Abstract
Pulsed laser interaction with small metallic and dielectric particles has been receiving attention as a method of drug delivery to many cells. However, most of the particles are attended by many risks, which are mainly dependent upon particle size. Unlike other widely used particles, biodegradable particles have advantages of being broken down and eliminated by innate metabolic processes. In this paper, the perforation of cell membrane by a focused spot with transparent biodegradable microspheres excited by a single 800 nm, 80 fs laser pulse is demonstrated. A polylactic acid (PLA) sphere, a biodegradable polymer, was used. Fluorescein isothiocyanate (FITC)-dextran and short interfering RNA were delivered into many human epithelial carcinoma cells (A431 cells) by applying a single 80 fs laser pulse in the presence of antibody-conjugated PLA microspheres. The focused intensity was also simulated by the three-dimensional finite-difference time-domain method. Perforation by biodegradable spheres compared with other particles has the potential to be a much safer phototherapy and drug delivery method for patients. The present method can open a new avenue, which is considered an efficient adherent for the selective perforation of cells which express the specific antigen on the cell membrane.
Introduction
Recent advances in nano/microparticle technology have accelerated the application of these particles in biomedicine. Various promising and potential applications of these particles have been reported, including tissue engineering,Citation1 diagnostics,Citation2 cancer therapy,Citation3 and drug delivery.Citation4,Citation5 However, many papers on the evaluation of risks in the use of the particles for biomedical applications are reported. Studies have reported nanoparticle-induced inflammation, effects on immunological systems, prethrombosis, and genotoxicity, which are dependent on particle size.Citation6 Particles that may induce toxic effects at the nanoscale include gold,Citation7,Citation8 silver,Citation9 titanium dioxide,Citation10 and carbon black particles.Citation11 Unlike the above particles, biodegradable particles have the advantage of degradation and elimination by innate metabolic processes. Biodegradable polymers have been widely used for sutures and bone fracture fixation materials in patients because of their degradation and nontoxic properties, and therefore biodegradable polymers have recently received considerable attention for use in drug delivery.Citation12,Citation13
There is a growing interest in laser interaction with particles for biomedical applications. Laser-mediated transfection is a promising nonviral method for spatially targeted therapy because of the high spatial controllability of laser pulsed energy delivery. Moreover, catheter-based transfection may also come into practical use because laser energy can be transmitted through an optical fiber.Citation14 Laser irradiation to the antibody-conjugated particles which are selectively bound to targeted cells realizes tumor detection,Citation15 photothermal therapy,Citation3,Citation16 and drug delivery.Citation17,Citation18 The use of a femtosecond laser with gold nanoparticles for the cell membrane perforation has also been reported.Citation19–Citation21 In this method, collective free electron oscillation (surface plasmon) inside the gold particle generates a highly enhanced near field, resulting in the generation of cavitation bubbles and shock waves in the close vicinity of the cell membrane. The use of carbon black nanoparticles as an optical absorber for initiating carbon-steam reactions has also perforated cell membranes.Citation22 These methods realize the membrane perforation of many cells by a single laser pulse treatment. However, the above concerns related to nanoparticle-induced cytotoxicity still remain unsolved.
Recently, cell membrane perforation by a focused optical far field generated by many dielectric microspheres excited by a single femtosecond laser pulse has been reported.Citation23 Fluorescent molecules and small interfering RNA (siRNA) were delivered into many cells in a large irradiated area by a single 80 fs laser pulse in the presence of antibody-conjugated polystyrene (PS) spheres. The transparent sphere works as a microlens,Citation24 and the cell membrane under the sphere can be perforated by a focused optical field. This method has an advantage in the high throughput in which many cells could be treated by laser scanning method.
