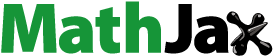
Abstract
Introduction
The current radiofrequency ablation technique requires invasive needle placement. On the other hand, most of the common photothermal therapeutic methods are limited by lack of accuracy of targeting. Gold and magnetic nanoparticles offer the potential to heat tumor tissue selectively at the cellular level by noninvasive interaction with laser and radiofrequency.
Methods
Gold nanospheres and gold-coated magnetic nanocomposites were used for inducing hyperthermia to treat subcutaneous Ehrlich carcinoma implanted in female mice.
Results
In mice treated with gold nanospheres, tumors continued to grow but at a slow rate. In contrast, more than 50% of the tumors treated with gold-coated magnetic nanocomposites completely disappeared.
Conclusion
This simple and noninvasive method shows great promise as a technique for selective magnetic photothermal treatment.
Introduction
Hyperthermia is under consideration currently as a noninvasive approach to cancer therapy, whereby biological tissues are exposed to higher than normal temperatures to promote selective destruction of abnormal cells.Citation1 In the mid 1970s, growing interest in conventional hyperthermia as a cancer treatment modality led to the development of techniques such as whole body hyperthermia, local body hyperthermia, and regional hyperthermia. These modalities were developed for oncology applications and investigation of the efficacy of hyperthermia in cancer treatment.Citation2 The available hyperthermic techniques for cancer therapy have low spatial selectivity in heating of tumors and surrounding healthy tissue.Citation3
The emergence of nanotechnology and nanoscience has led to the development and investigation of metallic nanostructure surfaces or materials for biomedical applications. Various nanotechnologies have been investigated for phototherapy, including magnetite nanoparticles, titanium oxide nanoparticles, and illumination with ultraviolet A irradiation.Citation4 Metallic nanostructures, such as half nanoshells (ie, nanospheres coated with silver), have previously been developed as a platform for surface-enhanced Raman scattering detection in gene diagnostics and cellular imagingCitation5 using surface-enhanced Raman scattering. One of the ways to improve laser spatial heating selectivity is photothermal labeling of tumor tissue by gold nanoparticles with different shapes and structures, such as nanoshells,Citation6 nanorods,Citation7 nanocages,Citation8 and others.Citation9,Citation10 By exposing nanoparticles to laser radiation near their plasmon-resonant absorption band, it is possible to produce local heating of nanoparticle-labeled cells without harming surrounding healthy tissues. Such an approach has been developed over the last 5 years and is known as plasmonic photothermal therapy.Citation11
Gold nanoshells belong to a prospective class of optical adjustable nanoparticles with a dielectric silica core encased in a thin metallic gold shell.Citation12 The absorption cross-section of a solid nanoshell is high enough to provide a competitive nanoparticle technology with application of indocyanine green dye, a typical photothermal sensitizer used in laser cancer therapy.Citation13,Citation14 There are several papers on in vitro experiments using gold nanoparticles in plasmonic photothermal therapy for cancer cells, but in vivo and ex vivo studies are quite limited.Citation15 There remain several areas to be studied more thoroughly. First of all, there is the question of controlled and localized hyperthermia without significant overheating of both the tumor and surrounding normal tissues. Precise control over local temperature distribution is the key factor to be considered in the context of enhanced plasmonic photothermal therapy efficacy. Laser heating can result in tumor necrosis, apoptosis, and accelerated tumor growth, depending on the accuracy of heating and rise in tumor temperature on illumination with laser light. Specifically, heating up to 39°C–45°C may lead to acceleration of biological reactions accompanied by production of heat shock proteins and vigorous growth of the tumor.Citation16–Citation18 This study evaluated two different approaches in the nanotechnology era for inducing hyperthermia in subcutaneous Ehrlich carcinoma cells.
Materials and methods
All chemicals used in this study were analytical grade reagents and used without further purification. Preparation of 5 × 103 mol/L of gold nanospheres was accomplished using the citrate reduction route in the following manner. In brief, HAuCl4 0.1699 g was added to 100 mL of double-distilled water, producing a faint yellowish solution; 5 mL of this solution was then added to 90 mL of double-distilled water. The solution was heated until boiling point, and 5 mL of 0.5% sodium citrate was added to the boiling solution with vigorous magnetic stirring. Heating was continued for another 15 minutes until the color of the solution gradually changed to a wine-red color. The solution was made up to 100 mL using double-distilled water, stirred for a further 15 minutes, and then stored at 4°C.
Preparation of nanocomposites
Firstly, magnetic nanoparticles were prepared according to the method described by Elsherbini et al.Citation19 The gold-coated magnetic nanoparticles were prepared as follows. A 0.01 mg aliquot of the prepared magnetic nanoparticles was suspended in 25 mL of glycerol. The suspension was shaken vigorously on the magnetic stirrer and heated to approximately 200°C. The solution of gold salt (5 mL of 5 × 103 mol/L) was then added dropwise. The color of the mixture changed from dark-brown to deep-red. Heating was continued with stirring for other 15 minutes. The AuFe3O4 nanoparticles were purified several times by adding 100 mL of absolute ethanol, and then dried at 60°C and stored at 4°C.
