Abstract
During recent decades there have been remarkable advances and profound changes in cancer therapy. Many therapeutic strategies learned at the bench, including monoclonal antibodies and small molecule inhibitors, have been used at the bedside, leading to important successes. One of the most important advances in biology has been the discovery that small interfering RNA (siRNA) is able to regulate the expression of genes, by a phenomenon known as RNA interference (RNAi). RNAi is one of the most rapidly growing fields of research in biology and therapeutics. Much research effort has gone into the application of this new discovery in the treatment of various diseases, including cancer. However, even though these molecules may have potential and strong utility, some limitations make their clinical application difficult, including delivery problems, side effects due to off-target actions, disturbance of physiological functions of the cellular machinery involved in gene silencing, and induction of the innate immune response. Many researchers have attempted to overcome these limitations and to improve the safety of potential RNAi-based therapeutics. Nanoparticles, which are nanostructured entities with tunable size, shape, and surface, as well as biological behavior, provide an ideal opportunity to modify current treatment regimens in a substantial way. These nanoparticles could be designed to surmount one or more of the barriers encountered by siRNA. Nanoparticle drug formulations afford the chance to improve drug bioavailability, exploiting superior tissue permeability, payload protection, and the “stealth” features of these entities. The main aims of this review are: to explain the siRNA mechanism with regard to potential applications in siRNA-based cancer therapy; to discuss the possible usefulness of nanoparticle-based delivery of certain molecules for overcoming present therapeutic limitations; to review the ongoing relevant clinical research with its pitfalls and promises; and to evaluate critically future perspectives and challenges in siRNA-based cancer therapy.
Introduction
During recent years, cancer therapy has undergone important changes and improvements. Learning from translational medicine, new therapeutic strategies, such as small molecule inhibitors and monoclonal antibodies, have been introduced into clinical practice.Citation1–Citation3 These successes have encouraged the research field, and regulation of gene expression is one of the most frequently addressed issues in translational medicine.
Traditionally, RNA in cells has been thought to have two fundamental functions. Until 1980, single-stranded messenger RNA (mRNA) was considered to be no more than a passive transducer of information between DNA and protein synthesis. The catalytic role of RNA was highlighted in the early 1980s, and in 1986 the concept of “the RNA world” was proposed by Walter Gilbert,Citation4 whereby ribosomal and transfer RNA were described to have structural, enzymatic, and information-decoding roles in the process of protein synthesis.
In 1998, Fire et alCitation5 described the phenomenon of RNA interference (RNAi) for the first time. They discovered the ability of double-stranded RNA (dsRNA) to silence gene expression in the nematode worm, Caenorhabditis elegans. Three years later, Elbashir et alCitation6 opened up the field of RNAi applications in the treatment of human disease by demonstrating that synthetic small interfering RNA (siRNA) could knock down a gene in a sequence-specific manner in several mammalian cell lines. The in vivo therapeutic potential of this new technology for transgene expression was rapidly shown in mice by effective targeting of a sequence from hepatitis C virus.Citation7 Since then, there has been an explosion of interest in use of this technology for clinical applications throughout the scientific world, and many papers have been published in the field.Citation8–Citation12 siRNAs show promise for future control of diseases caused by activity of one or several genes. The list of human diseases which could potentially be treated using this approach is long, and includes viral infections,Citation13–Citation17 dominant genetic disorders,Citation18–Citation20 autoimmune disease,Citation21 and cancer.Citation9–Citation11,Citation22–Citation24
In the case of cancer therapy, the appeal of miRNAs is becoming par ticularly strong as the molecular mechanisms underlying tumorigenesis become better defined and various molecular targets are identified. These molecular targets afford a good chance to develop new drugs, in that siRNA molecules can, in theory, be designed to hit almost any target, including those that are difficult or impossible to address at present. Small RNAs are indeed being vigorously pursued as therapies, and development of siRNA therapeutics is now rapidly gaining momentum.
RNAi is one of the fastest advancing fields in biology. In 2004, only six years after the discovery of RNAi, the first siRNA-based agent developed as a treatment for wet age-related macular degeneration entered Phase I clinical trials in humans.Citation25,Citation26 However, recent research has highlighted the need to overcome important issues concerning the safety and feasibility of siRNA-based drugs before their clinical use, and a major advance has been made in this regard with the advent of nanomedicine technology. Because of their tunable features, nanoparticles are being investigated in an attempt to overcome the safety and delivery issues associated with siRNA-based drugs.Citation26,Citation27 In 2010, the first-in-human Phase I clinical trial was started,Citation28 in which siRNAs were systemically administered to patients with solid tumors using a targeted nanoparticle delivery system.
Given the exceptional promise of these combined technologies, this review aims to address fully the main strengths and weaknesses of their applications, highlighting the potential problems and solutions that one day could transform RNAi into a conventional treatment for human malignancy. First, we provide an overview of the mechanism for siRNA, focusing on the applications of siRNA-based cancer therapy. Second, we discuss the possible use of siRNA in the clinic, highlighting several challenges that could limit their applications, with particular emphasis on siRNA delivery and biological barriers. Third, we review the wide range of strategies proposed for siRNA delivery, with attention paid to the advantages and current limitations of the nanoparticle-based approach. Further, we discuss strategies which have shown in vivo success in humans, reporting the recent promising results from clinical trials in cancer and other diseases. Finally, we provide a critical evaluation of future prospects and challenges for siRNA-based therapy in the treatment of cancer.
Principles of siRNA-based cancer therapy
RNAi is a fundamental regulatory pathway for most eukaryotic cells. It consists of complex enzymatic machinery able to control post-transcriptional gene expression through homology-dependent degradation of target mRNA by siRNA, ie, nontranslated dsRNA molecules.Citation26,Citation27 siRNA molecules are 21–23 nucleotides in length with a characteristic and highly specific structure (2–3 nucleotide 3′ overhangs and 5′ phosphate and 3′ hydroxyl groups) to prevent erroneous gene silencing.Citation29 They also contain a sense (passenger) strand and an antisense (guide) strand.Citation30
In mammalian cells, RNAi is triggered by molecules of long dsRNA, which may be of exogenous or endogenous origin, and are cleaved into siRNA by Dicer, an RNase III endonuclease. Once cleaved, siRNAs interact withArgonaute-2, a multifunctional protein, and become incorporated into RNA-induced silencing complexes. Here the duplex RNA is unwound and Argonaute-2 degrades the “passenger” strand. The activated RNA-induced silencing complex is directed specifically to recognize a target by intermolecular base pairing throughout a single-stranded “guide” RNA molecule.Citation30,Citation31 Argonaute-2 then selectively hunts down and degrades the mRNA complementary to the antisense strand,Citation32 and endonucleolytic cleavage occurs between bases 10 and 11 relative to the 5′ end of the antisense siRNA strandCitation33–Citation36 (). This activated complex can propagate gene silencing further, destroying additional mRNA targets. This effect may last for 3–7 days in rapidly dividing cells and for many weeks in nondividing cells.Citation37 Translating this into practical terms, siRNA could be produced synthetically and directly introduced into target cells, thereby bypassing Dicer mechanics. Other forms of siRNA could be discussed, such as micro RNA (miRNA),Citation38 short hairpin RNA,Citation39,Citation40 and Piwi-interacting RNA.Citation41 However, siRNA is the most commonly used for RNAi in therapeutic applications.
Figure 1 RNAi/miRNA pathway schematization and major challenges for naked siRNA delivery in vivo. (A) Schematization of RNA interference (RNAi): non-translated double-stranded RNA (dsRNA) molecules called small interfering RNA (siRNA), of exogenous or endogenous origin, post-transcriptional regulate gene-expression through a sequence specific degradation of target messenger RNA (mRNA). [1] Longer siRNA molecules (dark green) are cleaved by the nuclease Dicer and [2] incorporated into a multiprotein RNA-inducing silencing complex (RISC). [2] The duplex RNA is unwound leaving the anti-sense strand (light green). [3] to guide RISC to complementary mRNA (red) for subsequent endonucleolytic cleavage and gene silencing. [4] Short hairpin RNAs (shRNA) (violet) are sequences of RNA encoded by specific genes; they are introduced into the nucleus, transcribed and transported into the cytoplasm where they follow the same fate of siRNA. (B) miRNA processing: microRNA (blue) are considered as the “endogenous substrate” of the RNAi machinery. They are trascribed by RNA-Pol III in long primary transcripts (pri-miR), then processed within the nucleus into precursor miRNA (pre-miRNA) by the RNase III enzyme Drosha-DGCR8. Pre-miRNA hairpins are exported from the nucleus in a process involving the nucleo-cytoplasmic shuttle Exportin-5 (Exp.5). In cytoplasm, the pre-miRNA hairpin is cleaved by Dicer and loaded into RISC as for siRNA. miRNAs often share only partial complementarity with target mRNAs, usually in the 3′UTR, acting mainly as a translational repressors. (C) “Naked” siRNA pitfalls. In the box are reported the major obstacles for therapeutic efficacy of siRNA without modifications (“naked”). See text for details.
