyenDAVerhageVEpicardial application of cardiac progenitor cells in a 3D-printed gelatin/hyaluronic acid patch preserves cardiac function after myocardial infarctionBiomaterials20156133934826043062
- GaoLKupferMEJungJPMyocardial tissue engineering with cells derived from human-induced pluripotent stem cells and a native-like, high-resolution, 3-dimensionally printed scaffoldCirc Res201712081318132528069694
- OngCSFukunishiTZhangHBiomaterial-Free three-dimensional bioprinting of cardiac tissue using human induced pluripotent stem cell derived cardiomyocytesSci Rep20177121228127053
- ZhangBMontgomeryMChamberlainMDBiodegradable scaffold with built-in vasculature for cardiac tissue vascularization and surgical vascular anastomosisNat Mater201615666967826950595
- FeinbergAWFeigelAShevkoplyasSSMuscular thin films for building actuators and powering devicesScience200731758431366137017823347
- TanakaYMorishimaKShimizuTDemonstration of a PDMS-based bio-microactuator using cultured cardiomyocytes to drive polymer micropillarsLab Chip20066223016450032
- SheehySPGrosbergAQinPToward improved myocardial maturity in an organ-on-chip platform with immature cardiac myocytesExp Biol Med20172421716431656
- ElhassaGODrug development: stages of drug developmentJ Pharmacovigil20150303140142
- VargaZVFerdinandyPLiaudetLPacherPDrug-induced mitochondrial dysfunction and cardiotoxicityAm J Physiol Hear Circ Physiol20153099H1453H1467
- MarsanoAConficconiCLemmeMBeating heart on a chip: a novel microfluidic platform to generate functional 3D cardiac microtissuesLab Chip20161659961026758922
- ZhangBMontgomeryMChamberlainMDBiodegradable scaffold with built-in vasculature for organ-on-a-chip engineering and direct surgical anastomosisNat Mater201615666967826950595
- HortonREYadidMMccainMLAngiotensin II induced cardiac dysfunction on a chipPLoS One2016111e014641526808388
- KobuszewskaATomeckaEZukowskiKHeart-on-a-Chip: an investigation of the influence of static and perfusion conditions on cardiac (H9c2) cell proliferation, morphology, and alignmentSLAS Technol201722553654628430559
- SkardalAMurphySVDevarasettyMMulti-tissue interactions in an integrated three-tissue organ-on-a-chip platformSci Rep20177111628127051
- LindJUBusbeeTAValentineADInstrumented cardiac micro-physiological devices via multimaterial three-dimensional printingNat Mater201716330330827775708
- HasanASalibaJPezeshgi ModarresHMicro and nanotechnologies in heart valve tissue engineeringBiomaterials201610327829227414719
- MaidhofRTandonNLeeEJBiomimetic perfusion and electrical stimulation applied in concert improved the assembly of engineered cardiac tissueJ Tissue Eng Regen Med2012610e12e2322170772
- TandonNTaubmanACimettaESaccentiLVunjak-NovakovicGPortable bioreactor for perfusion and electrical stimulation of engineered cardiac tissueConf Proc IEEE Eng Med Biol Soc201320136219622324111161
- BrownMAIyerRKRadisicMPulsatile perfusion bioreactor for cardiac tissue engineeringBiotechnol Prog200824490792019194900
- OwakiTShimizuTYamatoMOkanoTCell sheet engineering for regenerative medicine: current challenges and strategiesBiotechnol J20149790491424964041
- LeeKKimCYoung YangJGravity-oriented microfluidic device for uniform and massive cell spheroid formationBiomicrofluidics201261014114
- TungYCHsiaoAYAllenSGHigh-throughput 3D spheroid culture and drug testing using a 384 hanging drop arrayAnalyst2011136347347820967331
- FuCYTsengSYYangSMA microfluidic chip with a U-shaped microstructure array for multicellular spheroid formation, culturing and analysisBiofabrication20146101500924589876
- LiQChowABMattinglyRRThree-dimensional overlay culture models of human breast cancer reveal a critical sensitivity to mitogen-activated protein kinase kinase inhibitorsJ Pharmacol Exp Ther2010332382182819952304
- BarrilaJRadtkeALCrabbéAOrganotypic 3D cell culture models: using the rotating wall vessel to study host-pathogen interactionsNat Rev Microbiol201081179180120948552
- TanYRichardsDJTruskTC3D printing facilitated scaffold-free tissue unit fabricationBiofabrication20146202411124717646
- NoguchiRNakayamaKItohMDevelopment of a three-dimensional pre-vascularized scaffold-free contractile cardiac patch for treating heart diseaseJ Heart Lung Transplant201635113714526433566
