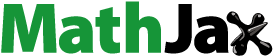
Abstract
Background and methods
Guided bone regeneration (GBR) is a new treatment for bone defects, and the property of membrane is critical to the success of GBR. This study focuses on a novel membrane tube for GBR, which was prepared by a nanocalcium-deficient hydroxyapatite–multi(amino acid) copolymer (n-CDHA-MAC) composite. The biomechanical strength and degradability of this membrane tube under mechanical loading after immersion in phosphate-buffered solution were investigated to evaluate the effects of mechanical loading on the membrane tube. The membrane-tube group with no mechanical loading and femora bone were used as controls.
Results
The compressive strength and bending strength of n-CDHA-MAC membrane tubes were 66.4 ± 10.2 MPa and 840.7 ± 12.1 MPa, which were lower than those of the goats’ femoral bones (69.0 ± 5.5 MPa and 900.2 ± 17.3 MPa), but there were no significant (P > 0.05) differences. In the in vitro degradability experiment, all membrane tubes were degradable and showed a surface-erosion degradation model. The PH of solution fluctuated from 7.2 to 7.5. The weight and mechanical strength of loaded tubes decreased more quickly than nonloaded ones, with significant differences (P < 0.05). However, the strength of the loaded group after degradation achieved 20.4 ± 1.2 MPa, which was greater than the maximum mechanical strength of 4.338 MPa based on goat femoral middle stationary state by three-dimensional finite-element analysis.
Conclusions
n-CDHA-MAC membrane tubes have good biomechanical strength during degradation under mechanical loading. Therefore, this membrane tube is an ideal GBR membrane for critical size defects of long bones in goats for animal experiments.
Introduction
In the orthopedic setting, treatment for large bone defects, which includes segmental or large cortical defects caused by trauma, infection, tumor resection, aseptic loosening around implants, and skeletal abnormalities, represents a great challenge. Bone grafting is the main treatment for large bone defects, which can be divided into bone autografts and allografts, biological artificial materials, and membrane-tube techniques. As is well known, due to limited resources in autografts it is difficult to meet the demands for large bone defects. Because of lower osteogenic capacity, rejection, and spreading infection, allografts are restricted in clinical practice. Hence, it is very important to develop and design a bone-substitute material.
In recent years, membrane-guided tissue regeneration as a kind of new-regeneration technology has become a new area of research in bone-reconstruction surgery.Citation1,Citation2 The concept of membrane-guided bone regeneration (MGBR) involves using a bioabsorbable or nonresorbable membrane that acts as a barrier to prevent soft-tissue invasion into the bone defect and forms a chamber to guide the bone-regeneration process.Citation3 The characteristics of these bioabsorbable or nonresorbable membranes are critical to the success of MGBR. Until now, most membrane materials, including expanded polytetrafluoroethylene, poly-L-lactic acid, poly(lactide-co-glycolic acid), and alginate membranes, have lacked initial mechanical strength and easily collapsed under the pressure of surrounding muscles and soft tissues.Citation4,Citation5 It is difficult to keep enough tube volume and a sufficient amount of new bone formation to repair bone defects, especially where there are large segmental defects of the limb. At the same time, most membranes lack mechanical stability, and have complex mechanical loads inducing membrane distortion, displacement, and loosening, which makes it difficult to protect new bone regeneration and remodeling.Citation6–Citation8
Recently, a nanocalcium-deficient hydroxyapatite–multi(amino acid) copolymer (n-CDHA-MAC) composite was developed.Citation9 This n-CDHA-MAC is a novel degradable composite with good mechanical strength and suitable degradation rate, in which compressive strength is close to natural human cortical bone.Citation9 Therefore, the membrane tube of the n-CDHA-MAC has great potential to keep enough tube volume in GBR. Biomaterials under mechanical load showed a more rapid degradation rate compared with biomaterials under static conditions. Consequently, it is necessary to investigate the degradation behavior of the n-CDHA-MAC membrane tube under mechanical loading.Citation9 Goats’ limb bones are the most similar ones to humans’, and this study is based on large segmental defects of goats.