In this study, the in vitro cell membrane perforation is demonstrated by using a biodegradable microsphere, instead of PS sphere, excited by an 800 nm femtosecond laser pulse to direct the method towards a much safer phototherapy and drug delivery outcome for patients. This process is based upon a single-shot femtosecond laser illumination to optically transparent polylactic acid (PLA) microspheres being conjugated to the cell membrane for perforation. PLA is a typical biodegradable polymer which can be broken down in biological tissue within a few months.Citation25
Materials and methods
Simulation system and procedure
The enhanced optical field under the PLA sphere in water was calculated by the three-dimensional (3D) finite-difference time-domain (FDTD) method. The simulation system consists of a PLA sphere (n = 1.45) in water (n = 1.326). A plane laser wave is incident to the sphere with the wave vector in the z direction. The incident wave of 800 nm in wavelength is linearly polarized along the x-axis. The enhanced optical field was simulated around the PLA sphere of 250, 500, 1000, and 2000 nm in diameter.
Cell culture
Human epithelial carcinoma cells (A431 cells) were obtained from RIKEN BRC (Tsukuba, Japan). The cells were cultured as a monolayer in Dulbecco’s Modified Eagle’s Medium supplemented with 10% fetal bovine serum under a humidified atmosphere of 95% air and 5% CO2 at 37°C. The cells were harvested and seeded in glass-bottom culture dishes for the experiments.
Cell membrane perforation
shows a conceptual diagram of dielectric sphere-mediated perforation using femtosecond laser. Spherical protein A conjugated PLA spheres, which have a diameter of 2000 nm, were mixed with anti-epidermal growth factor receptor (EGFR) mouse monoclonal antibody (Thermo Fisher Scientific, Fremont, CA). The anti-EGFR antibody can be employed to target overexpressed EGFR on A431 cells. The mixture was stirred for 25 minutes at room temperature. After the removal of unbound antibody by centrifugation for 10 minutes at 10,000 rpm, the conjugated PLA microspheres were resuspended in phosphate-buffered saline (PBS) and added to A431 cells. The cells were incubated for 40 minutes at 37°C and washed three times with PBS to remove the unbound microspheres. The uptake of the microspheres by the cells was not observed in the incubation time of 40 minutes in this study.
Figure 1 Conceptual diagram of dielectric sphere-mediated perforation using femtosecond (fs) laser.
Notes: Biodegradable spheres are conjugated to cell membrane via antigen– antibody interaction. Femtosecond laser illumination to the spheres generates a strongly enhanced optical field under the sphere for perforation.

A Ti:sapphire chirped pulse amplification laser system (Libra, Coherent, Santa Clara, CA), which generates 80 femtosecond (fs) laser pulses at 800 nm central wavelength, was used in the experiments. Photons at wavelength of 800 nm can penetrate deeply into tissue due to the low optical absorption and relatively low scattering coefficients. The laser beam was weakly focused by using a plano-convex lens (f = 200 mm) to a laser spot size of 300 μm. The cells were illuminated by a single shot of the linearly polarized laser from the top side of the cells. The fluorescein isothiocyanate (FITC)-dextran (20 kDa, Sigma, St Louis, MO) and Alexa Fluor-labeled siRNA (15.5 kDa, Qiagen, Chatsworth, CA) were used for the evaluation of the permeabilization. The FITC-dextran solution (0.1 mM) or the siRNA solution (5 μM) was added to the cells just before the 80 fs laser illumination. Two minutes after the illumination, the solution was removed and the cells were washed three times with PBS. Fluorescent molecules uptaken by the cells were observed by using a fluorescence microscope (Eclipse Ti-E, Nikon, Tokyo, Japan). The cell viability was evaluated by a trypan blue dye exclusion test.