- SongHGRummaRTOzakiCKEdelmanERChenCSVascular tissue engineering: progress, challenges, and clinical promiseCell Stem Cell201822334035429499152
- DattaPAyanBOzbolatITBioprinting for vascular and vascularized tissue biofabricationActa Biomater20175112028087487
- MurphySVAtalaA3D bioprinting of tissues and organsNat Biotechnol201432877378525093879
- MurphySVSkardalAAtalaAEvaluation of hydrogels for bio-printing applicationsJ Biomed Mater Res A2013101A1272284
- GaoQLiuZLinZ3D bioprinting of vessel-like structures with multilevel fluidic channelsACS Biomater Sci Eng201733399408
- HintonTJJalleratQPalcheskoRNThree-dimensional printing of complex biological structures by freeform reversible embedding of suspended hydrogelsSci Adv201519e150075826601312
- MaldaJVisserJMelchelsFP25th anniversary article: engineering hydrogels for biofabricationAdv Mater201325365011502824038336
- HochETovarGEMBorchersKBioprinting of artificial blood vessels: current approaches towards a demanding goalEur J Cardio-Thoracic Surg2014465767778
- KangHWLeeSJKoIKA 3D bioprinting system to produce human-scale tissue constructs with structural integrityNat Biotechnol201634331231926878319
- KoleskyDBHomanKASkylar-ScottMALewisJAThree-dimensional bioprinting of thick vascularized tissuesProc Natl Acad Sci U S A2016113123179318426951646
- van der MeerADOrlovaVVTen DijkePvan den BergAMummeryCLThree-dimensional co-cultures of human endothelial cells and embryonic stem cell-derived pericytes inside a microfluidic deviceLab Chip20131318356223702711
- ChrobakKMPotterDRTienJFormation of perfused, functional microvascular tubes in vitroMicrovasc Res200671318519616600313
- HasanAPaulAVranaNEMicrofluidic techniques for development of 3D vascularized tissueBiomaterials201435267308732524906345
- NorotteCMargaFSNiklasonLEForgacsGScaffold-free vascular tissue engineering using bioprintingBiomaterials200930305910591719664819
- KucukgulCOzlerSBInciI3D bioprinting of biomimetic aortic vascular constructs with self-supporting cellsBiotechnol Bioeng2015112481182125384685
- CuiXBolandTHuman microvasculature fabrication using thermal inkjet printing technologyBiomaterials200930316221622719695697
- FukunishiTBestCASugiuraTPreclinical study of patient-specific cell-free nanofiber tissue-engineered vascular grafts using 3-dimensional printing in a sheep modelJ Thorac Cardiovasc Surg2017153492493227938900
- SchönebergJde LorenziFTheekBEngineering biofunctional in vitro vessel models using a multilayer bioprinting techniqueSci Rep2018811043029992981
- PinnockCBMeierEMJoshiNNWuBLamMTCustomizable engineered blood vessels using 3D printed insertsMethods20169920–27202726732049
- LiuHZhouHLanH3D printing of artificial blood vessel: study on multi-parameter optimization design for vascular molding effect in alginate and gelatinMicromachines201788237
- JangJParkHJKimSW3D printed complex tissue construct using stem cell-laden decellularized extracellular matrix bioinks for cardiac repairBiomaterials201711226427427770630
- PatiFChoDWBioprinting of 3D tissue models using decellularized extracellular matrix bioinkMethods Mol Biol2017161238139028634957
- ItohMNakayamaKNoguchiRScaffold-free tubular tissues created by a Bio-3D printer undergo remodeling and endothelialization when implanted in rat aortaePLoS One2015109e013668126325298
- CabreraMSSandersBGoorOJGMComputationally designed 3D printed self-expandable polymer stents with biodegradation capacity for minimally invasive heart valve implantation: a proof-of-concept study3D Print Addit Manuf2017411929
- FujitaBKüttingMSeiffertMCalcium distribution patterns of the aortic valve as a risk factor for the need of permanent pacemaker implantation after transcatheter aortic valve implantationEur Heart J Cardiovasc Imaging201617121385139326758411
- Hernández-EnríquezMBrugalettaSAndreuDThree-dimensional printing of an aortic model for transcatheter aortic valve implantation: possible clinical applicationsInt J Cardiovasc Imaging201733228328527757564
- JungJIKohYSChangK3D printing model before and after trans-catheter aortic valve implantation for a better understanding of the anatomy of aortic rootKorean Circ J201646458858927482272
