Abstract
Lipid-structured vesicles have been applied for drug delivery system for over 50 years. Based on their origin, lipid-structured vesicles are divided into two main categories, namely synthetic lipid vesicles (SLNVEs) and vesicles of mammalian origin (MDVEs). Although SLNVEs can stably transport anti-cancer drugs, their biocompatibility is poor and degradation of exogenous substances is a potential risk. Unlike SLNVEs, MDVEs have excellent biocompatibility but are limited by a lack of stability and a risk of contamination by dangerous pathogens from donor cells. Since the first discovery of plant-derived vesicles (PDVEs) in carrot cell supernatants in 1967, emerging evidence has shown that PDVEs integrate the advantages of both SLNVEs and MDVEs. Notably, 55 years of dedicated research has indicated that PDVEs are an ideal candidate vesicle for drug preparation, transport, and disease treatment. The current review systematically focuses on the role of PDVEs in cancer therapy and in particular compares the properties of PDVEs with those of conventional lipid vesicles, summarizes the preparation methods and quality control of PDVEs, and discusses the application of PDVEs in delivering anti-cancer drugs and their underlying molecular mechanisms for cancer therapy. Finally, the challenges and future perspectives of PDVEs for the development of novel therapeutic strategies against cancer are discussed.
Introduction
Mammalian-derived vesicles (MDVEs) are small vesicles present in mammalian body fluids that are used to transmit biological signals between cells. However, when these vesicles were first discovered, it was thought that they were cellular debris or cellular litter.Citation1 With the development of new technologies, it was discovered that the vesicles not only existed in mammalian body fluids but were also found in multiple plants, which were termed as plant-derived vesicles (PDVEs).Citation2 A number of studies showed that plants were able to produce vesicles in response to a variety of biotic and abiotic environmental stresses, including pathogen infection and attack.Citation3,Citation4
PDVEs are structurally and functionally similar to MDVEs and carry large amounts of proteins, lipids, nucleic acids, and metabolitesCitation5 that are necessary for intercellular signal communication.Citation6 Notably, the signal transfer is cross-species.Citation7 The nucleic acids and proteins in PDVEs can alter the physiological activities of animal cells.Citation8 Unlike MDVEs, PDVEs are easier and faster to extract, are cost effective, and do not involve ethical issues.Citation9 Compared to synthetic lipid vesicles (SLNVEs), PDVEs are much safer. Emerging evidence suggests that PDVEs have good prospects in the development of nano-delivery systems ().
Figure 1 Priorities of plant-derived vesicles compared to mammalian-derived exosomes and liposome nanoparticles.
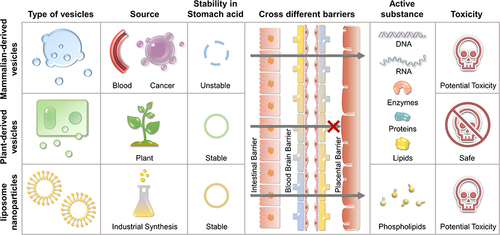
The tumor microenvironment (TME) is completely different from the normal physiological environment and is a prerequisite to support cancer cell proliferation and metastasis.Citation10 However, this unique TME also poses a challenge for cancer treatment. For example, the low pH of the TME can degrade chemotherapeutic drugs before their uptake.Citation11 The inflammatory microenvironment significantly promotes cancer proliferation, metastasis, and drug resistance.Citation12 The relatively hypoxic environment facilitates neoangiogenesis and provides a constant nutrient supply for cancer cell growth.Citation13 In light of these characteristics, nanodrug delivery systems were developed to design intelligent nanocarriers to increase drug targeting and improve therapeutic effects.Citation14 Currently, MDVEs and SLNVEs have been widely used in cancer therapy. However, a number of challenges still remain, such as trans-biological barrier capabilities, acid tolerance, cancer cell-specific targeting, safety, toxicity, potential environmental hazards, and large-scale manufacturing costs. The development of PDVEs has potential to overcome these problems. PDVEs can remain stable in gastric acid mimicsCitation15 and penetrate the intestinal barrier within 6 h.Citation16 Natural PDVEs have active cancer targeting properties and can be improved with acquired modifications.Citation17,Citation18 Furthermore, there are currently no reported instances of PDVEs causing explicit harm to organisms. Thus, it can be tentatively concluded that they are viable carriers for drug delivery, environmentally friendly, and cost-effective.Citation19,Citation20
Emerging studies have validated the unique therapeutic effects of PDVEs in multiple malignancies. For example, Petasites japonicus-derived vesicles were found to act as adjuvants to activate the immune response, and Han et al demonstrated that Petasites japonicus-derived vesicles treated dendritic cells strongly induced the proliferation and differentiation of naive T cells to Th1-type T cells and cytotoxic CD8+ T cells, leading to increased secretion of interferon-γ and interleukin-2.Citation21 Bitter melon-derived vesicles were also reported to inhibit oral squamous cell carcinoma proliferation, as well as overcome drug resistance.Citation22 Interestingly, PDVEs do not seem to exert significant anti-cancer effects on normal cells.Citation23 PDVEs warrant continued investigation in anti-cancer drug research and development in the future. Here, in a systematic review, we present the properties, preparations, and applications of PDVEs for cancer treatment.
Properties of PDVEs Compared to Traditional Vesicles
Comparison with Liposome Nanoparticles
SNLVEs consist of a wide range of particulate systems, including dendrimers, micelles, liposomes, and polymeric nanoparticles. Liposomes are one representative type of SLNVEs, and they have been applied in cancer therapy for a long time due to their good biocompatibilityCitation24 owing to their similar composition to cell membranes.Citation25 Previous research has confirmed the applications of liposomes in various fields, such as encapsulating DNA, RNA, water-soluble drugs, and hydrophobic drugs.Citation26,Citation27 However, liposomes are still limited due to their synthetic nature. It was shown that liposomes induced cellular stress, apoptosis, activation of inflammatory vesicles,Citation28 cytokine storms,Citation29 and immune responses.Citation30 Notably, liposomes were reported to cause severe DNA and RNA damage by upregulating the expression of Heat shock protein 70–2α and Heat shock protein 90.Citation31 In clinical practice, a drug for acute myelogenous leukemia named CPX-351, which is primarily composed of a dual-drug liposomal encapsulation of cytarabine and daunorubicin, caused nonhematologic adverse events to varying degrees.Citation32
Compared to liposomes, PDVEs have demonstrated significant advantages in several key areas. Firstly, the inherent nature of PDVEs ensures that they are safer for the body than SNLVEs,Citation33 and more environmentally friendly.Citation34 Additionally, the RNA and proteins carried within PDVEs facilitate communication with the body. Unlike SNLVES, which merely serve as carriers, PDVEs inherently possess therapeutic effects.Citation35 Furthermore, PDVEs showed an advantage in cellular uptake. A recent study found that the uptake efficiency of PDVEs reached over 80%, while only 40% of liposomes were internalized.Citation36 In summary, in contrast to traditional liposomes, PDVEs have showcased remarkable advantages in various pivotal domains, particularly in the realm of cancer treatment, offering new possibilities for future disease therapies.
Comparison with Mammalian-Derived Vesicles
MDVEs are shed from the cell membrane and carry with them the biological information and metabolic waste of the donor cells.Citation29,Citation30 According to vesicle size, MDVEs are classified into apoptotic vesicles (100–5000 nm in diameter), microvesicles (100–1000 nm in diameter), and the well-known exosomes (30–150 nm in diameter). It was found that MDVEs are widely distributed in mammalian tissues and body fluids, therefore providing a transport mechanism supporting biological signal communication between tissues and organs. MDVEs present remarkable biocompatibility and are widely applied in cancer treatment. For example, plasma-derived exosomes protected miRNAs from nuclease degradation and successfully promoted apoptosis of HepG2 hepatocellular carcinoma cells.Citation37 Additionally, exosome-encapsulated doxorubicin was reported to inhibit cancer grSwth with reduced cardiotoxicity.Citation38 Notably, cancer-derived exosomes are considered as adjuvants in immunotherapy due to the existence of cancer antigen information.Citation39 In addition to exosomes, apoptotic bodies derived from live cells represent a novel drug delivery system. The apoptotic body vehicle can deliver the ammunition to tumor and achieve deep penetration by macro- phage-hitchhiking.Citation40 Adipocyte-derived lipid droplets are also a type of mammalian-derived vesicle, and are increasingly paid great attention for anti-cancer therapies.Citation41 Furthermore, beyond the direct use of vesicles derived from cells, emerging studies have leveraged cell membranes to construct delivery systems ex vivo. For instance, since platelets target postsurgical wounds and circulating tumor cells, platelet-derived membrane vesicles are candidates for drug delivery in post-surgery cancer therapy. Nano-vesicles using platelet membranes have been employed to encapsulate oxaliplatin as a synergistic treatment for post-surgery tumor recurrence and metastasis.Citation42 However, MDVEs secreted by pathological cells have the potential to induce the spread of diseases.Citation43 Furthermore, there are moral and ethical arguments concerning the commercial utilization of MDVEs, and the manufacturing cost of MDVEs is high due to the limited donor availability and low yield. Therefore, MDVEs have not yet met the requirements for the development of clinical drugs.