Results and discussion
shows the optical intensity distribution at 800 nm wavelength under the PLA microsphere calculated by the 3D FDTD method. The optical near field in the vicinity of the PLA sphere with diameters of 250 and 500 nm is governed mainly by the Mie scattering process. The optical enhancement by the Mie scattering is basically dependent upon the size parameter α = 2πR/λ, where R is the sphere radius and λ is the incident wavelength. The low enhancement factors obtained with sphere diameters of 250 and 500 nm are attributed to the off-resonant Mie scattering regime and the low refractive index difference between PLA sphere (n = 1.45) and water (n = 1.326) at 800 nm wavelength.Citation24,Citation26 The underlying physics for enhanced optical field under the sphere shifts from Mie resonance scattering domain to microlens effect with the increase of the sphere diameter. The enhancement factor obtained with the PLA sphere diameter of 1000 nm is 4.0 in relation to the incident optical intensity, while that obtained with the PS sphere of 1000 nm diameter is enhanced to be 8.4.Citation23 The higher enhancement factor with the PS sphere is explained by the higher refractive index of the PS sphere (n = 1.577) compared with the PLA sphere.Citation26 The PLA sphere of 2000 nm diameter mainly behaves as a microlens and the optical intensity is enhanced by a factor of 9.7 in relation to the incident optical intensity at 870 nm under the sphere (). Based on the optical intensity distribution, the spheres conjugate to the top surface of the cell work for the perforation, while those conjugate to the side surface may not. The full width at half maximums (FWHMs) on the x- and y-axes were 609 and 551 nm, respectively, suggesting that the submicrometer pores may be formed on the cell membrane.
Figure 2 (A–D) Optical intensity distributions on the yz plane simulated by the three-dimensional finite-difference time-domain method for PLA spheres of different diameters: (A) 250 nm, (B) 500 nm, (C) 1000 nm, and (D) 2000 nm. A plane wave is illuminated to the sphere with the wave vector in the z direction. The incident wave of 800 nm in wavelength is linearly polarized along the x-axis. (E–G) Optical intensity distributions along (E) the z-axis under the sphere, (F) the x-axis under the sphere on the peak intensity, shown as red horizontal line in A–D, and (G) the y-axis under the sphere on the peak intensity. (H) Relative positions of focused far field, PLA sphere, and cell membrane on yz plane in the case of 2000 nm PLA sphere.
Note: Dashed circle and gray plane indicate the positions of the PLA sphere and the cell membrane, respectively.
Abbreviations: FWHM, Width at half maximum; PLA, polylactic acid.
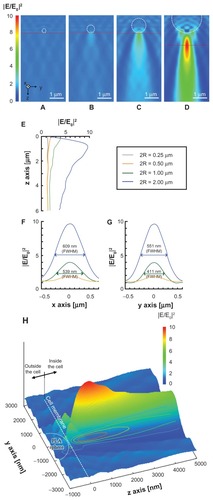
According to the calculated optical intensity, the PLA sphere of 2000 nm in diameter was used for the in vitro experiments.
shows a phase contrast image of the A431 cells and the PLA microspheres taken before the laser illumination. As can be seen in the figure, the spheres of 2000 nm diameter conjugated to the A431 cells, which are 40–50 μm in size. The average number of spheres conjugated to the cell membrane is 51.
Figure 3 Phase contrast image of the A431 cells before laser illumination.
Note: The polylactic acid spheres with a diameter of 2000 nm conjugated to the surface of the cells.