The purpose of this paper is to evaluate the effects of mechanical strength and degradability of these n-CDHA-MAC membrane tubes under mechanical loading, and to provide relevant data for further research in goats for animal experiments.
Materials and methods
n-CDHA-MAC was synthesized by an in situ polymerization method, as previously described in detail.Citation9,Citation19 The femur length and thickness were measured in six fresh femurs of 2-year-old goats (of either sex and either side of femur) to make the 30 mm-segment bone defects and measure the diameter of proximal and distal ends. Femur length from the intertrochanteric fossa to the midpoint of the medial and lateral condyle were measured as the standard length. The measurement results of the goat femur anatomical parameters are shown in .
Table 1 The results of goat femur anatomical parameters (n = 6)
Mechanical experiment of n-CDHA-MAC straight membrane tubes
Twelve straight membrane tubes were designed for the experimental group, prepared according to the anatomical parameters of goat femur (length of tube 70 mm, inner diameter 17 mm, tube-wall thickness 3 mm), while twelve goats’ mid-femoral cortical bone segments from which the bone marrow had been removed (the same size as straight membrane tube) served as the control group. Six straight membrane tubes and six goat femurs were taken for axial compression tests, and others for three-point bending tests. The biomechanics tests were carried out on a universal mechanical testing machine (Reger 3050; Shenzhen Reger Instrument, Shenzhen, People’s Republic of China). All tests were conducted at room temperature, and the measurements of samples were run at 5 mm/minute. The stress–strain curve was recorded. The pressure signal was recorded and analyzed by a computer data-acquisition system.
In vitro degradability experiments in phosphate-buffered solution
According to the mechanical analysis of lower-extremity load-bearing of goats,Citation10 a single limb bearing capacity was approximately a quarter of the animal’s weight. The weight of a 2-year-old goat was approximately 16–20 kg. Therefore, the 40 N and 50 N physiological mechanical loaded groups were defined as the experimental groups, and the nonloaded group was defined as the control group. A specially designed loading device () was made up of a metal rod with screw thread, Plexiglas support plate, stainless steel spring, locking nut, and pressure plate, which could provide axial compression loading to tubes. Shimadzu (Kyoto, Japan) Autograph in universal mechanical testing machine pressure probe was placed on the pressure-plate center, the pressure plate was paralleled with the experimental stage, and pressure was gradually increased according to the value on the display until 40 ± 0.5 N or 50 ± 0.5 N. To maintain the stability of the loading force, the nut on the metal rod was screwed tightly and fixed by denture powder, and then the compressed spring could provide continuous mechanical loading on the membrane tubes. The experimental group samples were divided into two groups by different loading (40 N/50 N). Each group had 15 samples that were fastened on the loading device and placed in a glass vessel containing phosphate-buffered saline (PBS; pH = 7.4 ± 0.2). Fifteen nonloaded samples (control group) were placed in the same glass vessel containing PBS as the experimental group. The degradation of membrane tubes was carried out in a shaking water bath (37.0°C and 30 rpm). The PBS was replenished every 2 weeks. The pH of the PBS was measured before being refreshed every 2 weeks using an electrolyte-type pH meter (PS-25; Leici Instrument Factory, Shanghai, People’s Republic of China). The color of the solution, and the presence or absence of other substance precipitation changes were observed in the degradation period. Scanning electron microscopy (SEM) was used to observe membrane tubes’ surface-morphology changes in each group every month randomly. Two experimental groups and the control group were detected in the internal cross-section changes by SEM and infrared analysis before degradation, after 1, 2, and 3 months’ degradation. Six samples of three groups (two samples from each group) were washed and dried every month before the residual weight (W) was calculated. The weight-loss ratio was calculated according to the following equation:
Figure 1 Schematic of self-designed loading device (left) and photo of device (right). The membrane tubes’ mechanical strength changed: after degradation of 3 months, six membrane tubes of each group were dried naturally in air, and then axial compression and three-point bending-stress tests were performed. The stress–strain curve was recorded. The pressure signal was recorded and analyzed by a computer data-acquisition system.