![Figure 1 RNAi/miRNA pathway schematization and major challenges for naked siRNA delivery in vivo. (A) Schematization of RNA interference (RNAi): non-translated double-stranded RNA (dsRNA) molecules called small interfering RNA (siRNA), of exogenous or endogenous origin, post-transcriptional regulate gene-expression through a sequence specific degradation of target messenger RNA (mRNA). [1] Longer siRNA molecules (dark green) are cleaved by the nuclease Dicer and [2] incorporated into a multiprotein RNA-inducing silencing complex (RISC). [2] The duplex RNA is unwound leaving the anti-sense strand (light green). [3] to guide RISC to complementary mRNA (red) for subsequent endonucleolytic cleavage and gene silencing. [4] Short hairpin RNAs (shRNA) (violet) are sequences of RNA encoded by specific genes; they are introduced into the nucleus, transcribed and transported into the cytoplasm where they follow the same fate of siRNA. (B) miRNA processing: microRNA (blue) are considered as the “endogenous substrate” of the RNAi machinery. They are trascribed by RNA-Pol III in long primary transcripts (pri-miR), then processed within the nucleus into precursor miRNA (pre-miRNA) by the RNase III enzyme Drosha-DGCR8. Pre-miRNA hairpins are exported from the nucleus in a process involving the nucleo-cytoplasmic shuttle Exportin-5 (Exp.5). In cytoplasm, the pre-miRNA hairpin is cleaved by Dicer and loaded into RISC as for siRNA. miRNAs often share only partial complementarity with target mRNAs, usually in the 3′UTR, acting mainly as a translational repressors. (C) “Naked” siRNA pitfalls. In the box are reported the major obstacles for therapeutic efficacy of siRNA without modifications (“naked”). See text for details.](/cms/asset/9b1772a2-08da-4fc5-af9d-ad5016fd6fde/dijn_a_23696_f0001_c.jpg)
In certain cases, only partial complementarity can occur between siRNA and its target mRNA, leading to suppression of translation or destabilization of transcripts. This phenomenon usually causes the so-called off-target effects of siRNA (see later) and mimics interactions with target sites of another class of regulatory small RNA, ie, miRNA. These molecules are thought to be the endogenous substrate for the RNAi machinery, because endogenous siRNA has not been found in mammalian systems. miRNA molecules have been identified in mammals as well as in many other organisms. They derive from long primary transcripts (pri-miRNA), which are processed within the nucleus into precursor miRNA (pre-miRNA, 60–70 bp hairpins) by the RNase III enzyme, Drosha-DGCR8 (). They are then exported to the cytoplasm by Exportin-5, where they are further processed by the Dicer enzyme which removes the loop, and one of the two strands is loaded into the RNA-induced silencing complex.Citation38
Unlike siRNAs, mature miRNAs often share only par tial complementarity with sequences of target mRNAs, usually in the three prime untranslated regions. Therefore, the primary mechanism of action is translational repression, although miRNA molecules with full sequence complementarity can degrade mRNA, so it could be said that while siRNAs are able to control single gene expression fully, microRNAs can moderate the expression of gene networks.Citation38
The short hairpin RNA approach is based on nuclear delivery of a gene that encodes for desired short hairpin RNAs. These are transcribed, transported to the cytoplasm via the miRNA machinery, and processed into siRNAs by Dicer, as mentioned earlier. The need for the short hairpin RNA gene to enter the nucleus adds complexity to the delivery and reduces the potency of siRNA gene silencing. On the other hand, the potential advantage of DNA-based RNAi is its stable introduction into cells as gene therapy, bypassing transient effects, so avoiding repeated or continuous administration in the clinical setting.Citation39,Citation40
Another variation of siRNA-based therapy is administration of longer precursors processed by Dicer endonuclease. Recent reports indicate that these precursors are several nucleotides longer and are much more powerful than the siRNAs widely used for inducing target gene silencing.Citation42 This is probably because direct coupling of Dicer processing and the Argonaute-2 loading mechanism of siRNA is much more efficient.Citation42
RNAi and cancer: therapeutic implications
Cancer is a disease that is often characterized by mutations in multiple signal transduction pathways, leading to uncontrolled cell proliferation. It is usually difficult to identify the key genes governing cell proliferation or survival which should be targeted to induce cell death. RNAi technology can be useful for cancer therapy because of its high efficacy and specificity in downregulating gene expression, and it is clearly important to understand the role of altered genes in the development of cancer. It must be stressed that blockage of a single gene is not sufficient to cure or control most cancers. One of the major likely benefits of the RNAi technique in the treatment of cancer is the feasibility of developing combination therapeutic RNAi approaches to inhibit multiple oncogenes or genes of proteins involved in tumorigenesis.Citation43 A correct approach could involve hitting multiple pathways simultaneously and/ or combining gene silencing with other therapies.
Many in vitro and in vivo studies have evaluated the silencing of genes involved in pathways that drive cancer, such as oncogenesis, apoptosis, cell cycle regulation, cell senescence, tumor-host interaction, and resistance to conventional therapies.Citation9,Citation43,Citation44 In addition, because miRNAs may work as both tumor suppressors and oncogenes, these molecules could also be investigated as therapeutic targets, in that they could be knocked down when expressed in excess or replaced in cells when they have been pathologically reduced or lost.Citation45 Deregulated miRNA could also serve as a helpful biomarker for diagnosis and monitoring of disease.Citation46 Biomarkers are easily and objectively measurable biological characteristics which can be used as indicators of normal and pathological processes. Notably, miRNAs can be detected in body fluids, such as blood and cerebrospinal fluid, enabling noninvasive and early detection of disease. A further example of the potential applications of miRNA is expression profiling in order to distinguish cancer from normal tissue and to classify, eg, cancer subtypes or the origin of metastatic cancer tissue.Citation46
siRNA in the clinic
Difficulties in therapeutic application
As discussed, siRNAs hold promise as therapeutics in view of their ability to silence any gene with a known sequence. However, effective and well controlled in vivo delivery is challenging, and presently limits the use of RNAi in the clinicCitation27 (see ). In fact, these molecules, without any other modifications (“naked”), encounter several obstacles that reduce their therapeutic efficacy (). To be efficacious, they need to reach the cytoplasm of the cell in sufficient amounts to enable sustained target inhibition.Citation47,Citation48 However, naked siRNAs are highly unstable intravascularly, with a short half-life due to their susceptibility to serum RNAse A-type nucleases and rapid renal clearance.Citation47–Citation49 Moreover, unmodified siRNA molecules are not able to enter most cells readily because of their size (13 kDa) and highly polyanionic nature.Citation47,Citation48,Citation50 Once in the cells, their effects are transient and diluted at each cell division,Citation51 so repeated dosing is required to achieve long-term therapeutic effects. Unmodified siRNA molecules also have potential toxicities,Citation52,Citation53 via three main mechanisms, ie, saturation of RNAi machinery and competition within the miRNA pathway, stimulation of the immune response, and off-target effects.
Table 1 Problems of Naked siRNA for clinical applications
Saturation of RNAi machinery and competition in miRNA pathway
RNAi is an important regulatory mechanism in the cell which could be perturbed by exogenous introduction of dsRNA. The components of the cellular machinery involved in gene silencing could be less accessible to miRNAs entering the natural cellular pathway.Citation47,Citation54,Citation55 Intact RNAi machinery is clearly essential for mammalian cells, as suggested by the early embryonic lethality of Dicer knockouts.
Stimulation of immune response
Some of the sequence motifs in siRNA molecules could have the ability to induce the innate immune response. The innate immune response is mediated principally by type I interferon and proinflammatory cytokines,Citation56–Citation58 and can be triggered in different ways, ie, mediated or not mediated by the Toll-like receptor (TLR). Briefly, when nucleic acids enter endosomal and lysosomal compartments, TLR-3, 7, and 8 are primed and activate interferon-alpha and inflammatory cytokines via nuclear translocation of the nuclear factor k-light chain enhancer of activated B cells.Citation59 The innate immune response that is not mediated by TLR is triggered principally by cytoplasmic RNA sensors, including the retinoic acid-inducible gene 1 and dsRNA-binding protein kinase. This mechanism can lead to activation of interferon-beta and other inflammatory mediators.
Off-target effects
siRNA was initially described to be specific for the target gene. However, early genome-wide monitoring of gene activity in siRNA-treated cells disproved its almost ideal specificity.Citation60,Citation61 SiRNA shows miRNA-like off-target silencing of a large number of unintended transcripts with partial identity to its sequence.Citation60,Citation61 This mechanism has unpredictable cellular consequences, with important toxic phenotypic effects. Specific research efforts have been made to understand better and control these undesirable off-target effects and their long-term implications, and even more so now, considering the possible clinical reality of siRNA.Citation47,Citation62 These difficulties are likely to be less pronounced when therapy is localized to the more accessible organs or tissues, eg, eye, skin, and mucus membranes. In these contexts, siRNA therapy has higher bioavailability and may have fewer of the adverse effects associated with nonspecific siRNA delivery.
The ideal route and obstacles which can be encountered by molecules introduced into the human body through systemic delivery are still a matter of debate. Much research effort using in vivo tracking and fluorescent methods is presently focused on these issues. It is generally accepted that, after intravenous injection, the molecules travel throughout the whole body, navigate the circulatory system, in which they need to avoid renal filtration, are taken up by phagocytes (both in the bloodstream and in the extracellular matrix tissue) and aggregate with serum proteins ().Citation50 They have to cross biological barriers, ie, pass into the bloodstream and across vascular endothelial walls to be able to reach the target organ or tissue. Specifically, it has been reported that molecules larger than 5 nm in diameter do not readily cross the capillary endothelium, and therefore remain in the body if they are not cleared. However, larger molecules up to 200 nm in diameter are able to enter some tissues, including the spleen, liver, and several types of tumor, due to their different vasculature. The molecules must also diffuse through the extracellular matrix, which consists of a dense network of polysaccharides and fibrous proteins. The extracellular matrix is also rich in macrophages, which can obstruct transport of macromolecules and nanoparticles. The siRNA complex must then be taken up by target cells, at which point they have to evade the endosome system to reach the cytoplasm.Citation63 Through a complex system of cell compartments with decreasing pH, the molecules can reach lysosomes to be degraded. Finally, to be effective, siRNA must be released from its carrier to reach the cellular machinery.Citation50
Figure 2 Encapsulation technologies for siRNA delivery in vivo: nanoparticles strategies and advantages.
Readapted from Shim et al.Citation165
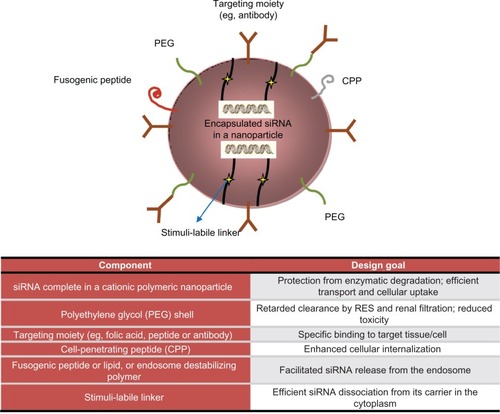
Strategies for siRNA delivery: advantages and current limitations
Early delivery strategies and advent of nanotechnology
Early on, siRNA therapeutics were administered locally using naked or chemically modified siRNA.Citation64 As already described, the complexity of physiological interactions, both biochemical and mechanical, immediately signaled the necessity to protect gene material and guide it to its target site of action. Preliminary approaches to protect siRNA arose from DNA transfection techniques. Generally speaking, these protective strategies can be divided in three categories, ie, mechanical, chemical, and biological. Mechanical approaches seek to enhance permeability of biological barriers, and in this way allow gene material to reach the cellular environment. The main mechanical permeabilization techniques are sonoporation and electroporation. Chemical and biological approaches, on the other hand, aim to transport genetic cargo through biological systems within a “Trojan horse” system. In this context, viral vectors provide the most efficient delivery, but their use is limited due to the host immune response and the inner complexities of this method. State-of-the art chemical approaches are the most promising, as a result of ever-increasing insight into techniques of synthesis and the biological behavior of nonbiological structures. Observations from clinically approved nanoparticle formulations suggest that lipidic carriers (Doxil®) and covalent coupling with albumin (Abraxane®)Citation65 are viable strategies for siRNA delivery. However, bioavailability remains an unresolved issue because these vectors are unable to stabilize and protect siRNA in the biological environment.