PDVEs share many similarities with MDVEs, such as a size between 30 and 500 nm, a predominantly circular or cup shape, a negatively charged surface, and an ability for uptake and crossing biological barriers facilitated by phospholipid bilayers. However, unlike MDVEs, PDVEs cannot penetrate the placental barrier, suggesting a better safety profile.Citation36,Citation44 In addition, PDVEs have abundant donor resources, allowing for large-scale production at low manufacturing costs. Ginger root has been reported to contain 0.5–2 × 1014 vesicles/kg, which is 10 times higher than that of MDVEs.Citation9 In addition, PDVEs are non-toxic and are a part of most daily diets as additives. More importantly, as a carrier, PDVEs can protect a drug from gastric acid degradation, while MDVEs are not tolerant of low pH, except for milk-derived MDVEs.Citation36
Preparation of PDVEs
The properties of PDVEs make them promising for cancer treatment. However, it is difficult to produce high purity PDVEs on a large scale,Citation45 given that vesicles tend to adhere to each other through macromolecules, such as starch, cellulose, and tannins.Citation46 Therefore, the extracted PDVEs should be highly dispersed and reproducible for subsequent studies and clinical applications. Generally, the extraction methods of PDVEs are divided into two broad categories, namely physically based techniques and chemically based techniques ().
Table 1 The Advantages and Disadvantages of Various Preparation Methods
Physically Based Techniques
The physically based methods include differential centrifugation, density gradient centrifugation, and ultrafiltration membrane separation. Differential centrifugation is considered as the standard for PDVE extraction and is the most widely used. It is based on the principle that large particles will settle at the bottom layer, while small particles will float at a higher layer under centrifugal force, and the target vesicles can be isolated by adjusting the centrifugal force.Citation47,Citation48 The method is easy to implement and cost-effective and is suitable for the mass production of PDVEs. However, vesicles may be disrupted by the ultra-intense centrifugal forces. A high-density hypotonic solution added to the bottom of the centrifuge tube can protect vesicles from being shattered. Additionally, experimental conditions for differential centrifugation are not standardized and are not interchangeable for each PDVE.Citation44,Citation49 Thus, a personalized centrifugation protocol for each type of PDVE is time-consuming.
To save on extraction time, density gradient centrifugation was developed. Specifically, a series of a continuous or discontinuous density medium are placed in a centrifuge tube according to the gradient, and vesicles with different densities are stratified by centrifugal force.Citation50,Citation51 The density gradient centrifugation method is simple, high yielding, and less time-consuming than many other methods. However, the vesicles obtained by this method are poorly homogeneous and easily clumped, which is not satisfactory for subsequent studies. Thus, differential centrifugation and density gradient centrifugation methods are usually applied together to improve the quality of the vesicles. Generally, a certain amount of vesicles is first extracted by differential centrifugation, and purer vesicles are further obtained by density gradient centrifugation according to the size and density of vesicles.Citation52
In recent years, ultrafiltration membrane separation, a method separating vesicles by size, has also been used to separate PDVEs. A solution is filtered sequentially through porous membranes of different sizes under a certain pressure, and the large particles are physically intercepted by the porous membrane, while small particles are unimpeded.Citation53 The purity of the vesicles extracted by this method is satisfactory. However, the concentration polarization phenomenon appearing during filtration changes the separation performance of the membrane. Also, membrane fouling usually occurs. The phenomenon of concentration polarization and membrane fouling can be effectively improved by adjusting the flow rate, pressure, and concentration of filtration, a process that is highly experience-dependent. In addition, clearance of soluble protein impurities was shown to avoid membrane fouling prior to ultrafiltration separation.Citation54
Chemically Based Techniques
Chemical-based techniques include co-precipitation and immunoaffinity separation methods. Co-precipitation separation is performed by adding a precipitant to a mixture to precipitate the target vesicle and then separating the precipitate to obtain the target vesicle. During the separation of vesicles, surface adsorption of the precipitate utilizing positive and negative charge attraction is the most common separation method.Citation55 Most kits available on the market for vesicle isolation are based on the principle of co-precipitation isolation. However, the method is costly and the vesicles that are obtained are generally impure and need further purification. Immunoaffinity separation is a method based on the specific binding of antibodies to antigens on the vesicle membrane. This method is fast, specific, and pure, but only small amounts can be extracted, and it is a high cost method.Citation56
Taken together, physically based techniques are suitable for mass processing and for obtaining pure vesicles, but the separation parameters are complex and time-consuming. Chemically based techniques are simple and reproducible, but the cost is high. Currently, much attention has been paid to combining both physical and chemical isolation techniques to obtain high purity and reproducible PDVEs.Citation57
Applications of PDVEs in Cancer Therapy
A number of anti-cancer drugs have exhibited strong cytotoxic killing effects in vitro, but the in vivo inhibitory effects were unsatisfactory. Furthermore, the significant systemic toxicity due to the poor bioavailability also limited their clinical application. The emergence of nano-drug delivery systems tends to overcome this problem. The nanocarriers can chemically or physically conjugate the drug and deliver it to the designated lesion site to improve therapeutic efficacy.Citation58 The efficiency of drug targeted delivery to the lesion is determined by the properties of the carriers, including physical properties, surface modifications, cellular uptake, active targeting ability, acid tolerance, and the trans-biological barrier characteristics. PDVEs were first utilized for drug delivery in 2014. The GfDVEs encapsulated methotrexate and were used for ameliorating dextran sulfate sodium induced mouse colitis.Citation44 In recent years, PDVEs with anti-inflammatory,Citation59 anti-cancer,Citation23 anti-bacterial,Citation60 and anti-oxidant aging effects have been found.Citation61–63 They are widely used to prevent or treat cancer,Citation64 colitis,Citation65 alcoholic liver disease,Citation66 and even COVID-19.Citation67 PDVEs are promising as drug delivery systems for cancer treatment because of their intrinsic targeting,Citation68 acid tolerance,Citation69 easy penetration of physiological barriers,Citation18 storage stability,Citation70 non-toxicity to the organism,Citation36 and low fabrication cost ().
As a Carrier
Properties
PDVEs are spherical, elliptical, or cup-shaped in shape, depending on the plant species and the extraction method (). PDVEs have a negative surface charge, which can effectively prolong their residence time in the blood.Citation71 The size of PDVEs from different species is inconsistent and mainly distributed between 30 and 500 nm. For example, the size of Beta vulgaris-derived vesicles is 50 nm,Citation72 that of asparagus-derived vesicles is 119 nm,Citation73 that of ginger-derived vesicles (GiDVEs) is 220–290 nm,Citation66 and that of ginseng-derived vesicles is 344.8 nm.Citation74 In addition, the isolation method also influences the size. For example, the size of GiDVEs extracted by differential centrifugation was 403 nm, while that of vesicles extracted by co-precipitation separation with a 15% concentration of polyethylene glycol-6000 (PEG6000) was 252 nm, which decreased with increase in PEG concentration.Citation55 The vesicle size determines the vesicle distribution in vivo; thus, different extraction methods should be carefully selected according to specific needs. Drug loading into PDVEs can be accomplished by simply co-incubating PDVEs with the drug, which is known as the passive loading technique. Active loading techniques such as ultrasonic treatment and freeze-thaw cycles can also be used to temporarily disrupt the PDVE membrane and increase the drug loading rate.Citation75
Table 2 Physicochemical Characterization of Plant-Derived EVs
pH Tolerance
In clinical practice, oral administration has the highest patient compliance compared to other administration routes. However, a drug can be degraded by gastric acid, which results in a decline of bioavailability. Therefore, it is crucial to improve the acid tolerance of anti-cancer drugs. Several studies performed in vitro acid tolerance tests on Kaempferia parviflora-derived vesicles, and the results showed that Kaempferia parviflora-derived vesicles were stable in simulated gastric acid, maintaining their structural integrity with the size slightly enlarged. The only significant change was that the surface charge changed from negative to positive, due to the neutralization effect by hydrogen ions in the gastric acid.Citation69 Notably, not all PDVEs change surface charges in gastric acid. The size and the electric potential of tea flower-derived vesicles remained unchanged in a gastric simulant, small intestinal simulant, and colonic stimulant.Citation98 An illustrative example of PDVEs applied for oral drug administration is sorafenib. The bioavailability of sorafenib is significantly limited when orally administered due to degradation in gastric acid. However, when encapsulated within Kiwifruit-derived PDVEs, sorafenib exhibited enhanced stability in simulated gastric conditions, subsequently showing improved absorption and therapeutic efficacy.Citation99 The TME is also acidic, and PDVEs with acid tolerance can protect anti-cancer drugs in the microenvironment until they are taken up by cancer cells. In addition, Zhang et al reported that PDVEs were not only acid-tolerant but also resistant to pepsin catabolism.Citation7 In conclusion, PDVEs resist gastric acid degradation in the stomach and avoid degradation by pepsin, which makes them excellent carriers for oral drug administration.
Crossing Barriers
Biological barriers block foreign substances to maintain homeostasis but also prevent drugs from reaching a lesion. When administered orally, most drugs are unable to enter the bloodstream because of the intestinal barrier. When treating brain-related diseases, drugs have difficulties entering the brain due to the blood-brain barrier and instead reach other organs, leading to potentially severe side effects.Citation100 Based on the phospholipid surface and nanostructure, PDVEs can transport a drug through a biological barrier and improve the bioavailability of the drug.