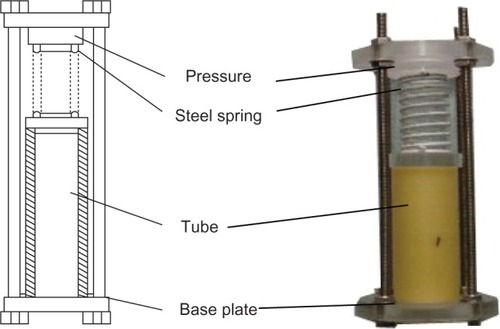
where W0 was the weight of the membrane tubes before degradation, and Wt the weight after degradation.
Results
Mechanical strength
The elastic modulus of n-CDHA-MAC straight membrane tubes was 3.5 ± 1.8 GPa, while goats’ femur was 3.66 ± 0.7 GPa. The compressive and bending strengths of straight membrane tubes were 66.4 ± 10.2 MPa and 840.7 ± 12.1 MPa, which were lower than the strength of goat femoral bone (69.0 ± 5.5 MPa and 900.2 ± 17.3 MPa), while the differences were not statistically significant between the two groups (P > 0.05). Therefore, the straight membrane tube had comparable mechanical strength with goat femur (). Further, in mechanical experimentation, the n-CDHA-MAC tube underwent deformation with local cracks but no occurrence of chipping, indicating that the membrane tube had high viscoelastic characteristics. Mechanical test curves of the two groups are shown in , , , and .
Table 2 Results of biomechanical experiments of the two groups
In vitro degradability experiments
During the soaking period, the PBS gradually became cloudy, and yellow flocculent precipitate appeared at the bottom. The membrane tubes showed no deformation, shape damage, or cracks. Before degradation, the surface and inside of the tube were observed by SEM (×1,000 magnification). The surface of the membrane tubes had knife marks and uplift stripes when the materials were processed; however, no collapse or holes were observed. As and show, the knife marks and uplift stripes of the membrane tubes gradually flattened without cracking or breaking throughout the degradation process. Compared to the control group before tube degradation, knife marks and uplift stripes flattened gradually, and were still visible after 3 months’ degradation. The surface of the nonmechanical loaded group also showed a flattened trend, but the change in the experimental group was more obvious, and the knife marks disappeared after 3 months’ degradation. Two experimental groups and the control group showed no significant changes in the internal cross-section by SEM (×1,000 magnification) and infrared analysis ( and ). They were not cracked or broken. Meanwhile, as shown in , in infrared analysis, the chemical groups of vibration peaks inside the membrane tubes did not change obviously during the degradation. In summary, with 3 months’ incubation in PBS, the membrane tube was degraded on the surface, and showed almost no change in internal structure.
Figure 6 (A–D) Surface morphology of 50 N-loaded tube group (×1,000 magnification): changes in membrane tube-surface morphology, uneven knife marks, and particles on the surface. Surface morphology of the membrane tubes before degradation (A), and after 1 (B), 2 (C), and 3 (D) months’ degradation. Knife marks and uplift stripes gradually became smooth, with no cracks or damage.
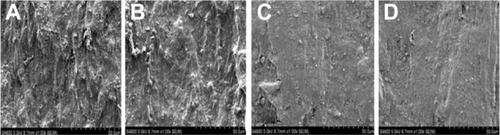
Figure 7 (A–D) Surface morphology of nonload group tube by scanning electron microscope (×1,000 magnification). Surface morphology of the membrane tubes before degradation (A), and after 1 (B), 2 (C), and 3 (D) months’ degradation. Knife marks and uplift stripes gradually became smooth, with no cracks or damage, but the degree of degradation was lower than the 50 N-loaded group.