Nanotechnology has been a most promising spinoff in molecular medicine. In this field, technical advances have suddenly accelerated towards more refined fabrication techniques and new materials with unique and innovative features.Citation66,Citation67 Starting from this technological platform, new possibilities and different points of view are coming to light which may resolve the present issues in drug delivery and remove the barriers to discovery of the “magic bullet” for cancer.Citation68,Citation69,Citation68,Citation69 Thus, a concrete opportunity to change current treatment regimens in a substantial way is offered by nanoparticles, which are nanostructured entities with tunable size, shape, surface, and biological behavior. The impact of nanostructured therapeutics in terms of efficacy and safety is promising to be revolutionary.
The dawn of nanotechnology, seen as the manipulation of matter on a nanometric scale, allows the design and fabrication of novel devices with uniquely tailored physicochemical properties and enormous therapeutic and diagnostic potential. Over the past two decades, there has been a steady rise in the number of commercially available nanoparticle therapeutics.Citation69 The first example of a nanostructure with biological application was the lipidic vesicle (liposome), a spherical self-assembled layer of amphiphilic lipidic molecules of nanometric size. Liposomes became used as drug delivery systems, because of their circumscribed inner volume which can be used to contain and transport a drug to its target site of action. Chemical surface modifications, such as poly(ethylene) glycol (PEG), have been developed to increase bioavailability. Limiting factors to their successful use as carriers remain, especially concerning their stability in biological environments and their ability to transport drugs across biological barriers. In the following sections, we briefly highlight the advantages of nanoparticles which make them suitable for integration into siRNA technology. A broad overview is also provided of almost all delivery strategies that have been attempted to date, underlining the eventual limitations and pitfalls.
Nanoparticles
Nanoparticles are physical entities with a characteristic length of 1–100 nm. They can be considered as a unit in term of physicochemical properties. In nanotechnology, the synthesis and characterization of these objects is a broad field in constant development, heading towards “smart” structures with superior capabilities in various scientific and technological contexts, including catalysis, energy conversion, sensors, and actuators.Citation70,Citation71 The most promising and rapidly growing applications of nanoparticles are directly linked to biomedical issues, with cellular trafficking and accurate in vitro and in vivo tracking of biological systems being two examples of the new frontiers opened up for biological study by the application of luminescent inorganic nanoparticles.Citation72
Nanoparticles can be categorized as organic or inorganic according to the bulk constituent materials in their structure. Classifications based on size, shape, surface, structure, and chemical behavior can be devised. Overall, what is particularly attractive about nanostructures is their surface to volume ratio. Nanosized entities enable a very limited volume to provide an enormous surface area for transport, chemical reactions, and interaction with biological systems. These features, combined with enhanced permeability to biological barriers, make nanoparticles the basis of nanomedicine and a powerful and promising tool in the design of new diagnostic and therapeutic devices.
Interest in nanoparticles has consistently grown in every branch of medicine. It has been driven by the potential of nanoparticles to achieve a high therapeutic index and corresponding clinical success with improved patient compliance and reduced side effects.Citation73 Nanoparticle-based drug delivery systems play an important role in the development and future applications of new pharmaceutical formulations. They may be capable of improving bioavailability and reducing the frequency and dosage needed for currently used drugs.Citation74,Citation167 With this novel type of drug formulation, in which nanoparticles act as drug carriers, it may be possible to: improve drug stability and carrier capacity; target drug molecules to a specific diseased cell or tissue, avoiding toxicity to normal cells; confine release of the drug to the site of interest; allow efficient permeation of epithelial and endothelial barriers; and combine therapies with codelivery of two or more drugs.Citation68,Citation75–Citation77 The latter could involve incorporation of a wide range of molecules, including both hydrophilic and hydrophobic substances, thereby improving the delivery of poorly water-soluble drugs.
Residence time in the circulation, maximal tolerated dose, and selectivity are the most important factors to consider when determining the optimal dosage.Citation73 In drug delivery systems, the choice of bulk material constituent for the carrier is a key point, and tailored biological behavior (bioactivity, biocompatibility, biodegradability) must be fulfilled, with an improved payload capacity. It is important that the nanomaterial carrier is able to be eliminated harmlessly from the body in a reasonable period of time after releasing its cargo, and, in case of theranostic nanoparticles, accomplishing a desired diagnostic function.Citation78 The drug could be adsorbed, dissolved, or dispersed throughout the matrix of the nanoparticles, or, alternatively, it can be covalently attached to the surface or inside the matrix. In the body, the drug loaded in nanoparticles is usually released from the matrix by diffusion, swelling, erosion, or degradation. Two kinds of targeting strategies are possible, ie, passive targeting and active targeting. Passive targeting does not require surface modification of the nanoparticles, and is achieved using the unique pathophysiology of diseased cells and controlling particle mobility through human tissues, according to particle size and route of administration. On the other hand, active targeting can be achieved by surface modification of nanoparticles and their functionalization with ligands or antibodies that can recognize and bind to complementary molecules or receptors found on the surface of specific cells.Citation79
A further advance in drug delivery is represented by the multistage delivery system,Citation80 which provides more accurate spatiotemporal targeting with efficient cargo release. Nanoparticles suitable for multistage drug delivery systems are hybrid nanostructures (for instance, with a core-shell structure) designed to reach injured tissues in a selective manner.Citation81,Citation82 Each part of the hybrid nanoparticle responds differently in the biological environment. These features, combined with conventional targeting against upregulated cell surface antigens, allow precise spatiotemporal drug delivery in selected areas of the host system. Furthermore, in the design of nanocarriers, it is possible to achieve stimuli-responsive behavior using pH-sensitive and/or temperature-sensitive materials.Citation83 These carriers hold promise for realizing effective spatiotemporal delivery of drugs. In this sense, nanoparticles are designed to be part of a delivery system and it is necessary to consider the overall behavior of such systems, ie, carrier, payload, and host system properties, as well as mutual interactions. In a gene delivery context, a key feature of the carrier is the ability to protect the gene payload until it reaches the target site, with no modification in molecular structure of the gene or its biochemical activity.
This review explores strategies for siRNA delivery by nanoparticles. The pursuit of gene delivery has been undertaken using viral-basedCitation84 and nonviral-basedCitation85,Citation86 vectors. These topics have already been extensively reviewed,Citation87,Citation88 so are not discussed in depth in this paper. Nanoparticle features in drug delivery are likely to overcome a number of issues concerning therapeutic applications of siRNA. The challenge of effective and nontoxic delivery is a crucial point and remains the most significant barrier to therapeutic application of siRNA technology.Citation89 Moreover, nanoparticles can be engineered to have controlled release of functional siRNA. As discussed above, slow, sustained, and controlled release could be helpful for decreasing the frequency of treatment and lead to more effective therapies, especially in siRNA therapeutics.
In order to achieve effective siRNA delivery, several strategies have been studied. Leaving apart the viral vector delivery setup, we consider here the chemical modifications of siRNA, ligand-based (targeted or conjugated) siRNA, polymers, cationic and neutral liposomes, sensu stricto nanoparticles, and combined approaches. A short description and a few examples of each of these strategies is presented (see ), and a more detailed revision can be found elsewhere.Citation42
Table 2 Delivery strategies for siRNA. Advantages and pitfalls
Chemical modifications to siRNA
Various chemical modifications have been introduced to increase the in vivo metabolic stability of siRNA molecules less than 10 nm in size. Examples of such modifications and their advantages (without affecting the efficiency of RNAi) are listed below and have been reviewed extensively elsewhere:Citation47,Citation48,Citation62,Citation90–Citation93
2′-O-Methyl modifications in the ribose structure of selected nucleotides within both sense and antisense strands
Introduction of phosphorothioate backbone linkages at the 3′ end of the RNA strands
Alternative 2′ sugar modifications (eg, fluorine substitution).
These chemical modifications, added to the sugars, backbone, or bases of dsRNA, improve intravascular stabilization and are able to reduce activation of the innate immune response, without significant loss of RNAi activity.Citation51,Citation59
Ligand-based targeting molecules
Among the chemical modifications, 3′ or 5′ modifications of siRNA deserve separate consideration. These can be useful for improving resistance to degradation, and also for introducing targeting or conjugating ligands less than 10 nm in size, such as peptides and aptamers. Indeed, the smallest siRNA nanoparticles derive from direct conjugation of small molecules, peptides, or polymers to the sense strand of siRNA. These modifications of the sense strand seem not to affect mRNA degradation by siRNA. Based on the additional features attributed to siRNA molecules, these further modifications can be classified as ligand-targeted (affecting target specificity) or ligand-conjugated (mostly affecting stability).
Ligand-targeted siRNAs
Terminal modification (5′ or 3′) of siRNA molecules using cholesterol is a useful strategy for increasing their stability and cellular uptake. In particular, it increases binding to serum albumin, with consequent improved biodistribution in certain target tissues, eg, the liver. The improvement in cellular uptake is mediated by in vivo interaction and incorporation into low-density and high-density lipoproteins. Cholesterol-modified siRNA are capable of silencing apolipoprotein B targets in the mouse liver and jejunum, and of ultimately reducing total cholesterol levels.Citation18,Citation94 However, although this type of chemical modification has improved siRNA delivery to tissues, it is often associated with impaired biological activity and increased toxicity.Citation62,Citation92,Citation93 In addition, siRNA can be conjugated with other lipid-like molecules, such as long-chain or medium-chain fatty acids and bile salt derivatives. These interact with high-density and low-density lipoprotein receptors, enhancing delivery into the liver and gene silencing in vivo. Moreover, albumin serves as a primary carrier for siRNA conjugated to medium-chain fatty acids.Citation42
One particular modification is that achieved by mipomersen, a 2′-O-(2-methoxyethyl)-modified single-stranded RNA molecule targeted to apolipoprotein B, frequently implicated in cardiovascular disease. Many promising Phase II trials have been reported in this regard, with encouraging safety and efficacy results.Citation95 A Phase III trial assessing the efficacy of mipomersen is currently ongoing in patients with a family history of hypercholesterolemia.