One prominent example showcasing the potential of PDVEs in surmounting biological barriers is their application in delivering dexamethasone (Dex) for neuroinflammation-related disease treatment. Dex is known for its anti-inflammatory properties, but its therapeutic potential is limited due to its poor bioavailability and inability to cross the blood-brain barrier efficiently. Researchers utilized Allium tuberosum-derived PDVEs to encapsulate Dex. Upon administration, these PDVEs were shown high efficiency in crossing the blood-brain barrier, and delivered Dex directly to the affected brain regions. This led to a significant alleviation of inflammation in microglial, demonstrating the potency of PDVEs as drug delivery vehicles for brain diseases.Citation101
To verify the ability of PDVEs to penetrate the intestinal barrier, grape-derived vesicles (GDVEs) were stained with fluorescent dyes. The results showed that GDVEs accumulated in the intestine during the first 6 h after oral administration and were decreased within 48 h.Citation35 Most brain cancers require surgical intervention since the restrictive blood-brain barrier usually prevents drugs from reaching a therapeutic concentration. However, it was found that GfDVEs carrying miR17 could significantly inhibit brain cancer growth, without any toxic effects.Citation92 Notably, although PDVEs could cross biological barriers, they could not penetrate the placental barrier when injected intravenously into pregnant mice, suggesting the high safety of PDVEs in pregnant women.Citation102 Taken together, PDVEs act as intelligent Trojan horses, encasing a drug and crossing a biological barrier to transport it to the lesion. Meanwhile, they are safe for the fetus in the uterus.
Surface Modifications and Active Targeting
Regardless of the delivery method, the drug will be transported everywhere in the body through the circulatory system after administration, which inevitably decreases the drug concentration at the cancer lesion and increases the risk of toxic side effects in other organs. Therefore, it is important to enhance the cancer-targeting ability of PDVEs to improve the bioavailability of drugs at the target and reduce their toxic side effects. This is crucial for increasing the efficacy of anti-cancer drugs.
Currently, there are two main approaches for improving PDVEs through surface modifications. One is loading the vesicle surface with ligands capable of binding with cancer-specific receptors. For example, a therapeutically siRNA is fused into the 3WJ core, modified with folic acid, coupled to cholesterol, and incubated with GiDVEs membranes. By cholesterol anchoring to the membrane, the complex is formed with 80% drug loading.Citation85 It was shown that the vesicles efficiently delivered survivin siRNA to a human oral epidermal-like (KB) cancer model and that folic acid ligands significantly promoted the uptake of GiDVEs by the KB cells. Another approach is to modify vesicles based on the TME. For example, the leukocyte membrane in a cancer-inflammatory environment was utilized to wrap around a GfDVE,Citation93 which avoided the clearance by the immune system and allowed the vesicles to directly deliver to the site of inflammatory cancer. Therefore, the surface of PDVEs is amenable for modification, such as loading small molecules or covering with bionic membranes. This property makes it possible to use PDVEs for enhanced targeting of cancer cells and warrants more in-depth investigation.
Cellular Uptake
The ability to be taken up by mammalian cells is a prerequisite for PDVEs to exchange information between plants and animals. As far as is known, PDVEs are taken up by mammalian cells through multiple pathways, depending on the type of plant donors and the type of animal recipient cells. For example, GfDVEs enter the intestinal cells through micropinocytosis,Citation35 whereas GiDVEs are specifically internalized by enterocytes via caveolin-mediated endocytosis and micropinocytosis.Citation103 The entry of GiDVEs into hepatocytes is ATP-dependent, and its uptake rate is slow at 4°C and increases with increasing temperature.Citation66 Furthermore, it was found that the main pathway of macrophage internalization of GiDVEs was not macrocytic drinking but clathrin-mediated endocytosis. Furthermore, the intracellular GiDVEs directly induced macrophage polarization and improved the cancer immune suppression microenvironment, resulting in an enhanced therapeutic efficacy. This phenomenon suggests that PDVEs can transport their contents into the cytoplasm, thus altering the physiological state of the recipient cells.Citation74
However, current research on the uptake mechanism of PDVEs by mammalian cells is still incomplete, and alterations of gene or protein expression of the recipient cells remain unknown. Elucidation of the molecular mechanisms involved in the internalization process will provide more efficient delivery and better therapeutic effects.
As a Therapeutic Agent
In nature, plants and animals are interdependent, and research on the information exchange between the two biological communities is ongoing. Recently, it was found that the nucleic acids, proteins and enzymes within PDVEs positively regulated the physiological homeostasis of animals when absorbed by their cells.Citation103,Citation104 Thus, PDVEs can act not only as carriers but also as therapeutic agents (). Emerging studies show that PDVEs have anti-cancer effects but have little influence on normal tissues,Citation105 which closely correlated with the contents of the PDVEs.Citation106 Here, we systematically describe the contents of PDVEs and their involvement in anti-cancer strategies, such as chemotherapy, starvation therapy, and immunotherapy ().
Table 3 Applications of PDVEs in Cancer Therapy
Contents
Like MDVEs, PDVEs contain various biological information of donors, including lipids, nucleic acids, proteins, and metabolites. To clarify the therapeutic mechanism of PDVEs, a number of studies have identified several types of inclusions in PDVEs.
In contrast to MDVEs, the lipids of PDVEs are diverse. For example, the lipids of GiDVEs consisted primarily of 42% phosphatidic acid (PA), 27% digalactosyl diacylglycerol, and 19% monogalactosyl diacylglycerol.Citation87 In contrast, the lipids of GfDVEs consisted primarily of 24% phosphatidylethanolamine, 23% phosphatidylcholine (PC), and 13% phosphatidylinositol.Citation111 The lipids of GDVEs were composed of 53% PA and 26% phosphatidylethanolamine.Citation35 Moreover, lipids from different PDVEs have been shown to have different functions. PAs from GiDVEs prolonged the resident time of PDVEs in the small intestine and were absorbed by the intestinal microbiota,Citation60 whereas PAs from GDVEs were able to mediate cell proliferation in the colon.Citation35
miRNAs represent another important component in PDVEs. A total of 198 miRNAs and 39 novel miRNAs were identified in ginger, among which 136 miRNAs were also found in GiDVEs. A molecular mechanism study showed that most co-expressed miRNAs between ginger and its vesicles were responsible for regulating stress responses, root development, and other physiological processes in plants.Citation68 In addition, high-throughput sequencing techniques and bioinformatics analyses have shown that plant-derived miRNAs have the potential to regulate human metabolic activities and even treat human diseases.Citation68 For example, miRNAs in GiDVEs regulated intestinal flora homeostasis and alleviated lipopolysaccharide induced colitis.Citation103 Furthermore, miRNAs extracted from coconut water-derived vesicles can target the metabolism-related mRNAs in human genome.Citation82
Finally, proteins are also considered as significant signaling molecules in PDVEs. Proteomic analysis identified 745 proteins in tea flower-derived vesicles. The Gene Ontology database and gene and genome (KEGG) annotation pathway analysis revealed that the proteins in the vesicles were mainly related to cellular composition, metabolism, and growth. Among them, 16 proteins had oxidation-related functions, suggesting that these vesicles may trigger intracellular oxidative stress.Citation98
In summary, the diverse biological components within PDVEs play pivotal roles in both plant and human physiology. The distinct lipid profiles of various PDVEs not only signify their uniqueness but also underline specific therapeutic potentials, especially in gastrointestinal applications. The presence of numerous miRNAs in PDVEs, particularly those with origins in plants like ginger, emphasizes their potential in regulating a myriad of cellular processes, from stress responses in plants to metabolic activities in humans. Moreover, the abundance of proteins, especially those with antioxidant properties, accentuates the potential of PDVEs in mediating cellular events, possibly even leading to responses like oxidative stress.