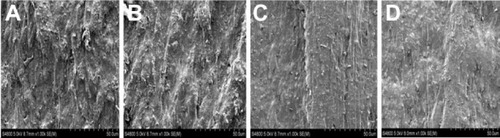
Figure 8 Scanning electron microscopy (×1,000 magnification) before degradation (A), and after 1 (B), 2 (C), and 3 (D) months’ degradation. The tubes’ internal cross-section had no substantial morphological changes during the degradation of 1, 2, and 3 months.
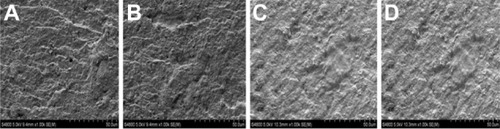
Figure 9 Fourier-transform infrared spectra of membrane tube before and after soaking. The membrane tubes in the phosphate-buffered saline degradation were removed to calculate the weight loss (%) after 1, 2, and 3 months. During the 3-month degradation, the weights of the membrane tubes had decreased, while the two loaded groups decreased significantly more quickly than the nonloaded group (P = 0.02). Compared to the 40 N-loaded group, the 50 N-loaded group of membrane tubes lost much more, but this was not statistically different (P = 0.92). Weight loss after 3 months’ incubation in phosphate-buffered saline is shown in . All groups showed rapid weight loss in 1 month, and then decreased slowly. The weight loss of the 50 N-loaded group was 3.27%, while the nonloaded group was lowest, with an average of 2.90%. In all observation periods, the weight loss of the 40 N- and 50 N-loaded groups was significantly different from the nonloaded group (P = 0.02).
Abbreviations: CN, carbon-nitrogen; NH, nitrogen-hydrogen; CH, carbon hydrogen; O=H, hydroxyl; N, loading force.
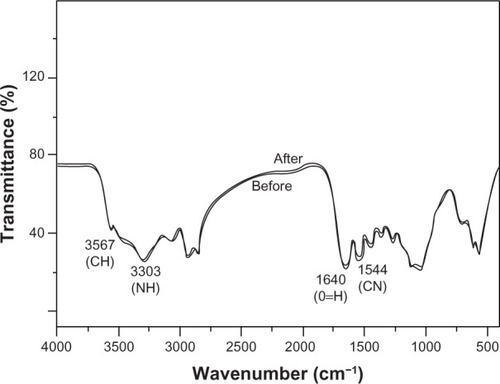
During the 3-month degradation, the compressive strength of tubes was decreased. The 40 N- and 50 N-loaded groups’ strength decreased more significantly than the nonloaded group’s (67.2%, 69.3%, and 57.0%, respectively). The two loaded groups compared with the nonloaded group strength decreases were statistically significant (P = 0.03). The mechanical strength of the 50 N group decreased more obviously than the 40 N-loaded group after 3 months’ degradation, but there were no statistically significant differences between the two groups (P = 0.87). Meanwhile, the bending strength of the three groups was reduced, but slightly less than the compressive strength decrease. Flexural strength of 50 N group was lowered to 29.5%, the 40 N group to 29.7%, and the non-load group to only 25.3%. There were statistically significant differences between the 40 N-/50 N-loaded groups and the nonloaded group (P = 0.02). The 40 N and 50 N groups showed no significant differences (P = 0.68). After 3 months’ degradation, the compressive strength of the tube was lower than the bending strength. The 50 N-loaded group’s compressive strength decreased quickly (69.3%). However, it was also possible to achieve compressive strength of 20.4 ± 1.2 MPa, which was greater than the maximum mechanical strength of 4.338 MPa based on goat femoral middle stationary state under three-dimensional finite-element analysis. After 3 months’ degradation, the mechanical strength of the membrane tube still met the goat femoral stationary state loading requirements.