The folate receptor is a membrane glycoprotein over-expressed in several human tumors, including ovarian, colorectal, and breast cancer, but is minimally present in normal tissues. This feature makes it an attractive target for drug delivery. Folate-mediated targeting has several advantages, including the small size of folic acid, its lack of immunogenicity, convenient availability, and easy chemical conjugation.Citation42,Citation96
Like the folate receptor, the transferrin receptor is a glycoprotein frequently overexpressed by tumor cells. The intracellular routing of transferrin receptor-mediated endocytosis has been well characterized, and this molecule is easily available. For this reasons, transferrin receptor-mediated delivery has been extensively explored in a variety of targets, including tumors, endothelial cells, and the brain.Citation42 Nanoparticles are usually conjugated chemically to either transferrin itself or to antitransferrin receptor antibodies.Citation42,Citation52,Citation97
Antibodies have also been used extensively in the treatment of cancer. Because of their high specificity and affinity for cancer cell antigens, they have the capacity to induce an immune response against target cancer cells. Antibodies have also been recognized as “delivery molecules”. Several studies have demonstrated antibody-targeted delivery of drugs and nucleic acids. Antibodies or their fragments can be used as targeting agents for nanoparticles. Among the successful in vivo demonstrations, are antibody-protamine fusions that bind siRNA.Citation98 Several problems remain to be resolved for effective application of this strategy, in particular the potential for inducing immunogenicity and the high cost of the antibodies.Citation42
Along with antibodies, aptamersCitation99 have been used for site-specific delivery of siRNA because of their high affinity and specificity for targets, with the advantages of a much smaller size (<15 kDa versus 150 kDa for antibodies). For instance, prostate-specific membrane antigen is a cell surface receptor usually detected at abnormal levels in prostate cancer cells and in tumor vascular endothelium, and has been proposed for targeting siRNA to these sites. Delivery of siRNA mediated by aptamers is promising, albeit limited by some challenges that need to be investigated further, in particular, the pharmacokinetics and biodistribution of these molecules.Citation42
Ligand-conjugated siRNAs
To increase in vivo transfer, siRNA can be conjugated with other molecules, eg, peptides or PEG, which are able to overcome steric hindrance, or linked with drugs or nucleic acids and nanoparticles. Cell-penetrating peptides, protein transduction domains, and membrane translocation sequences are counted among the peptides.Citation42 For example, cell-penetrating peptides are able to cross the cell membrane, with the advantages of increased target gene knockdown in vitro and in vivo.Citation100,Citation101 In the case of PEG, introduction of sugar molecules, eg, cyclodextrin,Citation97 and hyaluronic acidCitation102–Citation104 is the most common approach for preventing drug aggregation because of the surface charge of siRNA or other loaded drugs, which typically have a net positive charge.Citation105 The benefits of this charge neutralization are increased circulation time for the compound and enhanced targeting through limiting nonspecific interactions between the positively charged siRNA drug and the negatively charged cell membrane. Overall, these mechanisms result in increased target gene knockdown in vitro and in vivo.Citation50
Polymers are organic macromolecules that protect RNA from degradation and facilitate its sustained delivery to tissues. Many different types of polymers, varying in size, chemistry, and pharmacological properties, can be considered. Cationic polymersCitation62,Citation87,Citation88,Citation106–Citation109 can be useful as transfection agents, given that they can bind one or more large nucleic acids, package them reversibly into stabilized nanoparticles, and protect them against a degrading bioenvironment. These vectors avoid enzymatic degradation by nucleic acids by forming condensed complexes along with targeted delivery to cells and tissue.Citation110 Moreover, these compounds have demonstrated an ability to stimulate nonspecific endocytosis. Another useful feature for siRNA delivery is endosomal escape, whereby certain molecules, eg, polyethylenimine and cyclodextrin, lead to local accumulation of ions via a mechanism called the “proton-sponge” effect, and as a consequence, the osmotic pressure generated bursts the endosomal compartment in which they are contained. Cationic polymers are generally divided into synthetic and natural polymers, with a linear or branched structure. Synthetic polymers include branched or linear polyethylenimine,Citation111 poly-L-lysine, and cyclodextrin-based polycations. On the other side, natural cationic polymers include chitosan, atelocollagen, and cationic polypeptides. Another advantage of cationic polymer-based delivery is the ease with which siRNA and polymer complexes can be formulated due to their opposite charges. In fact, cationic polymers usually form a complex with negatively charged siRNA upon simple mixing. Despite these advantages, appreciable cytotoxicity (both necrosis and apoptosis) is a major problem with the use of these compounds.Citation112 However, cationic vectors with diminished cytotoxicity and enhanced efficacy are rapidly emerging as delivery systems of choice.Citation113,Citation114 A possible application of cationic polymers is their conjugation in more complex compounds such as polymeric nanoparticles. These molecules can be defined by the morphology and polymer composition in their core and coronaCitation73,Citation110 (). The therapeutic load (in this case siRNA) is usually conjugated to the surface of the nanoparticle or encapsulated and protected inside the core. Delivery systems composed of these compounds can be engineered to enable controlled or triggered release of therapeutic molecules.Citation73 Furthermore, the nanoparticle surface may be functionalized by various methods to form a corona, which can provide increased residence time in blood, reduced nonspecific distribution, and in some cases, target tissues or specific cell surface antigens with a targeting ligand. For example, PEG allows steric stabilization or prevention of protein absorption. The final result is avoidance of opsonization and rapid clearance from bloodstream due to phagocytosis in the liver or spleen. As described, this delivery strategy combines the protective effect and loading capacity of the polymer with the specificity of an active targeting strategy through chemical molecular conjugation. During the past few years, several papers have reported delivery of nucleic acids mediated by cationic polymer carriersCitation113,Citation114 and described the generation of precise polymers, site-specific conjugation strategies, and multifunctional conjugates for nucleic acid transport.Citation115,Citation116
Lipid vectors
Also important in the field of delivery systems based on organic molecule carriers are lipid vectors, such as liposomes and cationic lipids.
Liposomes
Liposomes are compounds characterized by a phospholipid bilayer and an aqueous core. They can be created from single or multiple types of lipid. Unilamellar and multilamellar liposomes are commonly used as vehicles for delivery of pharmaceuticals. Liposomes interact with siRNAs to form complexes stabilized by electrostatic interactions.Citation48,Citation92 These are the delivery methods that are currently most mature and closest to clinical application, given that the platform has been adopted in clinical trials performed by several leading RNAi companies.Citation117,Citation118 However, some disadvantages must be overcome before they can be used in vivo. Liposomes tend to accumulate in the reticuloendothelial system, leading to rapid clearance by the liver and a short half-life in serum, so require either continuous infusion or frequent administration. Liposomes also lack target tissue specificity and have reduced access to other tissues. A possible approach to circumvent this problem is to develop sustained-release polymer formulations.Citation107 For example, one group of polar head units can create the outer surface of the nanocomplex, while the other group of polar head units faces the interior hydrophilic core, which holds the nucleic acid payload (). It is also possible to construct amorphous structures with liposomes, in which lipids and nucleic acids alternate with each other. The additional flexibility of these molecules can optimize the physical and chemical properties of the nanoparticle.
Neutral nanoliposomes
To avoid the potential toxicity associated with other delivery systems,Citation112 neutral liposomes 30–40 nm in diameter which encapsulate siRNA are commonly being used. Unilamellar liposomes, such as dioleoyl phosphatidylcholine,Citation119,Citation120 with a hydrophilic core and a hydrophobic surface are able to protect siRNA from degradation by surrounding endonucleases and can enhance internalization through membrane fusion or receptor-mediated endocytosis.Citation121
Cationic lipids
Cationic lipids 100–300 nm in size are able to protect siRNA from degradation by nucleases and increase the circulating half-life and uptake by cells. Several cationic lipids have been synthesized for formulation with siRNA, and have been evaluated in preclinical studies or animal models. However, they show significant cell toxicity and elicit hypersensitivity reactions in vivo. Liposomes in general and cationic lipids [DOTAP and DOTMA (N-[1-(2,3-dioleyloxy) propyl]-N, N, N-trimethyl ammonium chloride] in particular are able to induce strong type I and II interferon responses.Citation122 They show dose-dependent toxicity, and pulmonary inflammation can arise as a result of reactive oxygen intermediates.Citation123 Chemical additives could improve their formulation and reduce cell toxicity, thus making liposomes safer. In particular, liposomal nanoparticles comprise electrostatic complexes of nucleic acids and cationic lipids, such as dioleoyl trimethylammonium propane and N,N-dimethylaminopropane carbamoyl cholesterol.
A modern class of biodegradable solid lipid nanoparticles has also been developed. These nanoparticles are used to incorporate various drugs or imaging agents, with the benefit of using physiological and nontoxic lipids that remain solid at body temperature.Citation124,Citation125 Stable nucleic acid lipid particles (SNALPs) are a major advance in lipid-based siRNA delivery. A proof-of-concept study of the effectiveness of SNALP-siRNA therapeutics has been reported by Geisbert et al.Citation126 In this recent work, siRNA was targeted to interfere with the expression of three Ebola virus proteins. This strategy was used successfully to protect nonhuman primates from lethal Ebola virus infection.
In the SNALP-siRNA panorama, it is worth mentioning a study by Morrissey et alCitation17 which suggested that hepatitis B virus replication could be inhibited by delivery of an siRNA-SNALP complex targeting hepatitis B virus RNA. Finally, in very recent work by Lobovkina et alCitation126 solid lipid nanoparticles were developed for sustained in vivo siRNA delivery in a mouse model. Even in this case, preparing nanoparticles from physiological lipids resulted in excellent biocompatibility, minimal toxicity, and less cost when compared with polymeric carriers.Citation127
Nanoparticles and microspheres have also been developed as gene delivery vehicles. These are promising strategies because they afford improved siRNA delivery and stability, with minimal toxicity in animal models.Citation47,Citation108 They can be engineered to obtain target-specific delivery, for example, by tagging nanoparticle-siRNA oligonucleotide complexes with antibodies that bind the desired target cells. On the other hand, direct injection of synthetic oligonucleotides into solid tumors can be done for some neoplasms, such as mesothelioma (by intrapleural injection), ocular tumors, brain tumors, and sarcomas, and could reduce or eliminate off-target effects.Citation62,Citation106,Citation108 Some companies are exploring these encapsulation technologies. Using this approach, oligonucleotides are sequestered in various kinds of nanoparticles to protect them from degradation and to direct them to appropriate tissues. The idea behind such an approach is that the nanoparticle is seen merely as a carrier, and thus the functions of the vector are to protect, stabilize, and transport the gene material, with the minimum of interaction. Here we give an overview of the main nanoparticles proposed for siRNA delivery and investigation, starting from inorganic crystals, noble metal nanoparticles, and other nanostructures (see ).