- MaragiannisDJacksonMSIgoSRReplicating patient-specific severe aortic valve stenosis with functional 3D modelingCirc Cardiovasc Imaging2015810e00362626450122
- MaragiannisDJacksonMSIgoSRFunctional 3D printed patient-specific modeling of severe aortic stenosisJ Am Coll Cardiol201464101066106825190245
- HoDSquelchASunZModelling of aortic aneurysm and aortic dissection through 3D printingJ Med Radiat Sci2017641101728134482
- MafeldSNesbittCMccaslinJThree-dimensional (3D) printed endovascular simulation models: a feasibility studyAnn Transl Med2017534228251121
- FujitaTSaitoNMinakataKTransfemoral transcatheter aortic valve implantation in the presence of a mechanical mitral valve prosthesis using a dedicated TAVI guidewire: utility of a patient-specific three-dimensional heart modelCardiovasc Interv Ther201732330831127568401
- GalloMD’OnofrioATarantiniG3D-printing model for complex aortic transcatheter valve treatmentInt J Cardiol201621013914026945434
- MashariAKnioZJeganathanJHemodynamic testing of patient-specific mitral valves using a pulse duplicator: a clinical application of three-dimensional printingJ Cardiothorac Vasc Anesth20163051278128527179613
- VukicevicMPuperiDSJane Grande-AllenKLittleSH3D printed modeling of the mitral valve for catheter-based structural interventionsAnn Biomed Eng201745250851927324801
- WitscheyWRPouchAMMcgarveyJRThree-dimensional ultrasound-derived physical mitral valve modelingAnn Thorac Surg201498269169425087790
- BartelTRivardAJimenezAMestresCAMüllerSMedical three-dimensional printing opens up new opportunities in cardiology and cardiac surgeryEur Heart J201839151246125428329105
- JacobsSGrunertRMohrFWFalkV3D-imaging of cardiac structures using 3D heart models for planning in heart surgery: a preliminary studyInteract Cardiovasc Thorac Surg2008716917925319
- MartelliNSerranoCvan den BrinkHAdvantages and disadvantages of 3-dimensional printing in surgery: a systematic reviewSurgery201615961485150026832986
- SchmaussDHaeberleSHaglCSodianRThree-dimensional printing in cardiac surgery and interventional cardiology: a single-centre experienceEur J Cardio-Thoracic Surg201547610441052
- YangDHKangJWKimNMyocardial 3-dimensional printing for septal myectomy guidance in a patient with obstructive hypertrophic cardiomyopathyCirculation2015132430030126216088
- ValverdeIGomezGGonzalezAThree-dimensional patient-specific cardiac model for surgical planning in Nikaidoh procedureCardiol Young201525469870424809416
- HuangJLiGWangWWuKLeT3D printing guiding stent graft fenestration: a novel technique for fenestration in endovascular aneurysm repairVascular201725444244627928064
- SchmaussDGerberNSodianRThree-dimensional printing of models for surgical planning in patients with primary cardiac tumorsJ Thorac Cardiovasc Surg201314551407140823312105
- TamMDLathamTRLewisMA pilot study assessing the impact of 3-D printed models of aortic aneurysms on management decisions in EVAR planningVasc Endovascular Surg20165014926912523
- BhatlaPTretterJTLudomirskyAUtility and scope of rapid prototyping in patients with complex muscular ventricular septal defects or double-outlet right ventricle: does it alter management decisions?Pediatr Cardiol201738110311427837304
- GarekarSBharatiAChokhandreMClinical application and multidisciplinary assessment of three dimensional printing in double outlet right ventricle with remote ventricular septal defectWorld J Pediatr Congenit Heart Surg20167334435027142402
- BiglinoGCapelliCKoniordouDUse of 3D models of congenital heart disease as an education tool for cardiac nursesCongenit Heart Dis201712111311827666734
- BramletMOlivieriLFarooqiKRipleyBCoakleyMImpact of three-dimensional printing on the study and treatment of congenital heart diseaseCirc Res2017120690490728302738
- CostelloJPOlivieriLJKriegerAUtilizing three-dimensional printing technology to assess the feasibility of high-fidelity synthetic ventricular septal defect models for simulation in medical educationWorld J Pediatr Congenit Heart Surg20145342142624958045
- FarooqiKMUppuSCNguyenKApplication of virtual three-dimensional models for simultaneous visualization of intracardiac anatomic relationships in double outlet right ventriclePediatr Cardiol2016371909826254102