Discussion
GBR is already a well-established therapy to repair mandible and alveolar bone defects in periodontal diseases.Citation11–Citation13 However, GBR technology is not mature enough in the orthopedic setting, especially for critical size defects of long bones.Citation14,Citation15 This is most likely attributable to the lower vascularity and more complicated mechanical environment of long-bone defects compared to mandible defects, and most of the bioabsorbable or nonresorbable membranes used in GBR are flexible and lack mechanical stability. The ideal membrane may require improved mechanical stability to maintain the space and protect the healing process in the case of long-bone defects.
Table 3 Weight-loss rates of the different groups after incubation in phosphate-buffered saline for different periods (%)
Table 4 Biomechanical results of compressive strength and bending strength before and after 3 months’ soaking in PBS ( + SD MPa)
The novel n-CDHA-MAC combined membrane tube we designed in this research has superior mechanical stability, and the n-CDHA:MAC ratio of this membrane tube was 3:7. In previous research,Citation9 it was found that the compressive strength of this n-CDHA-MAC composite was about 127 MPa, which is similar to that of natural cortical bones of humans.Citation16 In our study, the n-CDHA-MAC composites were processed into a tubular shape, thus preserving the continuity of the diaphysis for the repair of large diaphyseal bone defects. The compressive strength and bending intension of n-CDHA-MAC membrane tubes were 66.4 ± 10.2 MPa and 840.7 ± 12.1 MPa, which is close to that of femur cortical bones of goats (69.0 ± 5.5 MPa and 900.2 ± 17.3 MPa), and there was no statistically significant difference between them. It was verified that the n-CDHA-MAC membrane tubes had equivalent mechanical strength with goat cortex, which meets the deficiency of insufficient mechanical strength of most membranes.
In the research of Yang et al,Citation19 poly(lactide-co-glycolic acid) membrane scaffolds under dynamic mechanical load disintegrated after degradation for only 6 weeks, which could not meet the requirement of bone regeneration in long bones. After 3 months’ degradation, the mechanical properties of n-CDHA-MAC membrane tubes decreased significantly, and compressive strength changed more than bending strength in each group. The compressive strength of the 50 N-loaded group decreased the most (69.3%); however, it still maintained 20.4 MPa, which was greater than the maximum mechanical strength (4.338 MPa) of goat femoral diaphysis obtained by the three-dimensional finite-element analysis when the goat was in a standing situation. This indicates that the mechanical properties of n-CDHA-MAC membrane tubes after degradation can still meet the requirements of the strength for goats standing up. We can assume that the n-CDHA-MAC membrane tubes can maintain relatively safe mechanical properties to provide effective mechanical protection for the formation of new bone, and that they are beneficial for rebuilding of new bone.
In previous research,Citation9 the n-CDHA-MAC composites were examined by MTT (3-[4,5-dimethylthiazol-2yl]-2,5- diphenyl-2H-tetrazolium-bromide) assay and SEM observation. MG-63 cells were well adhered and spread on the composite surface, and they had a superior alkaline phosphatase activity. The viability and differentiation of MG-63 cells increased with time, indicating that the composite had no negative effects on MG-63 cells. Lee et al indicated that electrospun fibers immobilized with bone-forming peptide 1 derived from bone morphogenetic protein 7 may be a promising tool to develop clinically applicable bioactive material for GBR in craniomaxillofacial surgeryCitation12; however, with a lack of mechanical stability to maintain the space and protect the healing process in the case of long-segment femoral defects. Therefore, the n-CDHA-MAC membrane tube has not only good cytocompatibility but also superior mechanical stability. It is a promising candidate for GBR membranes.
As a novel bioabsorbable material, it is important to understand the degradation behavior of the n-CDHA-MAC membrane tube. Besides the properties of bioabsorbable materials, it has been proven that in vivo degradation would not only be affected by the chemical or biochemical condition but also by the mechanical loading.Citation17,Citation18 Biomaterials under mechanical load showed a more rapid degradation rate compared with biomaterials under static conditions. Consequently, it is necessary to investigate the degradation behavior of n-CDHA-MAC membrane tubes under mechanical loading. The compressive strength is the most common load when the goat is standing. We chose a specially designed device to provide 40 N or 50 N compressive loads, which is approximately one-quarter the weight of a goat.