Cytotoxic Killing Therapy
Apoptosis and cell cycle arrest are the main evaluating indicators for the development of anti-cancer drugs. It was demonstrated that garlic-derived vesicles (GaDVEs) induced apoptosis in cancer cells by activating caspase-mediated intrinsic pathways. The expression levels of pro-apoptotic genes such as TP53, BAX, CASP3, and CASP9 were significantly increased. In contrast, the expression levels of anti-apoptotic genes were significantly decreased in kidney and lung cancer cells following treatment with GaDVEs.Citation23 In addition, GaDVEs blocked the growth cycle of cancer cells, leading to S phase arrest. GADD45A, a gene regulating cell cycle arrest and DNA damage,Citation112 is an essential signal in the cellular network response to external damage. Yang et al found that lemon-derived vesicles induced upregulation of GADD45A in 3D cultures of SGC-7901 spheroids, resulting in cell cycle arrest.Citation57
However, it is essential to note that the cytotoxic killing effect of PDVEs can be varied depending on the donor species. In one study,Citation110 Cho et al analyzed vesicles derived from four Citrus species: C. sinensis, C. limon, C. paradisi, and C. aurantium, and showed that all four of these PDVEs inhibited the proliferation of A375 (human melanoma), A549 (human lung cancer), and MCF-7 (human breast cancer) cells. However, only vesicles from C. paradisi were able to block the cell cycle of melanoma cells in the G2/M phase by enhancing the gene expression of CDKN1A (encoding p21) and reducing the levels of cyclin B1 and cyclin B2. In addition to plant species differences, the anti-cancer effects of PDVEs produced from different locations of the same plant may also vary. For example, vesicles from the roots of Dendropanax morbifera inhibited melanoma by downregulating the expression of TYR, TRP-1, and TRP-2 in B16BL6 cells, but vesicles from leaves were more effective.Citation74
The contents in PDVEs also determine the cytotoxic killing effects against cancer cells. It was shown that the PDVEs from Cannabis sp. could be classified into high cannabidiol vesicles and low cannabidiol vesicles based on the concentration of cannabidiol. Compared to the low cannabidiol vesicles, the high cannabidiol vesicles significantly inhibited the cell viability of hepatocellular carcinoma cell lines HepG2 and Huh-7 in a time- and dose-dependent manner but had no inhibitory effect on normal growth of HUVECs. Molecular mechanism investigations further indicated that the high cannabidiol vesicles blocked the cell cycle at the G0/G1 phase and activated the mitochondria-dependent apoptotic signaling pathway in hepatocellular carcinoma cells.Citation107
Several experiments have demonstrated the high selectivity of PDVEs for cancer cells. First, the difference in the composition of cancer cell membranes and normal cell membranes affects the uptake pathway of cells, resulting in different amounts of PDVEs entering the cancer cells and normal cells. One study showed that HepG2 cells took up a two-fold increase of asparagus-derived vesicles compared to LO2 cells. Thus, asparagus-derived vesicles upregulated apoptotic factors and induced apoptosis in hepatocellular carcinoma cells but caused less damage to normal hepatocytes.Citation73 Second, PDVEs were more likely to activate apoptotic pathways to which cancer cells are susceptible, such as TRAIL-mediated apoptosis.Citation113 Specifically, vesicles in citrus (Rutaceae) sap exerted their anti-cancer effects by stimulating the TRAIL-mediated apoptotic pathwayCitation97 but had no toxic effects on normal cells.
In conclusion, the potential of PDVEs in anti-cancer therapies is vast, showcasing not only their ability to selectively target cancer cells but also the importance of their donor species and specific plant parts. Whether through specific molecular pathways like caspase-mediated intrinsic or TRAIL-mediated apoptosis, or through the differential composition influencing uptake by cancer versus normal cells, the versatility of PDVEs is evident. The findings also underscore the significance of studying individual constituents, such as cannabidiol concentration, in influencing the cytotoxic effects of these vesicles.
Immune Modulatory Therapy
With the recognition of the body’s innate ability to combat cancer, Immune modulatory therapy took center stage. This approach seeks to harness and amplify the body’s immune system’s natural capability to detect and destroy cancer cells. By modulating immune checkpoints or introducing tailored immune cells, this therapy offers a more targeted approach with fewer side effects than cytotoxic agents.
The TME is infiltrated by a large number of inflammatory cells, including TAMs, lymphocytes, natural killer cells, dendritic cells, and mast cells. TAMs are the most predominant subset.Citation114 TAMs are plastic and can be M1- or M2- polarized upon stimulation by different cytokines. Generally, the M1 phenotype has anti-cancer effects, while the M2 phenotype has pro-cancer properties.Citation115 PDVEs can modulate the immune microenvironment by altering the polarization of TAMs.
Cao et al demonstrated that GiDVEs promoted the polarization from the M2 phenotype to the M1 phenotype.Citation74 The results showed that GiDVE treatment significantly increased the secretion of M1 phenotype-associated cytokines, including tumor-necrosis factor-α (TNF-α), interleukin-12 and interleukin-6, while the expression of the M2 phenotype marker CD206 was significantly reduced.Citation74 To clarify the active components regulating macrophage polarization, TAMs were treated with DNase I, RNase I, proteinase K, or sonicated GiDVEs. The results showed that proteinase K and sonicated GiDVE-treated TAMs were unable to induce macrophage polarization. This indicates that the proteins in GiDVEs are the main components responsible for macrophage polarization, and their structural integrity is essential for regulating this process.
In the last decade, immune checkpoint inhibitors have received much attention in cancer therapy for their ability to promote cytotoxic T cell activity to kill cancer cells. However, immune checkpoint inhibitors are ineffective in solid cancers because of the insufficient infiltration of T cells; thus, these are referred to as cold tumors. PDVEs have been reported to enhance the therapeutic effects of immune checkpoint antibodies by reprogramming the microenvironment of cold tumors. The results showed that GiDVEs reprogrammed TAMs to increase CCL5 and CXCL9 secretion and recruited CD8+ T cells into the TME, converting the cold TME to a hot one. When combined with programmed death-1 inhibitors, the cancer progression in both colon and breast cancer models was inhibited, with no detectable systemic toxicity.Citation108
In summary, the profound effects of GiDVEs on TAM polarization and the subsequent modulation of the tumor microenvironment underscore their potential as vital tools in oncology. The specific protein components in GiDVEs, pivotal for macrophage polarization, emphasize the importance of understanding the molecular components driving these effects. Further, the ability of PDVEs to turn “cold” tumor environments “hot” by reprogramming TAMs, combined with immune checkpoint inhibitors, establishes a promising avenue in tackling even solid tumors.
Oxidative Therapy
However, certain tumors evade the immune system or develop resistance. This led to the exploration of Oxidative therapy, a more recent innovation. Cancer cells, due to their altered metabolism, are often more susceptible to oxidative stress than normal cells. Oxidative therapy capitalizes on this vulnerability by inducing a surge of reactive oxygen species (ROS) in the tumor environment, leading to cancer cell death while sparing most normal cells.
Reactive oxygen species (ROS), including peroxides, superoxides, hydroxyl radicals, singlet oxygen, and α-oxygen, are natural by-products of oxygen metabolism and play an essential role in cell signaling and maintenance of organism homeostasis. However, excessive levels of ROS can cause severe damage to cells.Citation105 Therefore, ROS bursts in the TME can trigger oxidative stress in cancer cells and achieve anti-cancer effects. It was reported that edible tea flower-derived vesicles inhibited metastatic breast cancer by generating ROS. The increased intracellular ROS levels triggered mitochondrial damage and blocked the cell cycle, thus suppressing breast cancer cell proliferation and migration. In contrast, the tea flower-derived vesicles led to little ROS production and minimal mitochondrial damage in normal cells.Citation98 A similar phenomenon was also observed with lemon-derived vesicles.Citation57
Elevated ROS may also improve the susceptibility of drug-resistant cancer cells. It was found that bitter melon-derived vesicles improved the chemosensitivity of 5-fluorouracil-resistant oral squamous cell carcinoma cells and thus enhanced the therapeutic effect of 5-fluorouracil in vitro and in vivo.Citation22 However, when treated with N-acetylcysteine, the chemosensitizing effects disappeared, suggesting that the synergistic effects were dependent on ROS production. Notably, bitter melon-derived PDVEs scavenged excessive ROS production caused by X-ray irradiation in normal H9C2 cells and thus rebalanced the mitochondrial membrane potential and attenuated DNA damage.Citation116 Therefore, the intracellular ROS level may be the underlying reason for the highly selective action of PDVEs against cancer cells.
In essence, the dual role of ROS, both as a potential enhancer of cancer therapies and as a possible protective agent against cellular damage, is evident. The selective action of PDVEs, underpinned by intracellular ROS levels, provides a fascinating insight into their anti-cancer potential and their ability to safeguard non-malignant cells from unwanted oxidative stress. Such a discerning approach offers a promising direction in optimizing cancer treatments while preserving the integrity of surrounding tissues.
Other Therapeutic Effects
In the TME, the relative hypoxic environment stimulates angiogenesis, which ensures the delivery of nutrients required by cancer cell proliferation. Therefore, angiogenesis inhibition can block the supply of nutrients and thus inhibit cancer growth. It was reported that GaDVEs inhibited cancer growth by reducing VEGF expression in vitro, without affecting normal human dermal fibroblasts.Citation23 However, such evidence is still limited, and further investigation is necessary to provide more evidence.
In addition to anti-cancer effects, PDVEs also exhibit remarkable preventive effects against carcinogenesis. It was found that oral administration of tea leaf-derived vesicles reduced the levels of pro-inflammatory cytokines, including TNF-α, interleukin-6, and interleukin-12, while increasing the expression of the anti-inflammatory cytokine interleukin-10, and thus effectively inhibited inflammatory bowel responses. At the same time, the expression of tight junction-related proteins zona occludens protein 1 and mucin 2 were elevated to restore the damaged colonic barrier. Furthermore, the vesicles enhanced the diversity and abundance of the intestinal microbiota, thereby preventing or alleviating inflammatory bowel disease and colitis-associated colon cancer.Citation117
In summary, the intricate interplay within the TME underscores the significance of angiogenesis as a potential therapeutic target. Preliminary findings highlight the inhibitory effects of GaDVEs on cancer growth through VEGF modulation. Yet, the journey to fully understand their potential is still underway, necessitating more rigorous studies. On another front, PDVEs are emerging as potent preventive agents against carcinogenesis. Their ability to modulate the inflammatory milieu, bolster barrier integrity, and foster a healthful gut microbiome place them at the forefront of innovative strategies against inflammatory diseases and associated cancers. The multipronged effects of PDVEs accentuate their vast potential in cancer research and treatment.