Table 3 Nanoparticles in siRNA-based delivery
Quantum dots are semiconducting inorganic crystals with superior photostability and tunable optical properties for an extensive selection of nonoverlapping colors.Citation134 They have no bioactivity, and in certain cases, depending on the bulk material used, have no toxicity but a low payload capacity. These features make quantum dots basically useless in designing delivery systems, but they are a powerful tool in cancer targeting, imaging in living animals, and investigation of pathophysiology in tumor tissue.Citation135
Incorporation of siRNA into gold nanoparticles was first accomplished by Oishi et al.Citation137 Further advances in the use of gold particles as carriers have included creation of a supramolecular structure in a layer-by-layer assemblyCitation138 and particle surface chemical modification.Citation139,Citation140 Even though the reduced size provides a large surface area for the loading of siRNA-nanoparticle conjugates, chemical modification of both the carrier surface and the transported drug is required. To overcome such problems, new delivery strategies based on porous siliconCitation80 have been developed.
New combination approaches
Multistage delivery systems show promise for meeting the challenges of targeting and overcoming biological barriers. Numerous, chemically different, core-shell nanoparticles with cationic cores and variable shells have been synthesized and tested for intracellular siRNA delivery. In general, this strategy enables tools for nanoparticle delivery to be equipped with the necessary targeting moieties, such as antibodies, aptamers, and small peptides, for direct delivery and improved specificity. These nanoparticles have been engineered to act in a depot manner, resulting in slow, sustained, and targeted release of siRNA. The majority of biodegradable formulations of this kind have used polymeric materials in which siRNA is incorporated in a polymeric core.Citation146–Citation149
Researchers have recently constrained siRNA between a cationic core composed of DOTAP and an outer lipid bilayer of 1,2-distearoyl-sn-glycero-3-phosphoethanolamine-N-(polyethylene glycol-2000) and egg phosphatidylcholine obtaining a bloodstream circulation time of up to 20 hours after injection.Citation150
A novel approach has been proposed by Tanaka et alCitation144 involving a multistage delivery system composed of two biodegradable and biocompatible carriers. The first-stage carriers are mesoporous, microscale, biodegradable silicon particles that enable loading and release of the second-stage nanocarriers, ie, dioleoyl phosphatidylcholine nanoliposomal siRNA. In vivo mouse models provided the first evidence that single administration of multistage siRNA-dioleoyl phosphatidylcholine delivery resulted in sustained in vivo gene silencing for 3 weeks, with a significant antitumor effect in two orthotopic mouse models of ovarian cancer, and no observable concurrent toxicity.
Current limitations of nanoparticle-siRNA delivery
Nanoparticle-based drug delivery still has some limitations, and the barriers and obstacles encountered by nanoparticle-siRNA complexes are schematized in (adapted from WhiteheadCitation50). First of all, these vehicles must reach and enter target cells. The biological barriers encountered are the mucosa and the cellular and humoral arms of the immune system. These molecules tend to be sequestered by negatively charged serum proteins in the bloodstream. Moreover, immune cells can collect them via opsonization.
Figure 3 Obstacles of Nanoparticles-based siRNA delivery in vivo. After administration into blood circulation the siRNA-nanoparticles (A) must avoid rapid degradation by plasma components (eg, cellular and humoral arm of the immune system) and sequester by negatively charged serum protein. (B) Then they need to escape renal filtration and/or clearance by the reticuloendothelial system (RES). (C) To reach the target cells they must overcome the capillary endothelium through an extravasation process and (D) overcome the extracellular matrix (ECM): a dense network of polysaccharides and fibrous proteins, rich in macrophages, which can obstacle the transport of nanoparticles. (E) Furthermore these particles must be taken up into the cells, usually bound to cellular receptors and transported into the cytoplasm through a receptor mediated endocytosis process. (F) Inside the cells the particles need to escape the endosome; (G) thus unpackage and release the siRNA to the RNA interference (RNAi) machinery.
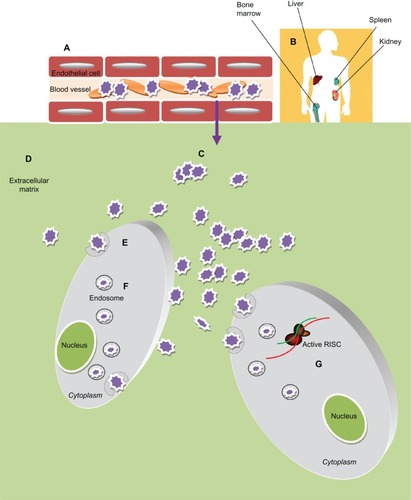
Nanoparticles can be engineered in terms of size, surface functionalization, and core structuresCitation151 to overcome these barriers. Indeed, addition of PEG or other hydrophilic conjugates to the surface of a delivery vehicle can reduce protein sequestration, help in evading the immune system, provide steric stabilization, and protect against the effects of the surrounding microenviroment. These nanocomplexes generally undergo rapid clearance.Citation152 The reticuloendothelial system in the liver, spleen, lung, and bone marrow is able to trap intravenously injected particles >100 nm in diameter, leading to their degradation by activated monocytes and macrophages. On the other hand, particles with a diameter <4 nm usually undergo rapid renal clearance. Thus, the optimal diameter of nanoparticles for intratumoral delivery, avoidance of the reticuloendothelial system, and renal clearance should be in the range of 5–100 nm.
Some tumor characteristics can be exploited for drug delivery. The enhanced permeability and retention effect takes advantage of the abnormal neovascularization typical of many cancers.Citation73 This effect is often found in tumors as well as at sites of inflammation and body tissue with other types of disease. The blood vessels are either disrupted or not fully formed, allowing molecules of a certain size to be retained to a greater extent in abnormal tissues than in healthy ones. It has been shown that therapeutic nanoparticles can accumulate in specific target tissues as a result of this effect, potentially allowing for drug release over a longer period of time than for the same drug administered as a conventional preparation.
Conversely, limitations can arise from the intricate tumor microenvironment and interfere with achieving therapeutic concentrations of these molecules. The higher interstitial fluid pressure found in solid tumor tissue could prevent diffusion of nanoparticles.Citation153,Citation154 Strategies adopted to overcome this issue could include normalizing the tumor vasculature (eg, with antiangiogenic therapy) and using nanoparticles with a diameter larger than that of normal vessel fenestrations. The molecules must also face the extracellular matrix, and often the desmoplastic process in cancer creates difficulties in this respect.
Once inside the target cells, the nanoparticles must enter the intracellular trafficking pathway. Many nanoparticles enter cells through endocytosis, and the endosomal vesicles containing RNAi must escape the late endosomal phase in the lysosome that degrades RNA. Fusogenic lipids, fusogenic peptides, photosensitive molecules, pH-sensitive lipoplexes, and pH-sensitive polyplexes are some of the mechanisms used to improve endosomal escape.Citation155
Nanoparticles have often been reported to have systemic toxicity, mostly in the liver.Citation144 Hemolysis, thrombogenicity, and complement activation can also occur as a consequence of certain physical or chemical features of nanoparticles, eg, charge and size, leading to altered biodistribution and potential toxicity.Citation156 Furthermore, most of the current methods used for RNAi delivery require frequent injectionsCitation119,Citation120 which could represent a great hindrance to their clinical application due to decreased patient compliance.
In the oncology field, given the heterogeneity of tumors, development of resistance seems inevitable. Furthermore, some cancers could have inherent resistance to some types of RNAi, owing to factors such as ethnicity, somatic mutations, altered RNAi processing machinery (Dicer, Drosha, RNA-induced silencing complex), and germline single nucleotide polymorphisms.Citation27
Ongoing clinical trials of siRNA in cancer
The clinical utility of RNAi is still a matter of debate. There has been increasing interest in harnessing this versatile and multifaceted mechanism as a novel pharmacological approach to the treatment of human disease. As has already happened in other developing human therapeutic fields, including gene and antibody therapy, there has been a cooling off after initial enthusiasm. This was driven by a realistic understanding of the various complex milestones that needed to be reached for efficient RNAi-based therapy, before eventual approval for use in human therapy. This trend is now reversing as a result of the excitement generated by the advent of nanomedicine as a potential way of overcoming the challenges in this field. In recent years, there has been an increase in the numbers of preclinical and clinical RNAi-based trials being undertaken. Early proof-of-principle studies in animal models have strengthened the usefulness of RNAi as specific and powerful inhibitors of gene expression without significant toxicity. Translation of animal studies has in some cases progressed to early human trials.
In particular, the research interest is strongest when the aim is targeting genes with single nucleotide polymorphism mutations, as found in dominant-negative disorders, genes specific for pathogenic tumor cells, and genes that are critical for mediating the pathology of various other diseases. In these cases, RNAi could represent the only entities providing an opportunity for a potent and specific approach.Citation157
The relevant clinical trials have addressed many kinds of disease, including retinal degeneration, dominantly inherited brain and skin diseases, viral infections, respiratory disorders, cancer, and metabolic disease). However, large-scale Phase III clinical trials and regulatory approvals remain distant. Use of RNAi for respiratory tract and neurological disorders, metabolic disease (such as hypercholesterolemia), and hepatic cancer has been widely revised in recent times.Citation158 Most of the more advanced clinical trials focus on the treatment of age-related macular degeneration, which is a leading cause of blindness arising from excessive growth of blood vessels and their rupture within the cornea. Of note, a recent study has showed that the efficacy of antivascular endothelial growth factor siRNA in the eye is not due to specific gene silencing, but is actually caused by nonspecific stimulation of the TLR-3 pathway, which can reduce angiogenesis.Citation59
Another important success has been achieved with a formulation targeting the nucleocapsid (N) gene of respiratory syncytial virus, a major cause of respiratory disease in infants and young children. An RSV01 formulation (ALN-RSV01) has completed Phase I investigations and was found to be well tolerated in healthy adults. In July 2009, the complete data from a Phase II study in adult lung transplant patients naturally infected with RSV were reported, documenting the significant antiviral efficacy, safety, and tolerability of this formulation. At this time, a Phase IIb study of ALN-RSV01 for the treatment of respiratory syncytial virus infection is ongoing in adult lung transplant patients. This study aims to repeat and extend the results already seen in this patient population.Citation13,Citation159
Another successful study is one of the siRNA, TD101.Citation160,Citation161 In this prospective, double-blind, split-body, vehicle-controlled, dose-escalation trial, TD101 was administered over a 17-week period to a single patient affected by pachyonychia congenita. This molecule specifically and potently targets keratin 6a N171K mutant mRNA without affecting wild-type keratin 6a mRNA. No adverse events were reported during the trial or during a 3-month washout period. This study was an example of siRNA application in a clinical setting to target a mutant gene or a genetic disorder, and the first to use siRNA targeted to human skin.