The rate of load-induced degradation of n-CDHA-MAC membrane tubes was faster than that of unloaded ones. The mechanical loads greatly accelerated the degradation process of n-CDHA-MAC membrane tubes. After 3 months’ degradation in PBS, all membrane tubes experienced weight loss, especially in the first degradation month. The 50 N-loaded n-CDHA-MAC membrane tubes degraded faster than the 40 N-loaded ones, and n-CDHA-MAC membrane tubes without load exhibited least degradation. The results indicated that the mechanical degradation mechanism played an important role in the degradation process. According to Fan et al,Citation18 the mechanical load could change the length or angle of the covalent bonds, which could further affect the stability of the bonds of the polymer. All of the favored chain-segment motion leading to rapid hydrolytic degradation, which makes water molecules diffuse more easily into the amorphous chain segment and thus speed up the degradation of the scaffold. In the n-CDHA-MAC composite, the polymer first degraded, and then the nanoparticles could be dissolved. Even the 50 N-loaded group had faster mass loss compared to the other groups, but the total mass-loss rate was less than 3.5%. In earlier experiments,Citation9 2 × 12 mm-diameter n-CDHA-MAC composite weight loss reached 41.5%. We thought that the difference was attributable to the specifications of this membrane tube. This membrane had a tubular shape, and the wall thickness was 3 mm, which decreased the contact area with the PBS. The degradation of those membrane tubes occurred at the surface, which was proved by SEM and infrared analysis. The surface morphology had fewer particles and was smoother after 3 months’ degradation; however, its internal morphology did not change through SEM observation, and the chemical groups of vibration peaks inside membrane tubes did not change obviously during the degradation.
We can assume that the n-CDHA-MAC membrane tubes can maintain relatively safe mechanical properties to provide effective mechanical protection for the formation of new bone, and that they are beneficial for rebuilding of the new bone. It was verified that the n-CDHA-MAC membrane tubes have excellent mechanical properties, which solves the problem of insufficient mechanical strength of most GBR membranes.
Currently, synthetic biodegradable polymer material has become a research focus in the field of membrane-tube materials. The n-HA-MACP used in this study was polymerized by a variety of amino acid material with the natural inorganic material HA. Both sources are widely accessible; the polymerization process is simple, easy to control and shape, and can be used to make various shapes. The nanostructures constituted by hydroxyapatite crystals and collagen are very similar to natural bone tissue. Degradation occurs mainly in the surface of the composite; mechanical strength is reduced more slowly during degradation. These advantages make it an ideal material for the production of new membrane tubes for GBR. The mechanical loading methods and experimental methods can be used as an experimental model for the in vitro study of different materials.
Only the membrane-tube axial stress load and three-point bending stress test were carried on the membrane tubes in the study, and membrane degradation observed. This in vitro strength-modeling test is different to complex stress by muscle strain and joint activities when the membrane tubes are implanted in the body. In addition, the repair of large-bone defects needs 3–8 months. The degradation experiment continued for only 3 months; this is another shortcoming of the experiment. More in-depth and meticulous research remains to be carried out for the n-CDHA-MAC membrane tube. Also, further animal experiments should be conducted to prove these novel n-CDHA-MAC membrane tubes are effective in vivo.
Conclusion
In this study, a novel n-CDHA-MAC membrane tube was fabricated for GBR. It had excellent initial mechanical properties equivalent with the cortical bone of goat femur. Even after 3 months’ degradation in PBS under mechanical load, it maintained relatively safe mechanical strength, which is beneficial for new bone growth and rebuilding. This membrane tube is an ideal GBR membrane for critical size defects of long bones in in vivo experiments.