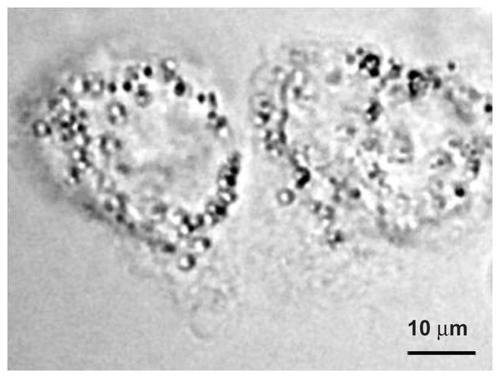
shows fluorescence images (A and C) and phase contrast images (B and D) of the cells after the 80 fs laser illumination at the laser fluence of 1.06 J/cm2, corresponding to the peak intensity of 1.29 × 1014 W/cm2 under the sphere. The corresponding incident energy to the fs laser illuminated area (300 μm in diameter) is 0.75 mJ. show experimental results obtained with the FITC-dextran, while show those obtained with the siRNA. The average number of cells in the fs laser illuminated area was 221. Many cells in the illuminated area showed fluorescence, demonstrating the increase in the cell membrane permeability. The average perforation efficiency, which was defined as the fraction of cells in the irradiated area that took up exogenous molecules, evaluated by using FITC-dextran at this laser fluence, was 26.4% ± 7.5% (N = 5), while that evaluated by using siRNA was 34.9% ± 6.5% (N = 7). No statistically significant difference was observed in the perforation efficiencies with FITC-dextran and siRNA (P > 0.05 in nonparametric Mann-Whitney test). The perforation efficiency obtained by using PLA spheres is comparable to that shown in a previous study using PS sphere,Citation23 demonstrating the effectiveness and applicability of biodegradable polymer for the perforation. As shown in , the PLA sphere of 2000 nm diameter works as a microlens and the focused intensity (far field) is kept high for distances longer than a few micrometers under the sphere, reaching a peak value at 870 nm under the sphere. The distance between the antibody-conjugated sphere and the cell membrane is several tens of nanometers. The long-focused zone of the sphere conjugated to the top surface of cell membrane is considered to be in the cytoplasm. Therefore, it is highly probable that the mechanism for perforation is not only due to the ablation of cell membrane.
Figure 4 Fluorescence (A and C) and phase contrast (B and D) images of A431 cells perforated by using antibody-conjugated polylactic acid spheres irradiated by a single fs laser pulse at 1.06 J/cm2 in the presence of fluorescein isothiocyanate-dextran (A and B) and Alexa Fluor-labeled small interfering RNA (C and D).
Note: Dashed circles (300 μm diameter) indicate the laser irradiated area.
Abbreviation: fs, femtosecond.
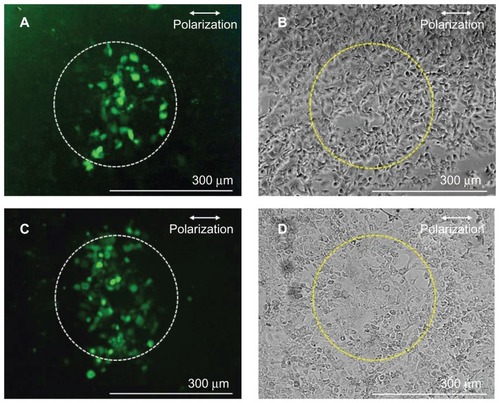
shows the dependence of perforation efficiency and the survival rate on the laser fluence. The estimated peak intensity under the PLA sphere which was derived from is also shown in the figure. At the laser fluences lower than 0.8 J/cm2, the perforation efficiency was lower than 10%. The perforation efficiency was steeply increased at the laser fluences exceeding 0.88 J/cm2, in which the corresponding peak laser intensity under the sphere is higher than 1.08 × 1014 W/cm2. In a previous studyCitation23 using PS sphere of 1000 nm in diameter, the steep increase in perforation efficiency was observed at 1.11 × 1014 W/cm2. These results suggest that the perforation is governed by the optical intensity under the sphere. At laser intensities of between 1013 and 1014 W/cm2, nonlinear optical interactions with liquid and solid media, such as self phase modulation, self-focusing, multiphoton absorption, white-light continuum generation, laser-induced breakdown, laser ablation, are observed.Citation27–Citation29 The utilization of a fs laser at such intensities realizes dissection of axon,Citation30 ablation of corneal stroma,Citation31 and protein crystallization.Citation32 It was reported that the peak pressure produced by fs laser-induced breakdown reaches several hundreds of MPa.Citation33 The peak pressure generated by the femtosecond laser-induced breakdown is much higher than that used for transfection by using a nanosecond laser-induced stress-wave.Citation34,Citation35 