Recent reports have discussed other RNAi clinical trials in detail.Citation43,Citation157,Citation158,Citation162 Various clinical trials are in progress, involving many companies.Citation163 We were able to find further as yet unpublished clinical trials registered at http://www.clinicaltrials.gov. Using the keyword “siRNA”, 24 trials were identified and are reported in . Among these, nine contained the keywords “siRNA and cancer therapy”. Although many hindrances remain for applying these technologies in the treatment of cancer, early clinical results are exciting, and suggest that we are moving forward quickly and also highlight how far we have already come.
Table 4 Ongoing clinical trials in cancer and other diseases
An example of the clinical feasibility of siRNA application for cancer therapy is represented by chronic myeloid leukemia. In more than 90% of cases, this disease is caused by a chromosomal aberration, ie, the so-called Philadelphia chromosome. This abnormality is due to fusion between the Abelson (Abl) tyrosine kinase gene at chromosome 9 and the breakpoint cluster (Bcr) gene at chromosome 22, resulting in the chimeric oncogene Bcr-Abl and a constitutively active Bcr-Abl tyrosine kinase implicated in the pathogenesis of chronic myeloid leukemia. On the basis of these observations, an attempt was made to exploit RNAi for inhibition of tumor-specific genes to hit cancer cells in a selective manner. To this end, the Bcr-Abl clinical trial addressed safety and efficacy issues that have now been published.Citation162,Citation164,Citation165 This clinical trial treated only one patient with recurrent Philadelphia chromosome-positive chronic myeloid leukemia by systemic administration of a nonvirally delivered synthetic siRNA against Bcr-Abl. However, effective knockdown of the target gene in circulating leukemic cells was difficult to assess due to concomitant treatment.Citation162 The findings of this study imply that the clinical application of synthetic siRNA is feasible and safe, and has real potential for a genetic-based therapy using a synthetic nonviral carrier.
In 2010, Davis et alCitation28 undertook the first proof-of-principle study of encapsulation technology in human patients. In this small Phase I clinical trial, nanoparticles packed with siRNA were administered systemically on days 1, 3, 8, and 10 every 21 days via a 30-minute intravenous infusion to patients with melanoma refractory to standard of care. This new “drug” was able to deliver its cargo to melanoma cells and to silence the targeted gene.
The nanoparticles used in this study are a paradigm of all that has been discussed to this point, in that they are comprised of separate components encapsulating RNA, targeting specific cell types, favoring stability, and avoiding aggregation, each of which is highly engineered and optimized. These nanoparticles contain a cyclodextrin-based polymer, a human transferrin protein to engage transferrin receptors on the surface of the cancer cells, PEG to promote stability of the nanoparticles in biological fluids, and siRNA. The results provide the first evidence of the importance of RNAi in humans, indicating that siRNA can be used as a gene-specific therapeutic agent.Citation28
Conclusion and future prospects
In just over a decade, RNAi technology has progressed rapidly from an academic discovery to a potential new class of treatment for human disease. Initial observations that were useful for studying gene function in worms were quickly translated to other organisms, and in particular to mammals, revealing the potential clinical applications of siRNA, including an ability to induce potent, persistent, and specific silencing of a wide range of genetic targets. Early excitement was soon dampened by the hurdles and concerns about full exploitation of the power of the RNAi pathway. These include the need to: identify strategies to select potent siRNA with specificity for the target gene, thus minimizing off-target effects; reduce immune stimulation and competition with cellular RNAi components; and enhance effective in vivo delivery to the appropriate cells or tissues by manipulating biopharmaceutical properties. Many of these issues have already been addressed, and others are being addressed at the moment.
The advent of nanomedicine has represented a milestone, contributing strongly towards a solution to problems often encountered in the clinical setting. Recently, nanoparticle-based delivery systems have been shown to have potent RNAi effects after systemic administration. However, their delivery remains one of the most significant obstacles to the widespread use of RNAi therapeutics in the clinical setting, so further progress in this area is needed. To this end, strategies encompassing multiple delivery technologies including novel conjugations and formulations are being explored. Looking forward, synthetic nanoparticles will likely have a fundamental role in the systemic application of siRNA in the clinic. The conjugation of tissue-specific ligands with these particles will enable targeting, and support in vivo biodistribution and delivery.
Tremendous progress has made in exploiting the biological and physical features of cancer to enhance the use of nanoparticles in this disease. The ability of nanoparticles to overcome biological barriers, recognize cancerous versus normal tissue, and deliver therapeutic RNAi is a key determinant of the therapeutic index. In this regard, researchers must also take into account the practical considerations of patient compliance with repeated dosing. Due to the polygenic nature of cancer, the efficacy and specificity of antitumor treatment will probably be further enhanced by using a combination approach of siRNA with chemotherapy, radiotherapy, photodynamic therapy, and/or immunotherapy. It is anticipated that siRNA and nanotechnology will lead the next wave of drug development. In the future, nanoparticles containing siRNA will gain importance as a conventional treatment for cancer and other human diseases.
Acknowledgements
This work was supported in part by the Consorzio Sapienza Innovazione, Associazione Italiana Ricerca Cancro, Ministero dell’Istruzione, dell’Università e della Ricerca (MIUR), Fondo per gli Investimenti della Ricerca di Base (FIRB), Progetti di Ricerca di Interesse Nazionale (PRIN) projects, the Istituto Italiano di Tecnologia (IIT) and European Framework Program 7 (EU-FP7) Initial Training Network (ITN) project n. 238186.
Disclosure
The authors declare that they have no conflicts of interest in this work.
References
- DrukerBGuilhotFO’BrianSFive-year follow-up of patients receiving imatinib for chronic myeloid leukemiaN Engl J Med20063552408241717151364
- GoldenbergMMTrastuzumab, a recombinant DNA derived humanized monoclonal antibody, a novel agent for the treatment of metastatic breast cancerClin Ther1999230931810211534
- PetersonCDrug therapy of cancerEur J Clin Pharmacol20116743744721336995
- GilbertWThe RNA worldNature1986319216
- FireAXuSMontgomeryMKKostasSADriverSEMelloCCPotent and specific genetic interference by double-stranded RNA in Caenorhabditis elegansNature19983918068119486653
- ElbashirSMHarborthJLendeckelWYalcinAWeberKTuschlTDuplexes of 21-nucleotide RNAs mediate RNA interference in cultured mammalian cellsNature200141149449811373684
- McCaffreyAPMeuseLPhamTTConklinDSHannonGJKayMAGene expression: RNA interference in adult miceNature2002418383912097900
- WallNRShiYSmall RNA: can RNA interference be exploited for therapy?Lancet20033621401140314585643
- DeviGRsiRNA-based approaches in cancer therapyCancer Gene Ther20061381982916424918
- AbdelrahimMSafeSBakerCAbudayyehCRNAi and cancer: implications and applicationsJ RNAi Gene Silencing2006213614519771215
- GartelALKandelESRNA interference in cancerBiomol Eng200623173416466964
- GrimmDKayMARNAi and gene therapy: a mutual attractionHematol Am Soc Hematol Educ Program2007473481
- DeVincenzoJLambkin-WilliamsRWilkinsonTA randomized, double-blind, placebo-controlled study of an RNAi-based therapy directed against respiratory syncytial virusProc Natl Acad Sci U S A20101078800880520421463
- SubramanyaSKimSSManjunathNShankarPRNA interference-based therapeutics for human immunodeficiency virus HIV-1 treatment: synthetic siRNA or vector-based shRNA?Expert Opin Biol Ther2010220121320088715
- ChenYChengGMahatoRIRNAi for treating hepatitis B viral infectionPharm Res20081728618074201
- AshfaqUAYousafMZAslamMEjazRJahanSUllahOsiRNAs: potential therapeutic agents against hepatitis C virusVirol J2011827621645341
- MorrisseyDVLockridgeJAShawLPotent and persistent in vivo anti-HBV activity of chemically modified siRNAsNat Biotechnol2005231002100716041363
- DiFigliaMSena-EstevesMChaseKTherapeutic silencing of mutant huntingtin with siRNA attenuates striatal and cortical neuropathology and behavioral deficitsProc Natl Acad Sci U S A2007104172041720917940007
- FarahMHRNAi silencing in mouse models of neurodegenerative diseasesCurr Drug Deliv2007416116717456035
- LiTKoshySFolkessonHGRNA interference for CFTR attenuates lung fluid absorption at birth in ratsRespir Res200895518652671
- CourtiesGPresumeyJDuroux-RichardIJorgensenCApparaillyFRNA interference-based gene therapy for successful treatment of rheumatoid arthritisExpert Opin Biol Ther2009953553819392574
- MartinezLANaguibnevaILehrmannHSynthetic small inhibiting RNAs: efficient tools to inactivate oncogenic mutations and restore p53 pathwaysProc Natl Acad Sci U S A200299148491485412403821
- PaiSILinYYMacaesBMeneshianAHungCFWuTCProspects of RNA interference therapy for cancerGene Ther20061346447716341059
- TakeshitaTOchiyaTTherapeutic potential of RNA interference against cancerCancer Sci20069768969616863503
- WhelanJFirst clinical data on RNAiDrug Discov Today2005101014101516055013