Preparation of tumor-bearing mice
Female 6–8-week-old Swiss albino mice (20.0–29.2 g, median 26.3 g) from the breeding unit of the Egyptian National Cancer Institute were subcutaneously inoculated with 1 × 106 Ehrlich carcinoma cells/mL to obtain a single grafted tumor. Ehrlich carcinoma cells were implanted in all mice on the same day and under the same conditions. During implantation, the mice were anesthetized using fentanyl dihydrochloride (Janssen Cilag, Neuss, Germany) 50 mg/kg body weight. The tumors started to appear within 7 days of implantation. Twenty days following implantation, tumor volumes for all mice were measured using calipers in three orthogonal diameters (D1, D2, and D3, see ).
Characterization instruments
Transmission electron microscopy of the prepared samples was performed by drying a drop of the solution on a carbon-coated copper grid. Particle sizes were determined from micrographs taken using a Joel-100S transmission electron microscope with a resolution of 0.3 nm. An X-ray diffraction analysis crystallographic study was performed on the nanocomposite powder using a rotating anode X-ray diffractometer (Rigaku, Tokyo, Japan) with Cu Ka radiation. Spectroscopic characterization of the colloidal solution of gold nanospheres and core shell nanoparticles was undertaken using an ultraviolet-visible spectrophotometer (Lambda 40, Perkin-Elmer, Redmond, WA) with a matched quartz cell and a path length of 1 cm.
In vivo laser experiments
The experiments started 4 weeks following tumor implantation when a grossly visible and palpable mass of tumor tissue was present. Tumor volume measurements showed that the initial mean tumor volume was 74.8 ± 1.3 mm3. The mice were allocated to four groups containing equal numbers of mice (n = 25), ie, group I (control), group II (phosphate-buffered saline), group III (gold nanospheres), and group IV (gold-coated magnetic [Au@Fe3O4] nanoparticles). The experiments were done in the following sequence.
The skin at the tumor site was swabbed with polyethylene glycol (PEG) as an index matching agent to maximize penetration of light into the tissue, and PEG-passivated green, a near infrared laser-absorbing agent. The group I (control) mice did not receive injections or laser exposure. All mice in group II were injected with 15 μL of 10 mM phosphate-buffered saline, and mice in group III and group IV were injected with 100 μL of gold nanospheres and Au@Fe3O4 nanoparticles, respectively. The injected volume of all solutions was fixed at 1.0 mL. All nanoparticles were administered via direct injection into the tumor interstitium at a rate of approximately 1 mL at 3-minute intervals. The mice were anesthetized with isoflurane via a face mask after a time interval of approximately 30–60 minutes from starting the injection. This time was sufficient to allow the nanoparticles to accumulate in the tumor tissue, and then the laser exposures were started. All tumors in group II and group III were extracorporeally exposed to superficial green laser light with the same parameters (CW λ 535 nm, power 0.5–1.0 W, laser fluency 200 J/cm2, and duration 15–30 minutes) as shown in , while tumors in group IV were injected with core shell nanoparticles and exposed to green diode laser superficially with the same parameters and interstitially exposed to near infrared light (CW, λ 980 nm, power 1 W/cm2) simultaneously, as shown in .
Figure 1 Mouse with Ehrlich tumor under experiment. Two different laser sources: the blue arrow refers to the superficial green diode laser in the visible region, while the red arrow refers to the interstitial red diode laser in the NIR region.
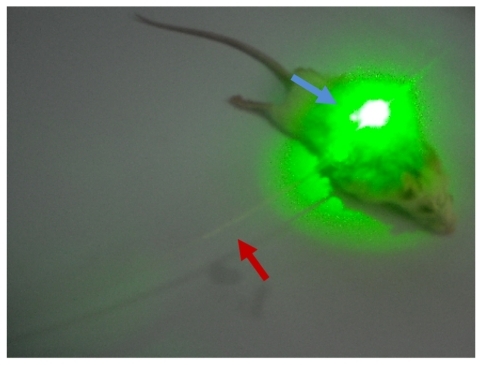
Temperature changes inside the tumor were recorded using a hand-held digital thermometer (Model 100, Barnant Company, Barrington, IL). For recording of interstitial temperature, a copper-constantan thermocouple was inserted and fixed into the tumor 2–3 mm below the surface. The tip of the thermostat was inserted into the injection site through a small incision, followed by exposure to laser light. Temperatures at the injection site were then recorded at 3-minute intervals prior to, during, and following exposure to laser light. The experiments were repeated at 5-day intervals for 30 days following the start of treatment ().