Although the membrane permeabilization depends not only on the peak pressure but also on the rise time and the impulse (temporal integration of pressure),Citation36,Citation37 it is highly probable that the femtosecond laser-induced cavitation bubble and the shock wave, as well as ablation of cell membrane, contributed to the cell membrane perforation with the peak intensity at 1014 W/cm2, which is higher than the experimental breakdown threshold in water (5.6 × 1013 W/cm2).Citation38 As can be seen in , at laser fluences lower than 0.8 J/cm2 a slope of 0.61 is obtained, while at laser fluences higher than 0.88 J/cm2 the slope is 1.69. At laser fluences lower than 0.8 J/cm2 with the slope of 0.61, liquid water absorbs laser photons mainly as a linear absorption process. The absorption coefficient of liquid water at 800 nm is as small as 0.01 cm−1. In this low fluence domain, the laser heating process may govern the perforation process. While at the higher laser fluence domain, the laser absorption process is mainly due to the two-photon nonlinear optical process. Actually, the cornea ablation by fs laser is reported at approximately 1 J/cm2.Citation39 In the present study, the high perforation rate is achieved by the two-photon absorption process. The survival rate was defined by the fraction of the cells in the illuminated area that stained with trypan blue. The survival rate slightly decreased with the increase of laser fluence, but it showed still higher than 95% at the highest laser fluence of 1.24 J/cm2 (1.50 × 1014 W/cm2 under the sphere). A slight decrease in the survival rate may be due to the excessive number of pores on the cell membrane for the cells which have too many spheres on the membrane.
Figure 5 Dependence of perforation efficiency evaluated by using fluorescein isothiocyanate-dextran (closed squares) and survival rate (open squares) on the laser fluence.
Notes: A single shot of 80-fs laser pulse was irradiated. The corresponding peak focused intensity under the polylactic acid sphere which was derived from is also shown on the top horizontal axis.
Abbreviation: fs, femtosecond.
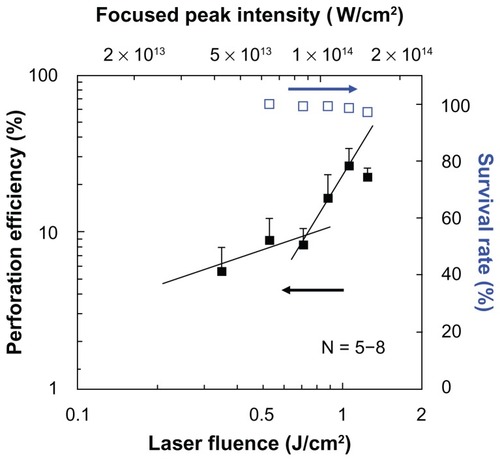
shows a comparison of the average perforation efficiency for the four sets of the experimental conditions. The perforation efficiency by the 80 fs laser illumination with the PLA spheres conjugated to the cell membrane was significantly higher than the three other conditions. Under the condition of the cells without antibody (ie, 80 fs laser illumination without the conjugation of the PLA microspheres to the cell membrane), the perforation was not observed. The unbound microspheres were washed out before the laser illumination because of the lack of the antigen–antibody conjugation. For the cells illuminated with 80 fs laser without the PLA spheres, no perforation was observed. For the cells without the illumination of the 80 fs laser, no perforation was observed. Therefore, combination of the 80 fs laser illumination and the conjugation of the PLA spheres to the cells by antigen–antibody reactions were found necessary for the cell perforation. Based on the calculated optical intensity, the high focused intensity is kept nearly constant for distances longer than 1 μm. Therefore, PLA spheres that are deposited on the cell surface or loosely associated with unwashed cells also work for the perforation when the pulsed laser is illuminated. Considering in-vivo therapy, PLA spheres are accumulated in the close vicinity of targeted cells by antigen–antibody reactions. Antibody works for the cell targeting, and then the laser illumination initiates the perforation. These results indicate that the present method can open a new avenue, which is considered an efficient adherent for the selective perforation of the cells which express the specific antigen on the cell membrane.