Discussion and Perspectives
Here, we systematically summarized the preparation, contents, and application of PDVEs in cancer prevention and treatment. Although SLNVEs meet the basic requirements for drug delivery,Citation118 they are still challenged by a number of problems such as cytotoxicity, non-specific tissue distribution, and therapeutic efficacy. SLNVEs tend to be phagocyted by the reticuloendothelial system, resulting in low bioavailability and hypersensitivity reactions.Citation119 In contrast, the natural properties of PDNVEs allow them to avoid these side effects. Compared to MDVEs, PDVEs have no zoonotic pathogens, can be manufactured from abundant resources, are easily extracted, and are environmentally friendly.Citation19
Although PDVEs have great potential in anticancer therapy, a series of issues remain to be solved. First, there is no standardization of PDVEs in the extraction process and no representative markers to qualify PDVEs. The differences in plant donor species make it difficult to establish quality control criteria for PDVEs. Macroscopic control can be performed based on commonalities of PDVEs, such as describing their shape, size, and potential, or identifying their compositions including lipids, proteins, and nucleic acids. For example, transmission electron microscopy is typically used to detect the ultrastructure of PDVEs, and dynamic light scattering is used to determine the size and potential of PDVEs. In addition, it is vital to identify the composition of PDVEs. Unlike other vesicles, PDVEs are composed of multiple lipids. By determining the lipid composition of the PDVEs extracted to date, it was found that each PDVE contains phospholipids and glycerol lipids, but none contain cholesterol.Citation120 It is known that vesicles of mammalian origin have well-defined protein markers, including the transmembrane proteins CD63, CD81, and CD9. PDVEs do not yet have clear protein markers, but all PDVEs inevitably require some hydrolase to cleave the cell wall during release,Citation121 and therefore, this hydrolase may be a candidate for protein markers.
Second, PDVEs may carry allergens from the donor. Stanly et al evaluated the allergen information carried in strawberry-derived vesicles.Citation122 Immunological testing revealed that several proteins in strawberry-derived vesicles, such as Fra a 1, Fra a 3, and Fra a 4, could competitively bind with immunoglobulin E, suggesting that PDVEs may induce allergic reactions in human beings. In addition to allergens, plant exposure to toxic substances during growth may also remain in the PDVEs,Citation78 such as organophosphorus, organonitrogen, organoarsenic, and dinitroaniline. Therefore, a strict selection of plant donors can effectively avoid potential risks, such as organic plants, in-season plants, and non-GMO plants.
In addition to the aforementioned lack of standardized extraction methods and potential allergenic issues associated with PDVEs, there are several other challenges confronting PDVE research: (1) The mechanisms of PDVE formation and release remain unclear; (2) There is no unified naming convention for PDVEs; (3) Standardized biomarkers for PDVEs are lacked; (4) Techniques for PDVE preservation and transportation are still in their infancy; (5) The distribution and metabolism of PDVEs within the body are not well-understood. Despite the burgeoning research in PDVEs, further efforts are required globally from the scientific community to fully harness its potential as a biological therapy or drug carrier. Specifically, it is necessary to elucidate the roles of PDVEs in plant growth, stress responses, and death, as well as the mechanisms underlying PDVE formation and release. Meanwhile, it is urgent to develop more cost-effective and time-saving extraction methods suitable for large-scale production, along with robust preservation and transportation techniques for clinical applications. In addition, we need to establish a comprehensive, intuitive, and easily adopted naming system that differentiates PDVEs based on type, origin, location within the plant, and state. More importantly, identification of generic biomarkers for PDVEs to standardize subsequent extractions is significant for mechanism exploration. Last but not least, the impacts of PDVEs, when introduced into the body via various administration routes, on critical systems such as the immune, hematological, and nervous systems, as well as their distribution patterns and metabolic pathways in the body, is worth to be intensively investigated on multiple diseases, such as malignancies and auto-immune diseases.
Finally, Traditional Chinese medicine (TCM) is a valuable resource to screen and prepare efficient PDVEs. TCM has been appreciated for cancer prevention and treatment in Asian countries for thousands of years. Although a number of formulas have been validated in clinical settings, the underlying molecular mechanisms remain unknown. In recent years, with the discovery of PDVEs, it was hypothesized that PDVEs are largely produced and precipitated from decoctions, and the proteins, nucleic acids, lipids, and metabolites in them may contribute to the therapeutic effects. The hypothesis is also supported by recent findings, which suggested that most of an herb’s active ingredients are covered in PDVEs.Citation123 It was found that ginger contained 237 miRNAs, 136 of which were found in GiDVEs and were mainly involved in modulating physiological processes such as a stress response. A total of 745 proteins were found in the vesicles from tea flower and were demonstrated to be crucial components responsible for biological processes including gene expression, signaling pathway transmission, and cellular metabolism.Citation98 Currently, characterization and molecular elucidation of PDVEs in herbs or formulas have become a topic of interest in TCM study.
Taken together, the available evidence suggests that PDVEs offer tremendous advantages in anti-cancer therapy, both as carriers and as therapeutic agents. PDVEs offer cancer patients a new strategy for targeted cytotoxic therapy, immunomodulatory therapy, oxidative therapy, as well as neoangiogenesis inhibition therapy. However, further research is still warranted to optimize PDVEs concerning their quality control, safety, and high-throughput production.
Author Contributions
All authors made a significant contribution to the work reported, whether that is in the conception, study design, execution, acquisition of data, analysis and interpretation, or in all these areas; took part in drafting, revising or critically reviewing the article; gave final approval of the version to be published; have agreed on the journal to which the article has been submitted; and agree to be accountable for all aspects of the work.
Disclosure
The authors report no conflicts of interest in this work.
Acknowledgments
This work was supported by the National Natural Science Foundation of China [82074165, 82374446, 81973526]; the State Key Laboratory of Dampness Syndrome of Chinese Medicine[SZ2021ZZ19]; Science and Technology Planning Project of Guangdong Province [2022B1515230002, 2021A0505030059, 2017B030314166]; The 2020 Guangdong Provincial Science and Technology Innovation Strategy Special Fund (Guangdong-Hong Kong-Macau Joint Lab) [2020B1212030006]; and the Foundation for Young Scholars of Guangzhou University of Chinese Medicine [QNYC20190101].
References
- Liu Y, Wang Y, Lv Q, Li X. Exosomes: from garbage bins to translational medicine. Int J Pharm. 2020;583:119333. doi:10.1016/j.ijpharm.2020.119333
- Halperin W, Jensen WA. Ultrastructural changes during growth and embryogenesis in carrot cell cultures. J Ultrastruct Res. 1967;18(3):428–443. doi:10.1016/s0022-5320(67)80128-x
- An Q, Ehlers K, Kogel KH, van Bel AJ, Hückelhoven R. Multivesicular compartments proliferate in susceptible and resistant MLA12-barley leaves in response to infection by the biotrophic powdery mildew fungus. New Phytol. 2006;172(3):563–576. doi:10.1111/j.1469-8137.2006.01844.x
- An Q, Hückelhoven R, Kogel KH, van Bel AJ. Multivesicular bodies participate in a cell wall-associated defence response in barley leaves attacked by the pathogenic powdery mildew fungus. Cell Microbiol. 2006;8(6):1009–1019. doi:10.1111/j.1462-5822.2006.00683.x