In vivo radiofrequency experiments
One hour after laser exposure, the group IV mice were positioned inside the magnetic field and subjected to radiofrequency for magnetic resonance imaging and treatment at the same time. The radiofrequency experiment was done as follows. The mice were anesthetized with isoflurane 2%–3% via face mask induction through a mobile inhalation system (DRE Inc, Louisville, KY). To focus the radiofrequency on the region of interest, a surface transmit and receive radiofrequency induction coil (inner diameter 7 cm, length 7 cm) specially designed for small animal imaging, was placed in the center of the tumor area in all experiments (). All imaging studies were performed in the Department of Radiology at the Children’s Cancer Hospital, using a 1.5 Tesla high-field magnetic resonance scanner (Magnetom, Espree, Siemens, German) equipped with high-performance gradients (23 mT/m maximum amplitude 120 T/m/sec) and fast receiver hardware (bandwidth ± 500 mHz). The imaging parameters were optimized for all experiments as follows: slice thickness 2 mm, interspaces 1 mm, field of view 160 mm, average 15, matrix 256 × 256, 12 slices per acquisition, power 25 kW, duty 100%, and bandwidth 9.62 kHz. The only variable factor was the duration of exposure, which was limited to 30–40 minutes. All mice in group IV were subjected to radiofrequency before and 15 minutes after localized injection of nanocomposites, which were injected directly into the central portion of the tumors over a 5-minute period.
Assessment of treatment response
The response of the tumor and overlying skin was monitored daily during treatment for 30 days. The changes that occurred in the tumors are described according to their shape and treatment period (3–6 days, 10–15 days, 15–20 days, and 21–30 days). Following the treatment sessions, samples of control tumors and those treated with nanocomposites were fixed in a 1% glutaraldehyde + 4% formaldehyde mixture in 0.1 M phosphate buffer, pH 7.2, followed 1 hour later by post-fixing in 1% osmium tetroxide. After washing with distilled water for 30 minutes, the tissue was stained en bloc with 2% uranyl acetate and lead citrate for 30 minutes at 60°C. The cells were then rinsed again in three changes of distilled water, dehydrated in progressively higher concentrations of ethanol and 100% propylene oxide, and embedded in resin. Thin (90 nm) sections were cut on a Reichert Ultracut E ultramicrotome, placed on 200 mesh copper grids, stained with lead citrate, and examined using a Philips CM100 transmission electron microscope. Pictures were captured using a charge-coupled device camera from Image Processing Systems GmbH (Gaunting, Germany) operating at 120 kV. The necrotic area in each specimen was analyzed morphometrically using image processing software (NIH Scion Image, Frederick, MD). The percentage of necrosis in proportion to the tumor area was calculated as:
where (n) represents necrotic area and (t) represents tumor cell area. All pathological studies were done at the Children’s Cancer Hospital, and all slides were assessed by a certified pathologist.
This study also assessed response to treatment by monitoring tumor growth rates, measuring changes in tumor volume before and after hyperthermia treatment, and the relative tumor volumes with respect to the control tumors. Ellipsoidal tumor volumes were measured following treatment. The tumor volume changes were scheduled and presented as the mean ± standard deviation, and are shown both in table form and as graphical representations in the form of growth curves for both the experimental and control groups.
All mice were housed in similar environmental conditions and received the same diet. The number of mice surviving 80 days in groups II, III, and IV were considered to be an indicator of treatment efficacy. The number of dead mice were recorded at 10-day intervals, with the pattern of mortality shown as the decrease in numbers of surviving mice during the follow-up period.
Statistical analysis
Statistical analysis was performed by comparing mean tumor sizes at day 0 and day 30 post-treatment and computing the level of significant difference as measured by the P value. Similarly, P values were computed to compare mean interstitial temperatures for groups treated with phosphate-buffered saline, gold nanospheres, and core shell nanoparticles. P values less than 0.05 were considered to be statistically significant, and less than 0.001 was considered to be very statistically significant. Statistical analysis of the area of necrosis was done using Student’s t-test with SPSS statistical package (v 12; SPSS Inc, Chicago, IL).
Results
Characterization of gold nanospheres and nanocomposites
The ultraviolet-visible absorption spectra and transmission electron micrographs shown in demonstrate a peak, with maximum absorption centered at a λ of approximately 520 nm, corresponding to gold nanospheres. The core shell peak is broader than that of the gold nanospheres and centered at a λ of approximately 537 nm ().
Figure 2 UV-Vis absorption spectra of (A) gold nanosphere and (B) Au@Fe3O4 core–shell nanocomposites. Inset: transmission electron microscope image of gold nanosphere.

Transmission electron microscopy of the gold nanospheres shows that the size was about 15 ± 1.5 nm. On the other hand, transmission electron microscopy of the prepared Au@Fe3O4 core shell nanoparticles shows approximately spherical shapes with different sizes of about 54 ± 3 nm as well as superparamagnetic iron oxide nanoparticles (Fe3O4) showing spherical shapes, with sizes in the approximate range of 22–55 nm ().