Conclusion
In this study, in vitro cell membrane perforation was demonstrated by using an antibody-conjugated biodegradable microsphere illuminated by a single 800 nm, 80 fs laser pulse. FITC-dextran and siRNA were delivered to human epithelial carcinoma cells (A431 cells) by applying an 80 fs laser pulse in the presence of antibody-conjugated PLA microspheres. This method has the advantage of high throughput, in which many cells can be treated by laser scanning method. The number of pulses for treatment area of 1 cm2 was 1415, which can be achieved in 1.4 seconds at the laser repetition rate of 1 kHz. The enhancement factor obtainable under the sphere is dependent on the diameter and the refractive index of the sphere. Biodegradable polymers which have a higher refractive index than PLA, such as polylactic-co-glycolic acid, are potential candidates to be used in future study. The present method, using biodegradable microspheres, can open a new avenue for safer phototherapy and drug delivery in humans.
Acknowledgments
This work was supported in part by a Grand-in-Aid for Young Scientists (A) (23680058) by MEXT, Japan. The authors are grateful to Prof Minoru Obara for constructive comments on this paper.
Disclosure
The authors report no conflicts of interest in this work.
References
- OliveiraMBManoJFPolymer-based microparticles in tissue engineering and regenerative medicineBiotech Prog2011274897912
- LiWBrownPKWangLVXiaYGold nanocages as contrast agents for photoacoustic imagingContrast Media Mol Imaging20116537037722025337
- BardhanRLalSJoshiAHalasNJTheranostic nanoshells: from probe design to imaging and treatment of cancerAcc Chem Res2011441093694621612199
- SinghMChakrapaniAO’HaganDNanoparticles and microparticles as vaccine-delivery systemsExpert Rev Vaccines20076579780817931159
- PauloCSPires das NevesRFerreiraLSNanoparticles for intracellular-targeted drug deliveryNanotechnology20112249494002/11122101232
- AiJBiazarEJafarpourMNanotoxicology and nanoparticle safety in biomedical designsInt J Nanomedicine201161117112721698080
- PanYNeussSLeifertASize-dependent cytotoxicity of gold nanoparticlesSmall20073111941194917963284
- ChenYSHungYCLiauIHuangGSAssessment of the in vivo toxicity of gold nanoparticlesNanoscale Res Lett20094885886420596373
- HussainSMHessKLGearhartJMGeissKTSchlagarJJIn vitro toxicity of nanoparticles in BRL 3A rat liver cellsToxicol In Vitro200519797598316125895
- WarheitDBWebbTRSayesCMColvinVLReedKLPulmonary instillation studies with nanoscale TiO2 rods and dots in rats: toxicity is not dependent upon particle size and surface areaToxicol Sci200691122723616495353
- YamawakiHIwaiNMechanisms underlying nano-sized air-pollution-mediated progression of atherosclerosis – carbon black causes cytotoxic injury/inflammation and inhibits cell growth in vascular endothelial cellsCirc J20067012914016377937
- UleryBDNairLSLaurencinCTBiomedical application of biodegradable polymersJ Polym Sci B Polm Phys20114912832864
- TranVTBenoîtJPVenier-JulienneMCWhy and how to prepare biodegradable, monodispersed, polymeric microparticles in the field of pharmacy?Int J Pharm20114071–211121256947
- SatoSAndoTObaraMOptical fiber-based photomechanical gene transfer system for in vivo applicationOpt Lett201136234545454722139237
- QianXPengXAnsariDOIn vivo tumor targeting and spectroscopic detection with surface-enhanced Raman nanoparticle tagsNat Biotechnol2008261839018157119
- Van de BroekBDevoogdtND’HollanderASpecific cell targeting with nanobody conjugated branched gold nanoparticles for photothermal therapyACS Nano2011564319432821609027