- CastanottoDRossiJJThe promises and pitfalls of RNA-interference-based therapeuticsNature200945742643319158789
- PecotCVCalinGAColemanRLLopez-BeresteinGSoodAKRNA interference in the clinic: challenges and future directionsNat Rev Cancer201111596721160526
- DavisMEZuckermanJEChoiCHEvidence of RNAi in humans from systemically administered siRNA via targeted nanoparticlesNature20104641067107020305636
- HammondSMDicing and slicing the core machinery of the RNA interference pathwayFEBS Lett20055795822582916214139
- MatrangaCTomariYShinCBartelDPZamorePDPassenger-strand cleavage facilitates assembly of siRNA into Ago2-containing RNAi enzyme complexesCell200512360762016271386
- ToliaNHJoshua-TorLSlicer and the ArgonautesNat Chem Biol20073364317173028
- AmeresSLMartinezJSchroederRMolecular basis for target RNA recognition and cleavage by human RISCCell200713010111217632058
- RandTAPetersenSDuFWangXArgonaute2 cleaves the anti-guide strand of siRNA during RISC activationCell200512362162916271385
- DykxhoornDMLiebermanJKnocking down disease with siRNAsCell200612623123516873051
- NovinaCDSharpPAThe RNAi revolutionNature200443016116415241403
- LingelASattlerMNovel modes of protein-RNA recognition in the RNAi pathwayCurr Opin Struct Biol20051510711515718141
- BartlettDWDavisMEInsights into the kinetics of siRNA-mediated gene silencing from live-cell and live-animal bioluminescent imagingNucleic Acids Res20063432233316410612
- GarzonRMarcucciGCroceCMTargeting microRNAs in cancer: rationale, strategies and challengesNat Rev Drug Discov2010977578920885409
- BrummelkampTRBernardsRAgamiRA system for stable expression of short interfering RNAs in mammalian cellsScience200229655055311910072
- RötherSMeisterGSmall RNAs derived from longer non-coding RNAsBiochimie2011931905191521843590
- GhildiyalMZamorePDSmall silencing RNAs: an expanding universeNat Rev Genet2009109410819148191
- YuBZhaoXLeeLJLeeRJTargeted delivery Systems for oligonucleotide therapeuticsAAPS J20091119520319296227
- ChenSHZhaoriGPotential clinical applications of siRNA technique: benefits and limitationsEur J Clin Invest20114122123220964680
- MasieroMNardoGIndraccoloSFavaroERNA interference: implications for cancer treatmentMol Aspects Med2007114316617307250
- BakerMHoming in on deliveryNature201046212251228
- De SmaeleEFerrettiEGulinoAMicroRNAs as biomarkers for CNS cancer and other disordersBrain Res2010133810011120380821
- AagaardLRossiJJRNAi therapeutics: principles, prospects and challengesAdv Drug Deliv Rev200759758617449137
- ZhaoXPanFHoltCMLewisALLuJRControlled delivery of antisense oligonucleotides: a brief review of current strategiesExpert Opin Drug Deliv2009667368619552611
- BumcrotDManoharanMKotelianskyVSahDWYRNAi therapeutics: a potential new class of pharmaceutical drugsNat Chem Biol2006271171917108989
- WhiteheadKALangerRAndersonDGKnocking down barriers: advances in siRNA deliveryNat Rev Drug Discov2009212913819180106
- KimDHRossiJJStrategies for silencing human disease using RNA interferenceNat Rev Genet2007317318417304245
- HeidelJDYuZLiuJYAdministration in non-human primates of escalating intravenous doses of targeted nanoparticles containing ribonucleotide reductase subunitM2 siRNAProc Natl Acad Sci U S A20071045715572117379663
- DesaiAASchilskyRLYoungAA phase I study of antisense oligonucleotide GTI-2040 given by continuous intravenous infusion in patients with advanced solid tumorsAnn Oncol2005695896515824081
- GrimmDStreetzKLJoplingCLFatality in mice due to over-saturation of cellular microRNA/short hairpin RNA pathwaysNature200644153754116724069
- CastanottoDSakuraiKLingemanRCombinatorial delivery of small interfering RNAs reduces RNAi efficacy by selective incorporation into RISCNucleic Acids Res2007355154516417660190
- HornungVGuenthner-BillerMBourquinCSequence-specific potent induction of IFN-α by short interfering RNA in plasmacytoid dendritic cells through TLR7Nat Med20051126327015723075
- JudgeADSoodVShawJRFangDMcClintockKMacLachlanISequence-dependent stimulation of the mammalian innate immune response by synthetic siRNANat Biotechnol20052345746215778705
- GantierMPWilliamsBRThe response of mammalian cells to double-stranded RNACytokine Growth Factor Rev20071836337117698400
- KleinmanMEYamadaKTakedaASequence- and target-independent angiogenesis suppression by siRNA via TLR3Nature200845259159718368052
- JacksonALBartzSRSchelterJExpression profiling reveals off-target gene regulation by RNAiNat Biotechnol20032163563712754523
- JacksonALBurchardJSchelterJWidespread siRNA “off-target” transcript silencing mediated by seed region sequence complementarityRNA2006121179118716682560
- DiasNSteinCAAntisense oligonucleotides: basic concepts and mechanismsMol Cancer Ther2002134735512489851
- OliveiraSvan RooyIKranenburgOStormGSchiffelersRFusogenic peptides enhance endosomal escape improving siRNA-induced silencing of oncogenesInt J Pharm200733121121417187949
- SchroederALevinsCGCortezCLangerRAndersonDGLipid-based nanotherapeutics for siRNA deliveryJ Intern Med201026792120059641
- MieleESpinelliGPMieleETomaoFTomaoSAlbumin-bound formulation of paclitaxel (Abraxane ABI-007) in the treatment of breast cancerInt J Nanomedicine200949910519516888
- De AngelisFGentileFMecariniFBreaking the diffusion limit with super-hydrophobic delivery of molecules to plasmonic nanofocusing SERS structuresNat Photonics20115682687
- PujiaADe AngelisFScumaciDHighly efficient human serum filtration with water-soluble nanoporous nanoparticlesInt J Nanomedicine201051005101521187942
- FarokhzadOCLangerRImpact of nanotechnology on drug deliveryACS Nano20093162019206243
- FerrariMCancer nanotechnology: opportunities and challengesNat Rev Cancer200556171
- WongMSNanoparticles and catalysisAstrucDidierAngewandte Chemie International EditionWeindham, GermanyWiley2008
- KnöpfelTAkemannWNanobiotechnology: remote control of cellsNat Nanotechnol20108560561
- MichaletXPinaudFFBentolilaLAQuantum dots for live cells, in vivo imaging, and diagnosticsScience200530753854415681376
- AlexisFPridgenEMolnarLKFarokhzadOCFactors affecting the clearance and biodistribution of polymeric nanoparticlesMol Pharm2008550551518672949
- GlassGPharmaceutical patent challenges – time for reassessment?Nat Rev Drug Discov200431057106215573104
- PeerDKarpJMHongSFarokhzadOCMargalitRLangerRNanocarrier as an emerging platform for cancer therapyNat Nanotechnol2007275176018654426
- WeislederRKimberlyKYi SunEShtalandTJosephsonLCell-specific targeting of nanoparticles by multivalent attachment of small moleculesNat Biotechnol2005231418142316244656
- ByrneJDBetancourtTBrannon-PeppasLActive targeting schemes for nanoparticle systems in cancer therapeuticsAdv Drug Deliv Rev2008601615162618840489
- ParkJi-HoGuLvon MaltzahnGRuoslahtiEBhatiaSNSailorMJBiodegradable luminescent porous silicon nanoparticles for in vivo applicationsNat Mater2009833133619234444
- JanibSMMosesASMacKayJAImaging and drug delivery using theranostic nanoparticlesAdv Drug Deliv Rev2010621052106320709124
- TasciottiELiuXBhavaneRMesoporous silicon particles as a multistage delivery system for imaging and therapeutic applicationNat Nanotechnol20083152157
- ChanJMZhangLTongSpatiotemporal controlled delivery of nanoparticles to injured vasculatureProc Natl Acad Sci U S A20101072213221820133865
- SenguptaSEavaroneDCapilaITemporal targeting of tumor cells and neovasculature with a nanoscale delivery systemNature200543656857216049491
- BigallNCCurcioAPernia LealMPMagnetic nanocarriers with tunable pH dependence for controlled loading and release of cationic and anionic payloadsAdv Mater2011235645565022095932
- WaehlerRRussellSJCurielDTEngineering targeted viral vectors for gene therapyNat Rev Genet2007857358717607305
- MintzerMASimanekEENonviral vectors for gene deliveryChem Rev200910925930219053809
- GaoYLiuXLLiXRResearch progress on siRNA delivery with nonviral carriersInt J Nanomedicine201161017102521720513
- KimWJKimSWEfficient siRNA delivery with non-viral polymeric vehiclesPharm Res20092665766619015957
- SchaffertDWagnerEGene therapy progress and prospects: synthetic polymer-based systemsGene Ther2008151131113818528432
- TokatlianTSeguraTsiRNA applications in nanomedicineNanomed Nanobiotechnol20102305315
- ChiuYLRanaTMsiRNA function in RNAi: a chemical modification analysisRNA200391034104812923253
- BehlkeMAProgress towards in vivo use of siRNAsMol Ther20061364467016481219
- MalikRRoyLDesign and development of antisense drugsExp Opin Drug Discov2008311891207
- ChiarantiniLCerasiAFraternaleAComparison of a novel delivery system for antisense peptide nucleid acidsJ Control Release2005109243616290244
- SoutschekJAkincABramlageBTherapeutic silencing of an endogenous gene by systemic administration of modified siRNAsNature200443217317815538359
- RaalFDSantosRDBlomDJMipomersen, an apolipoprotein B synthesis inhibitor, for lowering of LDL cholesterol concentrations in patients with homozygous familial hypercholesterolaemia: a randomised, double-blind, placebo-controlled trialLancet2010375998100620227758
- ZhaoXLiHLeeJRTargeted drug delivery via folate receptorsExpert Opin Drug Deliv2008530931918318652
- Hu-LieskovanSHeidelJDBartlettDWDavisMETricheTJSequence-specific knockdown of EWS-FLI1 by targeted, nonviral delivery of small interfering RNA inhibits tumor growth in a murine model of metastatic Ewing’s sarcomaCancer Res2005658984899216204072
- SongEZhuPLeeSKAntibody mediated in vivo delivery of small interfering RNAs via cell-surface receptorsNat Biotechnol20052370971715908939
- McNamaraJO2ndAndrechekERWangYCell type-specific delivery of siRNAs with aptamer-siRNA chimerasNat Biotechnol2006241005101516823371
- MuratovskaAEcclesMRConjugate for efficient delivery of short interfering RNA (siRNA) into mammalian cellsFEBS Lett2004558636814759517
- HatakeyamaHItoEAkitaHA pH-sensitive fusogenic peptide facilitates endosomal escape and greatly enhances the gene silencing of siRNA-containing nanoparticles in vitro and in vivoJ Control Release200913912713219540888