Figure 3 TEM images and XRD patterns of (A) SPIO nanoparticles (Fe3O4), and (B) Au@Fe3O4 core–shell nanocomposites.
Note: The red line represents XRD of SPIO (A), while the blue line represents the core shell (B).
Abbreviations: TEM, transmission electron microscopy; XRD, X-ray diffraction; SPIO, super paramagnetic iron oxide.
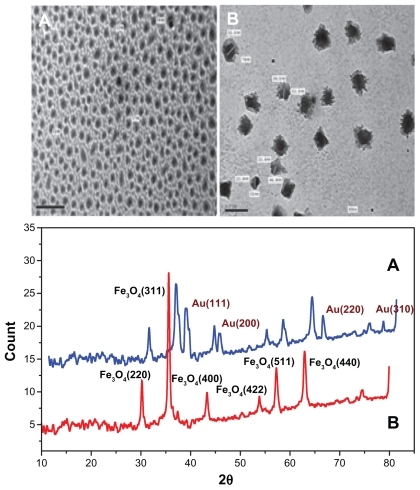
The X-ray diffraction spectra of the superparamagnetic iron oxide (Fe3O4) nanoparticles and Au@Fe3O4 core shell nanoparticles revealed characteristic diffraction peaks of Fe3O4 marked by their indices of 220, 311, 400, 422, 511, and 400 (at 2θ of 30.1°, 35.5°, 43.1°, 53.4°, 57.0°, 62.6°, and 75.1°, respectively), while the characteristic diffraction peaks of gold are marked by their indices of 111, 200, 220, and 310 (at 2θ of 38.20°, 45.10°, 65°, and 78.1°, respectively, ).
Temperature measurements
Interstitial temperatures were measured during each laser treatment method over the treatment sessions for all mice in group II (phosphate-buffered saline), group III (gold nanospheres), and group IV (core shell nanoparticles). The mean initial temperature inside the tumors was 26.1°C ± 1.2°C for all mice. Under laser irradiation, interstitial temperatures increased with time until a maximum temperature was achieved. The minimum and maximum temperatures were 30°C–34°C ± 2.5°C for group II (phosphate-buffered saline), 45°C–48°C ± 1.5°C for group III (gold nanospheres), and 48°C–50°C ± 1.5°C for group IV (core shell nanoparticles). The mean time taken to maximum temperature was (12 ± 3 minutes, 9 ± 2 minutes, and 5 ± 1 minutes, respectively, ).
Figure 4 Mean of thermal transient measurements of Ehrlich tumor interstitial during plasmonic photo thermal treatment, using direct injection of Au nanospheres, 1 mL 0.9% PBS and core shell (Au@Fe3O4).
Note: Data reported represents the mean values along the treatment sessions; errors reported as standard deviation.
Abbreviations: PBS, phosphate buffer saline; Au, gold.
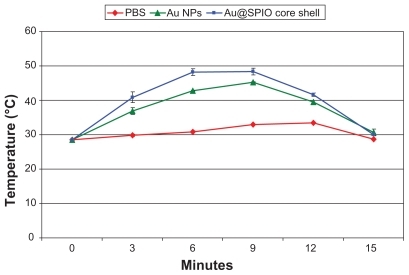
Macroscopic changes
Some morphological changes appeared on the tumor surfaces on different treatment days. Within 3–6 days of starting treatment, a black discoloration indicating necrosis appeared on the tumor surfaces. Within 10–15 days of repeating the treatment, this black discoloration turned into a crust covering an ulcer. The dimensions of these ulcers were small and varied considerably between the groups, being deeper and larger in group III (gold nanospheres) and group IV (core shells) than in group II (phosphate-buffered saline). After 15–20 days of treatment, the crusting covered the entire surface of the tumor. By day 21–30 of the experiment, the tumors had a cauliflower appearance with separation of the crusts, showing that some of the ulcers had started to heal (see ). Separation of the crusts left hairless areas on the backs of the mice that were covered again with a yellowish crust. Only about 10% of mice in group II (phosphate-buffered saline) had these findings compared with 90% of mice in group II (gold nanospheres) and group IV (core shells). In contrast with mice in group III (gold nanospheres), most of the mice treated with the nanocomposites showed a marked reduction in tumor depth (see ).