- Alfieri M, Leone A, Ambrosone A. Plant-derived nano and microvesicles for human health and therapeutic potential in nanomedicine. Pharmaceutics. 2021;13(4):498. doi:10.3390/pharmaceutics13040498
- Garaeva L, Kamyshinsky R, Kil Y, et al. Delivery of functional exogenous proteins by plant-derived vesicles to human cells in vitro. Sci Rep. 2021;11(1). doi:10.1038/s41598-021-85833-y
- Mu J, Zhuang X, Wang Q, et al. Interspecies communication between plant and mouse gut host cells through edible plant derived exosome‐like nanoparticles. Mol Nutr Food Res. 2014;58(7):1561–1573. doi:10.1002/mnfr.201300729
- Fujita D, Arai T, Komori H, et al. Apple-derived nanoparticles modulate expression of organic-anion-transporting polypeptide (OATP) 2B1 in caco-2 cells. Mol Pharm. 2018;15(12):5772–5780. doi:10.1021/acs.molpharmaceut.8b00921
- Bokka R, Ramos AP, Fiume I, et al. Biomanufacturing of tomato-derived nanovesicles. Foods. 2020;9(12):1852. doi:10.3390/foods9121852
- Hinshaw DC, Shevde LA. The tumor microenvironment innately modulates cancer progression. Cancer Res. 2019;79(18):4557–4566. doi:10.1158/0008-5472.Can-18-3962
- Boedtkjer E, Pedersen SF. The acidic tumor microenvironment as a driver of cancer. Annu Rev Physiol. 2020;82:103–126. doi:10.1146/annurev-physiol-021119-034627
- Greten FR, Grivennikov SI. Inflammation and cancer: triggers, mechanisms, and consequences. Immunity. 2019;51(1):27–41. doi:10.1016/j.immuni.2019.06.025
- Jiang X, Wang J, Deng X, et al. The role of microenvironment in tumor angiogenesis. J Exp Clin Cancer Res. 2020;39(1):204. doi:10.1186/s13046-020-01709-5
- Lee Y, Thompson DH. Stimuli-responsive liposomes for drug delivery. Wiley Interdiscip Rev Nanomed Nanobiotechnol. 2017;9(5). doi:10.1002/wnan.1450
- Bruno SP, Paolini A, D’Oria V, et al. Extracellular vesicles derived from citrus sinensis modulate inflammatory genes and tight junctions in a human model of intestinal epithelium. Front Nutrit. 2021:8. doi:10.3389/fnut.2021.778998
- Kumar A, Sundaram K, Teng Y, et al. Ginger nanoparticles mediated induction of Foxa2 prevents high-fat diet-induced insulin resistance. Theranostics. 2022;12(3):1388–1403. doi:10.7150/thno.62514
- Zhang M, Xiao B, Wang H, et al. Edible ginger-derived nano-lipids loaded with doxorubicin as a novel drug-delivery approach for colon cancer therapy. Mol Ther. 2016;24(10):1783–1796. doi:10.1038/mt.2016.159
- Mao Y, Han M, Chen C, et al. A biomimetic nanocomposite made of a ginger-derived exosome and an inorganic framework for high-performance delivery of oral antibodies. Nanoscale. 2021;13(47):20157–20169. doi:10.1039/d1nr06015e
- Cai Y, Zhang L, Zhang Y, Lu R. Plant-derived exosomes as a drug-delivery approach for the treatment of inflammatory bowel disease and colitis-associated cancer. Pharmaceutics. 2022;14(4):822. doi:10.3390/pharmaceutics14040822
- Karamanidou T, Tsouknidas A. Plant-derived extracellular vesicles as therapeutic nanocarriers. Int J Mol Sci. 2021;23(1):191. doi:10.3390/ijms23010191
- Han JM, Song H-Y, Lim S-T, Kim K-I, Seo HS, Byun E-B. Immunostimulatory potential of extracellular vesicles isolated from an edible plant, petasites japonicus, via the induction of murine dendritic cell maturation. Int J Mol Sci. 2021;22(19):10634. doi:10.3390/ijms221910634
- Yang M, Luo Q, Chen X, Chen F. Bitter melon derived extracellular vesicles enhance the therapeutic effects and reduce the drug resistance of 5-fluorouracil on oral squamous cell carcinoma. J Nanobiotechnology. 2021;19(1):259. doi:10.1186/s12951-021-00995-1
- Özkan İ, Koçak P, Yıldırım M, et al. Garlic (Allium sativum)-derived SEVs inhibit cancer cell proliferation and induce caspase mediated apoptosis. Sci Rep. 2021;11(1). doi:10.1038/s41598-021-93876-4
- Crommelin DJA, van Hoogevest P, Storm G. The role of liposomes in clinical nanomedicine development. What now? Now what? J Control Release. 2020;318:256–263. doi:10.1016/j.jconrel.2019.12.023
- Grimaldi N, Andrade F, Segovia N, et al. Lipid-based nanovesicles for nanomedicine. Chem Soc Rev. 2016;45(23):6520–6545. doi:10.1039/c6cs00409a
- Sonju JJ, Dahal A, Singh SS, Jois SD. Peptide-functionalized liposomes as therapeutic and diagnostic tools for cancer treatment. J Control Release. 2021;329:624–644. doi:10.1016/j.jconrel.2020.09.055
- Li X, Diao W, Xue H, et al. Improved efficacy of doxorubicin delivery by a novel dual-ligand-modified liposome in hepatocellular carcinoma. Cancer Lett. 2020;489:163–173. doi:10.1016/j.canlet.2020.06.017
- Hu M, Palić D. Micro- and nano-plastics activation of oxidative and inflammatory adverse outcome pathways. Redox Biol. 2020;37:101620. doi:10.1016/j.redox.2020.101620
- Yue X, Dai Z. Recent advances in liposomal nanohybrid cerasomes as promising drug nanocarriers. Adv Colloid Interface Sci. 2014;207:32–42. doi:10.1016/j.cis.2013.11.014
- Raemdonck K, Braeckmans K, Demeester J, De Smedt SC. Merging the best of both worlds: hybrid lipid-enveloped matrix nanocomposites in drug delivery. Chem Soc Rev. 2014;43(1):444–472. doi:10.1039/c3cs60299k
- Radmanesh F, Razi M, Shalizar-Jalali A. Curcumin nano-micelle induced testicular toxicity in healthy rats; evidence for oxidative stress and failed homeostatic response by heat shock proteins 70-2a and 90. Biomed Pharmacother. 2021;142:111945. doi:10.1016/j.biopha.2021.111945
- Lancet JE, Uy GL, Cortes JE, et al. CPX-351 (cytarabine and daunorubicin) liposome for injection versus conventional cytarabine plus daunorubicin in older patients with newly diagnosed secondary acute myeloid leukemia. J Clin Oncol. 2018;36(26):2684–2692. doi:10.1200/jco.2017.77.6112
- Anand U, Carpena M, Kowalska-Góralska M, et al. Safer plant-based nanoparticles for combating antibiotic resistance in bacteria: a comprehensive review on its potential applications, recent advances, and future perspective. Sci Total Enviro. 2022:821. doi:10.1016/j.scitotenv.2022.153472
- Feng J, Xiu Q, Huang Y, Troyer Z, Li B, Zheng L. Plant-derived vesicle-like nanoparticles as promising biotherapeutic tools: present and future. Adv Mater. 2023;35(24):e2207826. doi:10.1002/adma.202207826
- Ju S, Mu J, Dokland T, et al. Grape exosome-like nanoparticles induce intestinal stem cells and protect mice from DSS-induced colitis. Molecular Therapy. 2013;21(7):1345–1357. doi:10.1038/mt.2013.64
- Wang Q, Zhuang X, Mu J, et al. Delivery of therapeutic agents by nanoparticles made of grapefruit-derived lipids. Nat Commun. 2013;4:1867. doi:10.1038/ncomms2886
- Pomatto MAC, Bussolati B, D’Antico S, et al. Improved loading of plasma-derived extracellular vesicles to encapsulate antitumor miRNAs. Mol Ther Methods Clin Dev. 2019;13:133–144. doi:10.1016/j.omtm.2019.01.001
- Li Y, Gao Y, Gong C, et al. A33 antibody-functionalized exosomes for targeted delivery of doxorubicin against colorectal cancer. Nanomedicine. 2018;14(7):1973–1985. doi:10.1016/j.nano.2018.05.020
- Xiao L, Erb U, Zhao K, Hackert T, Zoller M. Efficacy of vaccination with tumor-exosome loaded dendritic cells combined with cytotoxic drug treatment in pancreatic cancer. Oncoimmunology. 2017;6(6):e1319044–1319088. doi:10.1080/2162402X.2017.1319044
- Sheng S, Yu X, Xing G, et al. An apoptotic body-based vehicle with navigation for photothermal-immunotherapy by precise delivery and tumor microenvironment regulation. Adv Funct Mater. 2023;33(5):2212118. doi:10.1002/adfm.202212118
- Liang T, Wen D, Chen G, et al. Adipocyte-derived anticancer lipid droplets. Adv Mater. 2021;33(26):2100629. doi:10.1002/adma.202100629
- Yu Y, Cheng Q, Ji X, et al. Engineered drug-loaded cellular membrane nanovesicles for efficient treatment of postsurgical cancer recurrence and metastasis. Sci Adv. 2022;8(49):eadd3599. doi:10.1126/sciadv.add3599
- Wang Y, Xie Y, Zhang A, Wang M, Fang Z, Zhang J. Exosomes: an emerging factor in atherosclerosis. Biomed Pharmacother. 2019;115:108951. doi:10.1016/j.biopha.2019.108951
- Wang B, Zhuang X, Deng ZB, et al. Targeted drug delivery to intestinal macrophages by bioactive nanovesicles released from grapefruit. Mol Ther. 2014;22(3):522–534. doi:10.1038/mt.2013.190
- Cong M, Tan S, Li S, et al. Technology insight: plant-derived vesicles-How far from the clinical biotherapeutics and therapeutic drug carriers? Adv Drug Deliv Rev. 2022;182:114108. doi:10.1016/j.addr.2021.114108