- BartlettDWSuHHildebrandtIJWeberWADavisMEImpact of tumor-specific targeting on the biodistribution and efficacy of siRNA nanoparticles measured by multimodality in vivo imagingProc Natl Acad Sci U S A2007104155491555417875985
- PirolloKFChangEHTargeted delivery of small interfering RNA: approaching effective cancer therapiesCancer Res2008681247125018316585
- LeeHMokHLeeSOhYKParkTGTarget specific intracellular delivery of siRNA using degradable hyaluronic acid nanogelsJ Control Release200711924525217408798
- RozemaDBLewisDLWakefieldDHDynamic polyconjugates for targeted in vivo delivery of siRNA to hepatocytesProc Natl Acad Sci U S A2007104129821298717652171
- ZuhornISEngbertsJBHoeckstraDGene delivery by cationic lipid vectors: overcoming cellular barriersEur Biophys J20073634936217019592
- ChirilaTVRakoczyPEGarrettKLLouXConstableIJThe use of synthetic polymers for delivery of therapeutic antisense oligodeoxynucleotidesBiomaterials20022332134211761152
- RosiNLGiljohannDAThaxtonCSLytton-JeanAKHanMSMirkinCAOligonucleotide-modified gold nanoparticles for intracellular gene regulationScience20063121027103016709779
- ConvertineAJBenoitDSDuvallCLHoffmanASStaytonPSDevelopment of a novel endosomolytic diblock copolymer for siRNA deliveryJ Control Release200913322122918973780
- WagnerEPolymers for siRNA delivery: inspired by viruses to be targeted, dynamic, and preciseAcc Chem Res20112210.1021/ar2002232
- Urban-KleinBWerthSAbuharbeidSCzubaykoFAignerARNAi-mediated gene-targeting through systemic application of polyethylenimine (PEI)-complexed siRNA in vivoGene Ther20051246146615616603
- OhYKParkTGsiRNA delivery systems for cancer treatmentAdv Drug Deliv Rev20096185086219422869
- NimeshSGuptaNChandraRCationic polymer based nanocarriers for delivery of therapeutic nucleic acidsJ Biomed Nanotechnol2011750452021870455
- SinghaKNamgungRKimWJPolymers in small-interfering RNA deliveryNucleic Acid Ther20112113314721749290
- TroiberCWagnerENucleic acid carriers based on precise polymer conjugatesBioconjug Chem2011221737175221749135
- DuncanRPolymer therapeutics as nanomedicines: new perspectivesCurr Opin Biotechnol20112249250121676609
- SempleSCAkincAChenJRational design of cationic lipids for siRNA deliveryNat Biotechnol20102817217620081866
- GuoPCobanOSneadNMEngineering RNA for targeted siRNA delivery and medical applicationAdv Drug Deliv Rev20106265066620230868
- MerrittWMLinYGSpannuthWAEffect of interleukin-8 gene silencing with liposome-encapsulated small interfering RNA on ovarian cancer cell growthJ Natl Cancer Inst200810035937218314475
- LandenCNJrChavez-ReyesABucanaCTherapeutic EphA2 gene targeting in vivo using neutral liposomal small interfering RNA deliveryCancer Res2005656910691816061675
- DharmapuriSPeruzziDMarraEIntratumor RNA interference of cell cycle genes slows down tumor progressionGene Ther20111872773321390070
- MaZLiJHeFWilsonAPittBLiSCationic lipids enhance siRNA-mediated interferon response in miceBiochem Biophys Res Commun200533075575915809061
- DokkaSToledoDShiXCastranovaVRojanasakulYOxygen radical-mediated pulmonary toxicity induced by some cationic liposomesPharm Res2000175215252155
- MehnertWMäderKSolid lipid nanoparticles: production, characterization and applicationsAdv Drug Deliv Rev20014716519611311991
- GallarateMBattagliaLPeiraETrottaMPeptide-loaded solid lipid nanoparticles prepared through coacervation techniqueInternational Journal of Chemical Engineering201116
- GeisbertTWLeeACRobbinsMPostexposure protection of non-human primates against a lethal Ebola virus challenge with RNA interference: a proof-of-concept studyLancet20103751896190520511019
- LobovkinaTJacobsonGBGonzalez-GonzalezEIn vivo sustained release of siRNA from solid lipid nanoparticlesACS Nano201159977998322077198
- KimSHJeongJHLeeSHKimSWParkTGPEG conjugated VEGF siRNA for anti-angiogenic gene therapyJ Control Release20061161239
- BoussifOLezoualc’hFZantaMAA versatile vector for gene and oligonucleotide transfer into cells in culture and in vivo: polyethylenimineProc Natl Acad Sci U S A199592729773017638184
- ZimmermannTSLeeACHAkincARNAi-mediated gene silencing in non-human primatesNature200644111111416565705
- AkincAGoldbergMQinJDevelopment of lipidoid-siRNA formulations for systemic delivery to the liverMol Ther20091787287919259063
- AkincAZumbuehlAGoldbergMA combinatorial library of lipid-like materials for delivery of RNAi therapeuticsNat Biotechnol20082656156918438401
- VaishnawAKGollobJGamba-VitaloCA status report on RNAi therapeuticsSilence201011420615220
- ChenAADerfusAMKhetaniSRBhatiaSNQuantum dots to monitor RNAi delivery and improve gene silencingNucleic Acids Res20053319015647502
- GaoXCuiYLevensonRMChungLWKNie1SIn vivo cancer targeting and imaging with semiconductor quantum dotsNat Biotechnol20042296997615258594
- YezhelyevMVQiLO’ReganRMNieSGaoXProton-sponge coated quantum dots for siRNA delivery and intracellular imagingJ Am Chem Soc20081309006901218570415
- OishiMNakaogamiJIshiiTNagasakiYSmart PEGylated gold nanoparticles for the cytoplasmic delivery of siRNA to induce enhanced gene silencing ChemLett20063510461047
- ElbakryAZakyALieblRRachelRGoepferichABreunigMLayer-by-layer assembled gold nanoparticles for siRNA deliveryNano Lett200952059206419331425
- LeeJSGreenJJLoveKTSunshineJLangerRAndersonDGGold, poly(beta-amino ester) nanoparticles for small interfering RNA deliveryNano Lett200962402240619422265
- Lytton-JeanALangerRAndersonDGFive years of siRNA delivery: spotlight on gold nanoparticlesSmall201171932193721681985
- MedarovaZPhamWFarrarCPetkovaVMooreAIn vivo imaging of siRNA delivery and silencing in tumorsNat Med2007337237717322898
- CurcioAMarottaRRiedingerAPalumberiDFalquiAPellegrinoTMagnetic pH-responsive nanogels as multifunctional delivery tools for small interfering RNA (siRNA) and iron oxide nanoparticles (IONPs)Chem Commun (Camb)2012482400240222266784
- XiaTKovochichMLiongMPolyethyleneimine coating enhances the cellular uptake of mesoporous silica nanoparticles and allows safe delivery of siRNA and DNA constructsACS Nano200933273328619739605
- TanakaTMangalaLSVivas-MejiaPESustained small interfering RNA delivery by mesoporous silicon particlesCancer Res2010703687369620430760
- KamNWLiuZDaiHFunctionalization of carbon nanotubes via cleavable disulfide bonds for efficient intracellular delivery of siRNA and potent gene silencingJ Am Chem Soc2005127124921249316144388
- LeeSYangSCKaoCYPierceRHMurthyNSolid polymeric microparticles enhance the delivery of siRNA to macrophages in vivoNucleic Acids Res200937145
- JacobsonGBShindeRMcCulloughRLNanoparticle formation of organic compounds with retained biological activityJ Pharm Sci2010992750275520039390
- KrebsMDJeonOAlsbergELocalized and sustained delivery of silencing RNA from macroscopic biopolymer hydrogelsJ Am Chem Soc20091319204920619530653
- PatilYPanyamJPolymeric nanoparticles for siRNA delivery and gene silencingInt J Pharm200936719520318940242
- YagiNManabeITottoriTA nanoparticle system specifically designed to deliver short interfering RNA inhibits tumor growth in vivoCancer Res2009696531653819654315
- PetrosRADeSimoneJMStrategies in the design of nanoparticles for therapeutic applicationsNat Rev Drug Discov2010961562720616808
- LongmireMChoykePLKobayashiHClearance properties of nanosized particles and molecules as imaging agents: considerations and caveatsNanomedicine (Lond)2008570371718817471
- DecuzziPCausaFFerrariMNettiPAThe effective dispersion of nanovectors within the tumor microvasculatureAnn Biomed Eng2006463364116568349
- LiLWilcoxDZhaoXTumor vasculature is a key determinant for the efficiency of nanoparticle-mediated siRNA deliveryGene Ther201116
- DominskaMDykxhoornDMBreaking down the barriers: siRNA delivery and endosome escapeJ Cell Sci20101231183118920356929
- DobrovolskaiaMAAggarwalPHallJBMcNeilSEPreclinical studies to understand nanoparticle interaction with the immune system and its potential effects on nanoparticle biodistributionMol Pharm2008448749518510338
- SeyhanAARNAi: a potential new class of therapeutic for human genetic diseaseHum Genet201113058360521537948
- DavidsonBLMcCrayPBCurrent prospects for RNA interference-based therapiesNat Rev Genet20111232934021499294
- Alnylam PharmaceuticalsAlnylam achieves first human proof of concept for an RNAi therapeutic with GEMINI study Available from: http://www.alnylam.com/Programs-and-Pipeline/Partner-Programs/index.php. Accessed April 17, 2012.
- LeachmanSAHickersonRPHullPRTherapeutic siRNAs for dominant genetic skin disorders including pachyonychia congenitaJ Dermatol Sci20085115115718495438
- LeachmanSAHickersonRPSchwarzMEFirst-in-human mutation targeted siRNA phase Ib trial of an inherited skin disorderMol Ther20101844244619935778
- GondiCRaoJSConcepts in in-vivo siRNA delivery for cancer therapyJ Cell Physiol200922028529119391103
- PtasznikANakataYKalotaAEmersonSGGewirtzAMShort interfering RNA (siRNA) targeting the Lyn kinase induces apoptosis in primary, and drug resistant, BCR-ABL1+ leukemia cellsNat Med2004101187118915502840
- KoldehoffMSteckelNKBeelenDWElmaagacliAHTherapeutic application of small interfering RNA directed against bcr-abl transcripts to a patient with imatinib-resistant chronic myeloid leukaemiaClin Exp Med20077475517609876
- ShimMSKwonYJEfficient and targeted delivery of siRNA in-vivoFEBS J20102774814481721078116
- VaccariLCantonDZaffaroniNPorous silicon as drug carrier for controlled delivery of doxorubicin anticancer agentMicr Eng20068315981601
- GentileFTirinatoLBattistaECells preferentially grow on rough substratesBiomaterials2010317205721220637503
- GaspariMMing-Cheng ChengMTerraccianoRNanoporous surfaces as harvesting agents for mass spectrometric analysis of peptides in human plasmaJ Proteome Res200651261126616674117