Microscopic changes
After six treatment sessions, pathological findings in all specimens from mice treated with gold nanospheres and nanocomposites showed widespread tumor cell disaggregation coupled with more nuclear hyperchromatism, cytoplasmic retraction, cell fragmentation, and loss of normal tissue architecture and membrane integrity, as well as infiltration and inflammatory cells, revealing different degrees of necrosis. Transmission electron micrographs were acquired from the control tumors and tumors treated with core shell nanoparticles only. In the control samples, there were no pathological changes (). shows two daughter cells immediately taking up the injected core shell nanoparticles. Nearly all nanoparticles visible are outside the cells and aggregated within the extracellular spaces (marked arrows). shows evidence of uptake of nanoparticles inside the cell by endocytosis after a time delay of about 1 hour, indicating phagosomes containing significant numbers of nanoparticles. shows deformation of the cell membrane after the first treatment session. After three sessions of treatment, some cellular changes were evident on transmission electron microscopy, as shown in , which reveals that the cytoplasm is more condensed, with partial detachment of the cell membrane and disappearance of all morphological features when compared with controls. Transmission electron microscopy at the end of the treatment sessions showed similar signs of cell damage in tumors treated with core shell nanoparticles and gold nanospheres, with the cytoplasm containing large vacuoles, destruction of the cell membrane (yellow arrows), and cytoplasm spilling out into extracellular space. Adjacent to the cell, there are unstructured necrotic structures (blue arrows) which may be the remains of destroyed cells, as well as evidence of the core shell nanoparticles (red arrows, ).
Figure 5 Transmission electron microscope pictures (2.4 μm) of subcutaneous EAC (A) control, (B and C) after injection by core shell NPs and (D–G) after (laser + RF irradiation) one, three, and six sessions of treatments, respectively.
Abbreviations: EAC, Ehrlich ascites carcinoma; NPs, nanoparticles; RF, radio frequency.
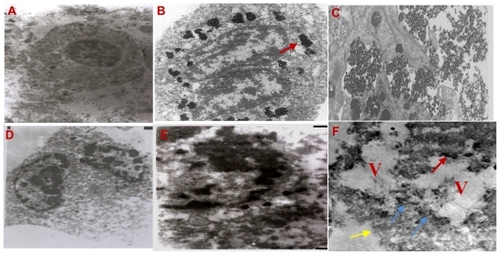
Tumor volume measurements
Changes in tumor volume were measured at 5-day intervals for 30 days and are presented as the mean ± standard deviation for groups I, II, III, and IV from the day of starting treatment (day 0, volume 74.8 ± 1.3 mm3). The mean change in tumor volume for each group is reported in .
Table 1 Mean value of tumor volume growth of the four groups after 30 days
Quantitative analysis of the tumor volumes for the 25 mice in each group is presented in , showing a delay in mean tumor growth for all mice subjected to green diode laser and treated with gold nanospheres compared with tumor growth in the control mice and those treated with phosphate-buffered saline. This delay gradually increased over the treatment sessions to at least 75% at day 30. In contrast, complete tumor regression was observed in many of the mice in group IV, and shows the decrease in tumor volume as compared with group I, II, and III. Tumor volumes in this group steadily decreased during the experiments, with at least an 85% reduction noted at the end of treatment. No tumor regrowth was observed following complete regression over a period of 30 days. Magnetic resonance imaging showed tumor regression in many of the mice treated with core shells, and finally complete disappearance of 50% of the tumors, as shown in , with a concomitant decrease in signal intensity in the regions of interest. The greatest relative decrease in signal intensity was observed after core shell injection, where the change was 26.8% and continued to a signal loss of 64.9%, with a complete loss of signal at the end of the treatment.
Figure 6 Efficacy of photo thermal therapy of the groups (laser@PBS, laser@AuNPs and core shell treated one against control).
Note: Data reported in the Figure represents the mean values along the treatment sessions.
Abbreviations: PBS, phosphate buffer saline; AuNPs, gold nanospheres.
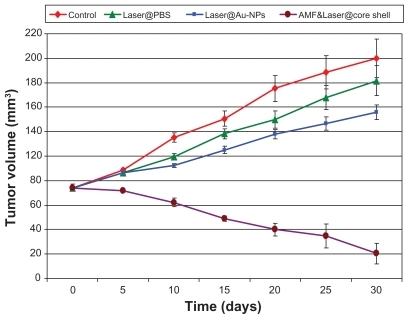
Figure 7 Sagittal T1WI MR images before and after core shell treatment. (A and B) The images revealed loss of signal intensities and complete disappearance of the tumor at the end of the treatment after subjecting to green, NIR, and AMF.
Abbreviations: T1WI, T1 weighted images; MR, magnetic resonance; NIR, near-infrared; AMF, alternative magnetic field.
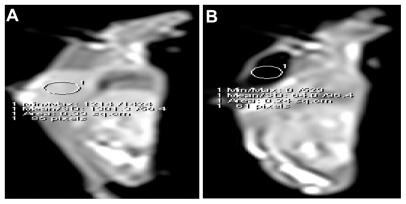
Necrosis
Statistical analysis revealed a mean area of necrosis of 15.3% ± 2.5% in group I (controls), 21.1% ± 2.0% in group II (phosphate-buffered saline), 65.7% ± 1.8% in group III (gold nanospheres), and 85.9% ± 1.3% in group IV (core shell nanoparticles, ).
Figure 8 Results of assessment of necrosis in EAC, indicating a significant difference P < 0.05.