- Stanly C, Moubarak M, Fiume I, Turiak L, Pocsfalvi G. Membrane transporters in citrus clementina fruit juice-derived nanovesicles. Int J Mol Sci. 2019;20(24):6205. doi:10.3390/ijms20246205
- Baldini N, Torreggiani E, Roncuzzi L, Perut F, Zini N, Avnet S. Exosome-like nanovesicles isolated from citrus limon l. Exert antioxidative effect. Curr Pharm Biotechnol. 2018;19(11):877–885. doi:10.2174/1389201019666181017115755
- Pocsfalvi G, Turiák L, Ambrosone A, et al. Protein biocargo of citrus fruit-derived vesicles reveals heterogeneous transport and extracellular vesicle populations. J Plant Physiol. 2018;229:111–121. doi:10.1016/j.jplph.2018.07.006
- Chen X, Liu B, Li X, et al. Identification of anti-inflammatory vesicle-like nanoparticles in honey. J Extracell Vesicles. 2021;10(4):e12069. doi:10.1002/jev2.12069
- Stanly C, Fiume I, Capasso G, Pocsfalvi G. Isolation of exosome-like vesicles from plants by ultracentrifugation on sucrose/deuterium oxide (D2O) density cushions. Methods Mol Biol. 2016;1459:259–269. doi:10.1007/978-1-4939-3804-9_18
- Li P, Kaslan M, Lee SH, Yao J, Gao Z. Progress in Exosome Isolation Techniques. Theranostics. 2017;7(3):789–804. doi:10.7150/thno.18133
- Chen X, Zhou Y, Yu J. Exosome-like nanoparticles from ginger rhizomes inhibited NLRP3 inflammasome activation. Mol Pharm. 2019;16(6):2690–2699. doi:10.1021/acs.molpharmaceut.9b00246
- You JY, Kang SJ, Rhee WJ. Isolation of cabbage exosome-like nanovesicles and investigation of their biological activities in human cells. Bio Mater. 2021;6(12):4321–4332. doi:10.1016/j.bioactmat.2021.04.023
- Woith E, Melzig MF. Extracellular vesicles from fresh and dried plants-simultaneous purification and visualization using gel electrophoresis. Int J Mol Sci. 2019;20(2):357. doi:10.3390/ijms20020357
- Kalarikkal SP, Prasad D, Kasiappan R, Chaudhari SR, Sundaram GM. A cost-effective polyethylene glycol-based method for the isolation of functional edible nanoparticles from ginger rhizomes. Sci Rep. 2020;10(1). doi:10.1038/s41598-020-61358-8
- Kang YT, Hadlock T, Lo TW, et al. Dual-isolation and profiling of circulating tumor cells and cancer exosomes from blood samples with melanoma using immunoaffinity-based microfluidic interfaces. Advan Sci. 2020;7(19):2001581. doi:10.1002/advs.202001581
- Yang M, Liu X, Luo Q, Xu L, Chen F. An efficient method to isolate lemon derived extracellular vesicles for gastric cancer therapy. J Nanobiotechnology. 2020;18(1):100. doi:10.1186/s12951-020-00656-9
- Moura RP, Pacheco C, Pêgo AP, Des Rieux A, Sarmento B. Lipid nanocapsules to enhance drug bioavailability to the central nervous system. J Control Release. 2020;322:390–400. doi:10.1016/j.jconrel.2020.03.042
- Raimondo S, Urzì O, Meraviglia S, et al. Anti-inflammatory properties of lemon-derived extracellular vesicles are achieved through the inhibition of ERK /NF-κB signalling pathways. J Cell Mol Med. 2022;26:4195–4209. doi:10.1111/jcmm.17404
- Sundaram K, Miller DP, Kumar A, et al. Plant-derived exosomal nanoparticles inhibit pathogenicity of porphyromonas gingivalis. iScience. 2019;21:308–327. doi:10.1016/j.isci.2019.10.032
- Perut F, Roncuzzi L, Avnet S, et al. Strawberry-derived exosome-like nanoparticles prevent oxidative stress in human mesenchymal stromal cells. Biomolecules. 2021;11(1):87. doi:10.3390/biom11010087
- Peršurić Ž, Pavelić SK. Bioactives from bee products and accompanying extracellular vesicles as novel bioactive components for wound healing. Molecules. 2021;26(12):3770. doi:10.3390/molecules26123770
- Lee R, Ko HJ, Kim K, et al. Anti‐melanogenic effects of extracellular vesicles derived from plant leaves and stems in mouse melanoma cells and human healthy skin. J Extracell Vesicles. 2019;9(1). doi:10.1080/20013078.2019.1703480
- Raimondo S, Naselli F, Fontana S, et al. Citrus limon-derived nanovesicles inhibit cancer cell proliferation and suppress CML xenograft growth by inducing TRAIL-mediated cell death. Oncotarget. 2015;6(23):19514–19527. doi:10.18632/oncotarget.4004
- Deng Z, Rong Y, Teng Y, et al. Broccoli-derived nanoparticle inhibits mouse colitis by activating dendritic cell AMP-activated protein kinase. Mol Ther. 2017;25(7):1641–1654. doi:10.1016/j.ymthe.2017.01.025
- Zhuang X, Deng ZB, Mu J, et al. Ginger-derived nanoparticles protect against alcohol-induced liver damage. J Extracell Vesicles. 2015;4:28713. doi:10.3402/jev.v4.28713
- Kalarikkal SP, Sundaram GM. Edible plant-derived exosomal microRNAs: exploiting a cross-kingdom regulatory mechanism for targeting SARS-CoV-2. Toxicol Appl Pharmacol. 2021;414:115425. doi:10.1016/j.taap.2021.115425
- Teng Y, Ren Y, Sayed M, et al. Plant-derived exosomal MicroRNAs shape the gut microbiota. Cell Host Microbe. 2018;24(5):637–652.e8. doi:10.1016/j.chom.2018.10.001
- Raza K, Nemidkanam V, Chaichanawongsaroj N, Raza K, Khan YA. Characterizing Kaempferia parviflora extracellular vesicles, a nanomedicine candidate. PLoS One. 2022;17(1). doi:10.1371/journal.pone.0262884
- Kim K, Park J, Sohn Y, et al. Stability of plant leaf-derived extracellular vesicles according to preservative and storage temperature. Pharmaceutics. 2022;14(2). doi:10.3390/pharmaceutics14020457
- Vader P, Mol EA, Pasterkamp G, Schiffelers RM. Extracellular vesicles for drug delivery. Adv Drug Deliv Rev. 2016;106(Pt A):148–156. doi:10.1016/j.addr.2016.02.006
- Mahdipour E. Beta vulgaris juice contains biologically active exosome-like nanoparticles. Tissue Cell. 2022;76:101800. doi:10.1016/j.tice.2022.101800
- Zhang L, He F, Gao L, et al. Engineering exosome-like nanovesicles derived from asparagus cochinchinensis can inhibit the proliferation of hepatocellular carcinoma cells with better safety profile. Int J Nanomedicine. 2021;16:1575–1586. doi:10.2147/IJN.S293067
- Cao M, Yan H, Han X, et al. Ginseng-derived nanoparticles alter macrophage polarization to inhibit melanoma growth. J Immunother Cancer. 2019;7(1):326. doi:10.1186/s40425-019-0817-4
- Kim J, Li S, Zhang S, Wang J. Plant-derived exosome-like nanoparticles and their therapeutic activities. Asian J Pharm Sci. 2022;17(1):53–69. doi:10.1016/j.ajps.2021.05.006
- Trentini M, Zanotti F, Tiengo E, et al. An apple a day keeps the doctor away: potential role of miRNA 146 on macrophages treated with exosomes derived from apples. Biomedicines. 2022;10(2):415. doi:10.3390/biomedicines10020415
- Rutter BD, Innes RW. Extracellular vesicles isolated from the leaf apoplast carry stress-response proteins. Plant Physiol. 2017;173(1):728–741. doi:10.1104/pp.16.01253
- Logozzi M, Di Raimo R, Mizzoni D, Fais S. Nanovesicles from organic agriculture-derived fruits and vegetables: characterization and functional antioxidant content. Int J Mol Sci. 2021;22(15):8170. doi:10.3390/ijms22158170
- De Robertis M, Sarra A, V D, et al. Blueberry-derived exosome-like nanoparticles counter the response to TNF-α-induced change on gene expression in EA.hy926 cells. Biomolecules. 2020;10(5):742. doi:10.3390/biom10050742
- Xiao J, Feng S, Wang X, et al. Identification of exosome-like nanoparticle-derived microRNAs from 11 edible fruits and vegetables. PeerJ. 2018;6:e5186. doi:10.7717/peerj.5186
- Kim DK, Rhee WJ. Antioxidative effects of carrot-derived nanovesicles in cardiomyoblast and neuroblastoma cells. Pharmaceutics. 2021;13(8):1203. doi:10.3390/pharmaceutics13081203
- Zhao Z, Yu S, Li M, Gui X, Li P. Isolation of exosome-like nanoparticles and analysis of MicroRNAs derived from coconut water based on small RNA high-throughput sequencing. J Agric Food Chem. 2018;66(11):2749–2757. doi:10.1021/acs.jafc.7b05614
- Abraham AM, Wiemann S, Ambreen G, et al. Cucumber-derived exosome-like vesicles and plantcrystals for improved dermal drug delivery. Pharmaceutics. 2022;14(3):476. doi:10.3390/pharmaceutics14030476
- Liu B, Li X, Yu H, et al. Therapeutic potential of garlic chive-derived vesicle-like nanoparticles in NLRP3 inflammasome-mediated inflammatory diseases. Theranostics. 2021;11(19):9311–9330. doi:10.7150/thno.60265
- Li Z, Wang H, Yin H, Bennett C, Zhang HG, Guo P. Arrowtail RNA for ligand display on ginger exosome-like nanovesicles to systemic deliver siRNA for cancer suppression. Sci Rep. 2018;8(1):14644. doi:10.1038/s41598-018-32953-7