- PitsillidesCMJoeEKWeiXAndersonRRLinCPSelective cell targeting with light-absorbing microparticles and nanoparticlesBiophys J20038464023403212770906
- YaoCQuXZhangZHüttmanGRahmanzadehRInfluence of laser parameters on nanoparticle-induced membrane permeabilizationJ Biomed Opt2009145054034/1719895136
- BaumgartJHumbertLLalondeBSLebrunJJMeunierMPlasmonic enhanced fs-laser optoporation of human melanoma cellsProc SPIE2011792579250I/16
- SchomakerMBaumgartJMotekaitisDMechanisms of gold nanoparticle mediated ultrashort laser cell membrane perforationProc SPIE2011792579250F/16
- BaumgartJHumbertLBoulaisELachaineRLebrunJJMeunierMOff-resonance plasmonic enhanced femtosecond laser optoporation and transfection of cancer cellsBiomaterials20123372345235022177619
- ChakravartyPQianWEl-SayedMAPrausnitzMRDelivery of molecules into cells using carbon nanoparticles activated by femtosecond laser pulsesNat Nanotechnol20105860761120639882
- TerakawaMTanakaYDielectric microsphere mediated transfection using a femtosecond laserOpt Lett201136152877287921808344
- SakaiTTanakaYNishizawaYTerakawaMObaraMSize parameter effect of dielectric small particle mediated nano-hole patterning on silicon wafer by femtosecond laserAppl Phys A20109913946
- MillerRABradyJMCutrightDEDegradation rates of oral resorbable implants (polylactates and polyglycolates): rate modification with changes in PLA/PGA copolymer ratiosJ Biomed Mater Res197711711719893490
- TanakaYObaraGZenidakaATerakawaMObaraMFemtosecond laser near-field nanoablation patterning using Mie resonance high dielectric constant particle with small size parameterAppl Phys Lett201096261103/13
- BrodeurAChinSLUltrafast white-light continuum generation and self-focusing in transparent condensed mediaJ Opt Soc Am B1999164637650
- NaguraCSudaAKawanoHObaraMMidorikawaKGeneration and characterization of ultrafast white-light continuum in condensed mediaAppl Opt200241183735374212078700
- GlezerENSchafferCBNishimuraNMazurEMinimally disruptive laser-induced breakdown in waterOpt Lett199722231817181918188376
- YanikMFCinarHCinarHNChisholmADJinYBen-YakarANeurosurgery: functional regeneration after laser axotomyNature2004432701982215602545
- OliviéGGiguèreDVidalFWavelength dependence of femtosecond laser ablation threshold of corneal stromaOpt Exp200816641214129
- KashiiMKitanoHHosokawaYFemtosecond laser processing of protein crystals in crystallization dropJpn J Appl Phys20054427L873875
- VogelANoackJHüttmanGPaltaufGMechanisms of femtosecond laser nanosurgery of cells and tissuesAppl Phys B200581810151047
- TerakawaMSatoSAshidaHIn vitro gene transfer to mammalian cells by the use of laser-induced stress waves: effects of stress wave parameters, ambient temperature, and cell typeJ Biomed Opt2006111014026/1716526903
- AizawaKSatoSTerakawaMAccelerated adhesion of grafted skin by laser-induced stress wave-based gene transfer of hepatocyte growth factorJ Biomed Opt2009146064043/1920059281
- MulhollandSELeeSMcAuliffeDJDoukasAGCell loading with laser-generated stress waves: the role of the stress gradientParm Res1999164514518
- KodamaTHamblinMRDoukasAGCytoplasmic molecular delivery with shock waves: importance of impulseBiophys J20007941821183211023888
- SchafferCBNishimuraNGlezerENKimAMMazurEDynamics of femtosecond laser-induced breakdown in water from femtoseconds to microsecondsOpt Exp2002103196203
- JuhaszTLoeselFHKurtzRMHorvathCBilleJFMourouGCorneal refractive surgery with femtosecond lasersIEEE J Sel Top Quantum Electron199954902910