Note: Values indicate the mean percentage of necrosis and standard deviations.
Abbreviations: EAC, Ehrlich ascites carcinoma; PBS, phosphate buffer saline; Au- NP, gold nanospheres; AMF, alternative magnetic field; NP, nanoparticles.
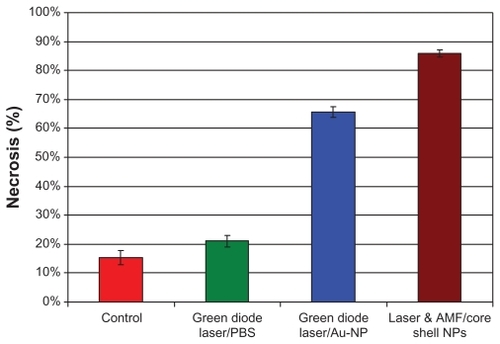
Survival times
Survival at the start of treatment was 100% in all four treatment groups. At 10 days after the start of treatment, group I (controls) and group II (phosphate-buffered saline) mice showed 80% survival, while group III (gold nanospheres) and group IV (core shell nanoparticles) mice showed survival rates of 90% and 95%, respectively. At 20 days, survival in group I was 60%, 70% in group II, 85% in group III, and 90% in group IV. At 30 days, survival was 50% in group I, 60% in group II, 75% in group III, and 80% in group IV. At 40 days, survival was 20% in group I, 35% in group II, 55% in group III, and 60% in group IV. At 50 days, survival was 0% in group I, 10% in group II, 50% in group III, and 55% in group IV. At 60 days, survival was 0% in both group I and group II mice, 40% in group III, and 45% in group IV. At 70 days, survival was 40% in group III and 35% in group IV. After that, only 10% of mice in group IV were still alive until the last day of follow-up ().
Discussion
Ultraviolet-visible absorption spectroscopy is the most widely used method for characterizing the optical properties of nanospheres. In our study, the core shell nanospheres were slightly red-shifted in surface plasmon resonance wavelength, as shown in . This may be attributable to the larger dimensions of the core shell nanoparticles compared with gold nanospheres, as seen in the transmission electron microscopic images. It is worthy of note that the surface plasmon resonance absorption peak of the gold-iron oxide colloid appeared at about 537 nm. This is attributed to the magnetic optical resonance phenomenon, because it is well known that iron oxide particles have no specific absorption peak in this region and that the surface plasmon resonance of gold is approximately 520–530 nm. This phenomenon is thought to be the result of overlap between the plasmonic and magnetic resonances of the gold shell and the core iron oxide, respectively. However, the nature of this overlap remains ambiguous and studies which demonstrate this phenomenon are still quite rare.
Our X-ray diffraction results show that the characteristic peaks of the gold-iron oxide nanospheres were similar to those of the pure iron oxide nanospheres (see ), indicating that the shape of the core determines the shape of the core shell structure. Also, the X-ray diffraction spectrum revealed diffraction peaks of gold that may be attributed to overlap of gold and iron oxide. This is considered to be evidence that the iron magnetic cores are well protected by the gold shell.
The interstitial temperature measurements obtained during each laser treatment method for the 25 mice each in group II, group III, and group IV are described. These temperature measurements are a surrogate for the temperature reached within the tumor cells. As shown in , rapid heating was observed upon laser exposure, followed by a steady-state equilibrium. It was noted that more than 90% of the observed temperature increase occurred within the first 3 minutes. The mean temperature of the core shells was significantly (P < 0.001) higher than that of the gold nanospheres and the phosphate buffer system, and hence the maximum temperature was achieved by the core shell nanoparticles, and with the shortest time taken to reach maximum temperature. This is attributed to irradiation by green visible and near infrared laser light at the same time. After switching off the laser, the tissues showed cooling behavior, as expected. It is worth mentioning that the temperature perturbation induced inside the tumor was mainly due to interaction between the accumulated nanospheres and laser as well as radiofrequency. In fact, determination of the spatial distribution of temperature with different depths and concentrations of nanoparticles in biological tissue is one of the most commonly encountered obstacles in this type of research and remains a complicated issue. This problem is due to the geometry of tumors that are often irregularly shaped in both area and depth.
Use of transmission electron microscopy is very important for characterizing particle uptake and distribution into phagosomes or lysosomes in the cytoplasm. Transmission electron micrographs revealed some aggregation of the injected core shell nanospheres, as shown in , which may have been formed during preparation for electron microscopy. There is evidence that the injected nanospheres penetrated the cell membrane and accumulated in the cytoplasmic vesicles, as shown in . Good transmission electron microscopy pictures () revealed a high response to the nanocomposite treatment technique.