- ValverdeIThree-dimensional printed cardiac models: applications in the field of medical education, cardiovascular surgery, and structural heart interventionsRev Esp Cardiol201770428229128189544
- LimKHLooZYGoldieSJAdamsJWMcmenaminPGUse of 3D printed models in medical education: a randomized control trial comparing 3D prints versus cadaveric materials for learning external cardiac anatomyAnat Sci Educ20169321322126468636
- AbouhashemYDayalMSavanahSŠtrkaljGThe application of 3D printing in anatomy educationMed Educ Online2015202984726478143
- DrakeRLPawlinaWAn addition to the neighborhood: 3D printed anatomy teaching resourcesAnat Sci Educ20147641925355164
- EstaiMBuntSBest teaching practices in anatomy education: a critical reviewAnn Anat201620815115726996541
- CoakleyMFHurtDEWeberNThe NIH 3D print exchange: a public resource for bioscientific and biomedical 3D printsAddit Manuf201413137140
- WoodRPKhobragadePYingLInitial testing of a 3D printed perfusion phantom using digital subtraction angiography. In Gimi B, Molthen RC, editorsProceedings of SPIE – the International Society for Optical Engineering2015941794170V
- MooneyJJSarwaniNColemanMLFotosJSEvaluation of three-dimensional printed materials for simulation by computed tomography and ultrasound imagingSimul Healthc201712318218828166189
- BiglinoGCapelliCWrayJ3D-manufactured patient-specific models of congenital heart defects for communication in clinical practice: feasibility and acceptabilityBMJ Open201554e007165
- OlivieriLJSuLHynesCFJust-In-Time” simulation training using 3-D printed cardiac models after congenital cardiac surgeryWorld J Pediatr Congenit Heart Surg20167216416826957398
- McfarlandCDThomasCHDefilippisCSteeleJGHealyKEProtein adsorption and cell attachment to patterned surfacesJ Biomed Mater Res200049220021010571906
- BianchiMEDAMPs, PAMPs and alarmins: all we need to know about dangerJ Leukoc Biol200781115
- JonesJAChangDTMeyersonHProteomic analysis and quantification of cytokines and chemokines from biomaterial surface-adherent macrophages and foreign body giant cellsJ Biomed Mater Res A200783A3585596
- DefifeKMJenneyCRMcnallyAKColtonEAndersonJMInterleukin-13 induces human monocyte/macrophage fusion and macrophage mannose receptor expressionJ Immunol19971587338533909120298
- AuroraABOlsonENImmune modulation of stem cells and regenerationCell Stem Cell2015151425
- SpillerKLKohTJMacrophage-based therapeutic strategies in regenerative medicineAdv Drug Deliv Rev2017122748328526591
- WynnTAVannellaKMMacrophages in tissue repair, regeneration, and fibrosisImmunity201644345046226982353
- JulierZParkAJBriquezPSMartinoMMPromoting tissue regeneration by modulating the immune systemActa Biomater201753132828119112
- AlvarezMMLiuJCTrujillo-de SantiagoGDelivery strategies to control inflammatory response: modulating M1-M2 polarization in tissue engineering applicationsJ Control Release201624034936326778695
- ProjahnDSimsekyilmazSSinghSControlled intramyocardial release of engineered chemokines by biodegradable hydrogels as a treatment approach of myocardial infarctionJ Cell Mol Med201418579080024512349
- KimYSParkHJHongMHTNF-alpha enhances engraftment of mesenchymal stem cells into infarcted myocardiumFront Biosci20091428452856
- ChenWCLeeBGParkDWControlled dual delivery of fibroblast growth factor-2 and interleukin-10 by heparin-based coacervate synergistically enhances ischemic heart repairBiomaterials20157213815126370927
- PrabhakaranDAnandSWatkinsDCardiovascular, respiratory, and related disorders: key messages from Disease Control Priorities, 3rd editionThe Lancet20183911012612241236
- BiglinoGCapelliCTaylorAMSchievanoSNew Trends in 3D PrintingLondonInTech2016
- VukicevicMMosadeghBMinJKLittleSHCardiac 3D printing and its future directionsJACC Cardiovasc Imaging201710217118428183437
- GuiLDashBCLuoJImplantable tissue-engineered blood vessels from human induced pluripotent stem cellsBiomaterials201610212012927336184
- DimitrievskaSNiklasonLEHistorical perspective and future direction of blood vessel developmentsCold Spring Harb Perspect Med201882a02574228348177
- MironovAVGrigoryevAMKrotovaLI3D printing of PLGA scaffolds for tissue engineeringJ Biomed Mater Res A2017105110410927543196
- WangZLeeSJChengH-JYooJJAtalaA3D bioprinted functional and contractile cardiac tissue constructsActa Biomater201870485629452273