- Zhang M, Collins JF, Merlin D. Do ginger-derived nanoparticles represent an attractive treatment strategy for inflammatory bowel diseases? Nanomedicine. 2016;11(23):3035–3037. doi:10.2217/nnm-2016-0353
- Zhang M, Viennois E, Prasad M, et al. Edible ginger-derived nanoparticles: a novel therapeutic approach for the prevention and treatment of inflammatory bowel disease and colitis-associated cancer. Biomaterials. 2016;101:321–340. doi:10.1016/j.biomaterials.2016.06.018
- Zhang M, Wang X, Han MK, Collins JF, Merlin D. Oral administration of ginger-derived nanolipids loaded with siRNA as a novel approach for efficient siRNA drug delivery to treat ulcerative colitis. Nanomedicine. 2017;12(16):1927–1943. doi:10.2217/nnm-2017-0196
- Cho EG, Choi SY, Kim H, et al. Panax ginseng-derived extracellular vesicles facilitate anti-senescence effects in human skin cells: an eco-friendly and sustainable way to use ginseng substances. Cells. 2021;10(3):486. doi:10.3390/cells10030486
- Pérez-Bermúdez P, Blesa J, Soriano JM, Marcilla A. Extracellular vesicles in food: experimental evidence of their secretion in grape fruits. Eur J Pharm Sci. 2017;98:40–50. doi:10.1016/j.ejps.2016.09.022
- Teng Y, Mu J, Hu X, et al. Grapefruit-derived nanovectors deliver miR-18a for treatment of liver metastasis of colon cancer by induction of M1 macrophages. Oncotarget. 2016;7(18):25683–25697. doi:10.18632/oncotarget.8361
- Zhuang X, Teng Y, Samykutty A, et al. Grapefruit-derived nanovectors delivering therapeutic miR17 through an intranasal route inhibit brain tumor progression. Mol Ther. 2016;24(1):96–105. doi:10.1038/mt.2015.188
- Wang Q, Ren Y, Mu J, et al. Grapefruit-derived nanovectors use an activated leukocyte trafficking pathway to deliver therapeutic agents to inflammatory tumor sites. Cancer Res. 2015;75(12):2520–2529. doi:10.1158/0008-5472.CAN-14-3095
- Lei C, Teng Y, He L, et al. Lemon exosome-like nanoparticles enhance stress survival of gut bacteria by RNase P-mediated specific tRNA decay. iScience. 2021;24(6):102511. doi:10.1016/j.isci.2021.102511
- Aquilano K, Ceci V, Gismondi A, et al. Adipocyte metabolism is improved by TNF receptor-targeting small RNAs identified from dried nuts. Communic Bio. 2019;2:317. doi:10.1038/s42003-019-0563-7
- Regente M, Corti-Monzón G, Maldonado AM, Pinedo M, Jorrín J, de la Canal L. Vesicular fractions of sunflower apoplastic fluids are associated with potential exosome marker proteins. FEBS Lett. 2009;583(20):3363–3366. doi:10.1016/j.febslet.2009.09.041
- Johnstone RW, Frew AJ, Smyth MJ. The TRAIL apoptotic pathway in cancer onset, progression and therapy. Nat Rev Cancer. 2008;8(10):782–798. doi:10.1038/nrc2465
- Chen Q, Li Q, Liang Y, et al. Natural exosome-like nanovesicles from edible tea flowers suppress metastatic breast cancer via ROS generation and microbiota modulation. Acta Pharmaceutica Sinica B. 2022;12(2):907–923. doi:10.1016/j.apsb.2021.08.016
- Fang Z, Song M, Lai K, Cui M, Yin M, Liu K. Kiwi-derived extracellular vesicles for oral delivery of sorafenib. Eur J Pharm Sci. 2023;191:106604. doi:10.1016/j.ejps.2023.106604
- Langen UH, Ayloo S, Gu C. Development and cell biology of the blood-brain barrier. Annu Rev Cell Dev Biol. 2019;35:591–613. doi:10.1146/annurev-cellbio-100617-062608
- Ishida T, Kawada K, Jobu K, et al. Exosome-like nanoparticles derived from Allium tuberosum prevent neuroinflammation in microglia-like cells. J Pharm Pharmacol. 2023;75(10):1322–1331. doi:10.1093/jpp/rgad062
- Zhang M, Viennois E, Xu C, Merlin D. Plant derived edible nanoparticles as a new therapeutic approach against diseases. Tissue Barriers. 2016;4(2):e1134415. doi:10.1080/21688370.2015.1134415
- Yin L, Yan L, Yu Q, et al. Characterization of the MicroRNA profile of ginger exosome-like nanoparticles and their anti-inflammatory effects in intestinal caco-2 cells. J Agric Food Chem. 2022;70(15):4725–4734. doi:10.1021/acs.jafc.1c07306
- Sriwastva MK, Deng ZB, Wang B, et al. Exosome-like nanoparticles from Mulberry bark prevent DSS-induced colitis via the AhR/COPS8 pathway. EMBO Rep. 2022;23(3):e53365. doi:10.15252/embr.202153365
- Kim K, Yoo HJ, Jung JH, et al. Cytotoxic effects of plant sap-derived extracellular vesicles on various tumor cell types. J Funct Biomater. 2020;11(2). doi:10.3390/jfb11020022
- Potestà M, Minutolo A, Gismondi A, et al. Cytotoxic and apoptotic effects of different extracts of Moringa oleifera Lam on lymphoid and monocytoid cells. Exp Ther Med. 2019;18(1):5–17. doi:10.3892/etm.2019.7544
- Tajik T, Baghaei K, Moghadam VE, Farrokhi N, Salami SA. Extracellular vesicles of cannabis with high CBD content induce anticancer signaling in human hepatocellular carcinoma. Biomed Pharmacother. 2022;152. doi:10.1016/j.biopha.2022.113209
- Han X, Wei Q, Lv Y, et al. Ginseng-derived nanoparticles potentiate immune checkpoint antibody efficacy by reprogramming the cold tumor microenvironment. Mol Ther. 2022;30(1):327–340. doi:10.1016/j.ymthe.2021.08.028
- Rahimi Ghiasi M, Rahimi E, Amirkhani Z, Salehi R. Leucine-rich repeat-containing G-protein coupled receptor 5 gene overexpression of the rat small intestinal progenitor cells in response to orally administered grape exosome-like nanovesicles. Adv Biomed Res. 2018;7:125. doi:10.4103/abr.abr_114_18
- Stanly C, Alfieri M, Ambrosone A, Leone A, Fiume I, Pocsfalvi G. Grapefruit-derived micro and nanovesicles show distinct metabolome profiles and anticancer activities in the A375 human melanoma cell line. Cells. 2020;9(12):2722. doi:10.3390/cells9122722
- Tang Z, Jun Y, Lv Y, et al. Aptamer-conjugated and doxorubicin-loaded grapefruit-derived nanovectors for targeted therapy against HER2(+) breast cancer. J Drug Target. 2020;28(2):186–194. doi:10.1080/1061186x.2019.1624970
- Tamura RE, de Vasconcellos JF, Sarkar D, Libermann TA, Fisher PB, Zerbini LF. GADD45 proteins: central players in tumorigenesis. Curr Mol Med. 2012;12(5):634–651. doi:10.2174/156652412800619978
- Nesterov A, Nikrad M, Johnson T, Kraft AS. Oncogenic Ras sensitizes normal human cells to tumor necrosis factor-alpha-related apoptosis-inducing ligand-induced apoptosis. Cancer Res. 2004;64(11):3922–3927. doi:10.1158/0008-5472.Can-03-2219
- Pan Y, Yu Y, Wang X, Zhang T. Tumor-associated macrophages in tumor immunity. Front Immunol. 2020;11:583084. doi:10.3389/fimmu.2020.583084
- Wu K, Lin K, Li X, et al. Redefining tumor-associated macrophage subpopulations and functions in the tumor microenvironment. Front Immunol. 2020;11:1731. doi:10.3389/fimmu.2020.01731
- Cui -W-W, Ye C, Wang K-X, et al. Momordica. charantia-derived extracellular vesicles-like nanovesicles protect cardiomyocytes against radiation injury via attenuating DNA damage and mitochondria dysfunction. Front Cardiovasc Med. 2022:9. doi:10.3389/fcvm.2022.864188
- Zu M, Xie D, Canup BSB, et al. ‘Green’ nanotherapeutics from tea leaves for orally targeted prevention and alleviation of colon diseases. Biomaterials. 2021;279:121178. doi:10.1016/j.biomaterials.2021.121178
- Srivastava A, Rathore S, Munshi A, Ramesh R. Organically derived exosomes as carriers of anticancer drugs and imaging agents for cancer treatment. Semin Cancer Biol. 2022;86:80–100. doi:10.1016/j.semcancer.2022.02.020
- Asghar S, Khan IU, Salman S, Khalid SH, Ashfaq R, Vandamme TF. Plant-derived nanotherapeutic systems to counter the overgrowing threat of resistant microbes and biofilms. Adv Drug Deliv Rev. 2021;179. doi:10.1016/j.addr.2021.114019
- Dad HA, Gu TW, Zhu AQ, Huang LQ, Peng LH. Plant exosome-like nanovesicles: emerging therapeutics and drug delivery nanoplatforms. Mol Ther. 2021;29(1):13–31. doi:10.1016/j.ymthe.2020.11.030
- Woith E, Guerriero G, Hausman JF, et al. Plant extracellular vesicles and nanovesicles: focus on secondary metabolites, proteins and lipids with perspectives on their potential and sources. Int J Mol Sci. 2021;22(7):3719. doi:10.3390/ijms22073719
- Stanly C, Kim H, Antonucci G, et al. Crosstalk between the immune system and plant-derived nanovesicles: a study of allergen transporting. Front Bioeng Biotechnol. 2021:9. doi:10.3389/fbioe.2021.760730
- Man F, Meng C, Liu Y, et al. The study of ginger-derived extracellular vesicles as a natural nanoscale drug carrier and their intestinal absorption in rats. AAPS Pharm Sci Tech. 2021;22(6). doi:10.1208/s12249-021-02087-7