The dramatic changes in tumor growth seen in are due to variation in hyperthermic effects which, in turn, depend on the heating efficiency of laser irradiation in the treated tissues of the groups that received phosphate-buffered saline or gold nanospheres compared with controls. The higher therapeutic efficacy of the core shell nanocomposites in group IV compared with the delay in growth rate in response to treatment with gold nanospheres in group III may be attributed to homogenous heat distribution throughout the surface area of the tumor enclosed in the periphery of the tumor by applying green diode laser, as well as interstitial heating by near infrared and radiofrequency, both of which had a high penetration depth, whereas the penetration of green diode laser in group III was not enough to penetrate the entire tumor depth. Nanocomposites were more efficient than the individual gold nanospheres, in that the former have optical interactions with near infrared laser, represented in the gold shell, as well as magnetic interactions with radiofrequency, represented in the magnetic core, whereas the gold nanospheres only have optical interactions with the green diode laser. Therefore, the effect on cancer cells was more strongly reflected in disappearance of the tumor, and evident in .
The cell death mechanism is temperature-dependent. In our study, the temperature reached was sufficient to induce necrosis, and is confirmed in the transmission electron microscopic images. The quantitative assay of necrosis is shown in , and reveals that the extent of necrosis was significantly higher in group IV than in group III (P < 0.05), and was almost nonexistent in group I and group II. The effect of hyperthermia on the cell death mechanism is still under investigation. Researchers in this field have offered various explanations for these effects. Huff et alCitation20 state that, due to their rapid metabolic rates, tumor cells are regarded as increasingly vulnerable to hyperthermic effects, such as disruption of metabolic signaling processes, protein denaturation, and the onset of acidosis or apoptosis caused by the production of heat shock proteins. On the other hand, Hildebrandt et alCitation21 state that small increases in local temperature result in disruption of nuclear and cytoskeletal assemblies. A recent report by Tong et alCitation22 indicates that there is significant membrane blebbing under hyperthermic conditions. However, in severe conditions, the effects of hyperthermia result in irreversible damage to tumor cells, which indeed was achieved in group IV mice in our study.
The survival rates shown in are in agreement with the microscopic results for tumor responses to the different treatment schedules. Group IV mice showed the best survival rate of 60% in the first 10 days after the end of the treatment compared with 55% in group III. At the end of the follow-up period, survival was about 10% in group IV mice, whereas all the mice in group III died. In general, the shortest survival was in the group I mice and longest in group IV mice.
There are two main advantages of the plasmonic photothermal therapy technique. Firstly, there is the benefit of photostability compared with the photosensitizer dyes used in photodynamic therapy, which suffer from photobleaching as well as diffusion under laser irradiation. Secondly, there is the advantage of absorption and scattering cross-sections of gold nanoparticles, which are significantly superior to the absorbing dyes conventionally used in biological systems. Mie theory estimates that the optical cross-sections of gold nanospheres are typically four to five orders of magnitude higher than those of conventional dyes.
In spite of much progress having been made using the plasmonic photothermal therapy technique for cancer treatment in a laboratory setting, there are still many factors which must be taken into account before this method may be taken to a clinical setting, and they need to be studied further. First of all, the distribution of the elevated temperature under plasmonic photothermal therapy treatment is related to absorption of light by nanospheres acting as point wise local heat sources and by thermal diffusion over surrounding tissues. At the practical level, plasmonic photothermal therapy needs to provide an appropriate temperature increment, ΔT, gold nanosphere concentration, laser power density, duration of laser exposure, optimization of absorption and scattering cross-sections of nanospheres, as well as penetration of the laser light into the area of interest. It should be noted that the biological effects have a nonlinear dependence on changes in particle concentration and laser power density, which is defined by the type of tissue and thermoregulation ability of the living organism.
Conclusion
The current study investigated two approaches to the application of photothermal therapy. Photothermolysis of subcutaneous tumors was studied in vivo by local injection of gold nanospheres and nanocomposites into tumor tissue. We have demonstrated that a pair of synthetic nanospheres can work together more effectively for inducing hyperthermia than individual nanospheres, whereby more than 50% of nanocomposite-treated mice showed complete tumor regression.
Acknowledgments
We gratefully acknowledge the financial support of the National Institute of Laser, Cairo University, and the Children’s Cancer Hospital in this research.
Supplementary figures
Figure S1 (A) Photographs showing measurement of tumor size in mice by a caliper in two dimensions, ie, vertical and horizontal. (B) Core shell-treated mouse with morphological changes appearing on the tumor surface after being subjected to green diode laser and interstitial near infrared at the same time followed by AMF. Red arrow refers to reduction of height dimension.
Abbreviation: AMF, alternative magnetic field.

Figure S2 (A) Photograph of mouse with subcutaneous Ehrlich carcinoma tumor injected with colloidal gold nanosphere solution, and subjected to green diode laser. An interstitial probe was inserted into the tumor for measuring interstitial temperature (red arrow). The digital thermometer reading was 34.4°C within the first 2 minutes of exposure. (B) Photograph of mouse with subcutaneous Ehrlich carcinoma tumor injected with gold nanospheres and subjected to green diode laser. An interstitial probe was inserted into the tumor for measuring temperature. Maximum temperature was achieved after 5 minutes of radiation, as shown in inset.