Acknowledgments
The work described in this paper was supported by the National Natural Science Foundation of China (31070834) and the National Science and Technology Support Program of China (2007BAE131304).
Disclosure
The authors report no conflicts of interest in this work.
References
- DonosNKostopoulosLTonettiMKarringTLong-term stability of autogenous bone grafts following combined application with guided bone regenerationClin Oral Implants Res200516213313915777321
- GiardinoRAldiniNNFiniMGiavaresiGTorricelliPEnhanced guided bone regeneration with a resorbable chamber containing demineralized bone matrixJ Trauma200252593393711988662
- BuserDDulaKBelserUHirtHPBertholdHLocalized ridge augmentation using guided bone regeneration. 1. Surgical procedure in the maxillaInt J Periodontics Restorative Dent199313129458330945
- TaguchiYAmizukaNNakadateMA histological evaluation for guided bone regeneration induced by a collagenous membraneBiomaterials200526316158616615885767
- FanYXiuKDuanHZhangMBiomechanical and histological evaluation of the application of biodegradable poly-L-lactic cushion to the plate internal fixation for bone fracture healingClin Biomech (Bristol, Avon)200823S7S16
- PinheiroALMartinez GerbiMEde Assis LimeiraFJrBone repair following bone grafting hydroxyapatite guided bone regeneration and infrared laser photobiomodulation: a histological study in a rodent modelLasers Med Sci200924223424018418643
- FangTDNacamuliRPSongHJFongKDShiYYLongakerMTGuided tissue regeneration enhances bone formation in a rat model of failed osteogenesisPlast Reconstr Surg200611741177118516582784
- ChenFMZhangJZhangMAnYChenFWuZFA review on endogenous regenerative technology in periodontal regenerative medicineBiomaterials20103317892792720684986
- LiHGongMYangAMaJLiXYanYDegradable biocomposite of nano calcium-deficient hydroxyapatite-multi(amino acid) copolymerInt J Nanomedicine201271287129522457591
- YosibashZPadanRJoskowiczLMilgromCA CT-based high-order finite element analysis of the human proximal femur compared to in-vitro experimentsJ Biomech Eng2007129329730917536896
- NymanSLindheJGottlowJNew attachment following surgical treatment of human periodontal diseaseJ Clin Periodontol1982942902966964676
- LeeYJLeeJHChoHJKimHKYoonTRShinHElectrospun fibers immobilized with bone forming peptide-1 derived from BMP7 for guided bone regenerationBiomaterials201334215059506923578562
- ClementiniMMorlupiAAgrestiniCBarlattaniAImmediate versus delayed positioning of dental implants in guided bone regeneration or onlay graft regenerated areas: a systematic reviewInt J Oral Maxillofac Surg201342564365023481543
- YangHLiKLiuYLiuZMiyoshiHPoly (D, L-lactide-co-glycolide) nanoparticles encapsulated fluorescent isothiocyanate and paclitaxol:preparation, release kinetics and anticancer effectJ Nanosci Nanotechnol20099128228719441308
- SommerladSMackenzieDJohanssonCAtwellRGuided bone augmentation around a titanium bone-anchored hearing aid implant in canine calvarium:an initial comparison of two barrier membranesClin Implant Dent Relat Res200791223317362494
- VerheyenCCde WijnJRvan BlitterswijkCAde GrootKEvaluation of hydroxyapatite/poly(L-lactide) composites: mechanical behaviorJ Biomed Mater Res19922610127712961331112
- BoehringerIKChemikalienAEAccelerated degradation test on resorbable polymersPlankHDaunerMRenardyMDegradation Phenomena on Polymeric BiomaterialsBerlinSpringer19926789
- FanYBLiPZengLHuangXJEffects of mechanical load on degradation of poly(D,L-lactic acid) foamPolym Degrad Stab2008933677683
- YangYFTangGWHongZhang HControlled release of BSA by microsphere-incorporated PLGA scaffolds under cyclic loadingMater Sci Eng C Mater Biol Appl2011312350356