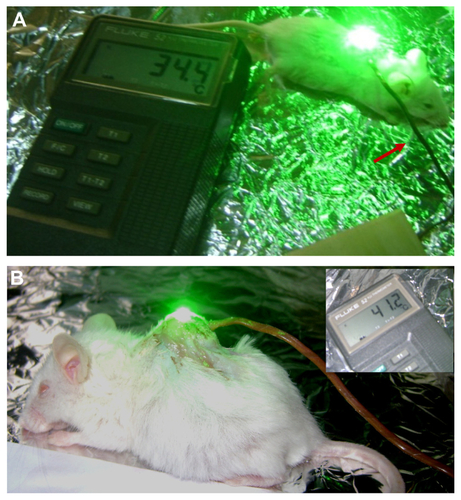
Disclosure
The authors report no conflicts of interest in this work.
References
- MullerGRogganALaser-Induced Interstitial ThermotherapyBellingham, WASPIE Press1995
- WustPHildebrandtBSreenivasaGHyperthermia in combined treatment of cancerLancet Oncol2002348749712147435
- TuchinVVTissue Optics: Light Scattering Methods and Instruments for Medical Diagnosis2nd edBellingham, WASPIE Press2007
- ItoABTanakaKLKondoKNShinkaiMMHondaHKobayashiTTumor regression by combined immunotherapy and hyperthermia using magnetic nanoparticles in an experimental subcutaneous murine melanomaCancer Sci20039430831312824927
- KasiliPMDinhTVPhotothermal treatment of human carcinoma cells using liposome-encapsulated gold nanoshellsNanobiotechnology20051245252
- MaksimovaILAkchurinGGKhlebtsovBNTerentyukGSNear-infrared laser photothermal therapy of cancer by using gold nanoparticles computer simulations and experimentMed Laser Appl200722199206
- DickersonEBDreadenECHuangXGold nanorods assisted near-infrared plasmonic photothermal therapy PPTT of squamous cell carcinoma in miceCancer Lett2008269576618541363
- ChenJWangDXiJImmuno gold nanocages with tailored optical properties for targeted photothermal destruction of cancer cellsNano Lett200771318132217430005
- PissuwanDValenzuelaSMCortieMBTherapeutic possibilities of plasmonically heated gold nanoparticlesTrends Biotechnol200624626716380179
- VisariaRBischofJCLorenMNanotherapeutics for enhancing thermal therapy of cancerInt J Hyperthermia20072350151117952764
- HuangXJainPKEl-SayedIHEl-SayedMAPlasmonic photothermal therapy PPTT using gold nanoparticlesLasers Med Sci20082321722817674122
- HirschLRGobinAMLoweryARMetal nanoshellsAnn Biomed Eng200634152216528617
- LiuVGCowanTMJeongSWJacquesSLLemleyECChenWRSelective photothermal interaction using an 805-nm diode laser and indocyanine green in gel phantom and chicken breast tissueLasers Med Sci20021727227912417982
- GobinAMLeeMHHalasNJJamesWDDrezekRAWestJLNear-infrared resonant nanoshells for combined optical imaging and photothermal cancer therapyNano Lett200771929193417550297
- LooCLoweryAHalasNWestJDrezekRImmunotargeted nanoshells for integrated cancer imaging and therapyNano Lett2005570971115826113
- YangWLNairDGMakizumiRHeat shock protein 70 is induced in mouse human colon tumor xenografts after sublethal radiofrequency ablationAnn Surg Oncol20041139940615070600
- HirschLRStaffordRJBanksonJANanoshell-mediated near-infrared thermal therapy of tumors under magnetic resonance guidanceProc Natl Acad Sci U S A2003100135491355414597719
- DiagaradjanePNShettyASWangJKElliotALSchwartzJSKrishnanSModulation of in vivo tumor radiation response via gold nanoshell-mediated vascular-focused hyperthermia: Characterizing an integrated antihypoxic and localized vascular disrupting targeting strategyNano Lett200881492150018412402
- El-SherbiniAAMahmoudSAMohamedAAAhmedAWHeshamASMagnetic nanoparticles-induced hyperthermia treatment under magnetic resonance imagingJ Magn Reson Imaging201129272280
- HuffMTTongYNZhaoLMHansenJWChengKXWeiAAHyperthermic effects of gold nanorods on tumor cellsNanomedicine2007212513217716198
- HildebrandtBAWustBPAhlersAOThe cellular and molecular basis of hyperthermiaCrit Rev Oncol Hematol200243335612098606
- TongLNZhaoYYHuffTJHansenMKWeiAChengJGold nanorods mediate tumor cell death by compromising membrane integrityJ Adv Mater20071931363141