Abstract
Improved understanding of interactions between nanoparticles and biological systems is needed to develop safety standards and to design new generations of nanomaterials. This article reviews the molecular mechanisms of cellular uptake of engineered nanoparticles, their intracellular fate, and their distribution within an organism. We have reviewed the available literature on the uptake and disposition of engineered nanoparticles. Special emphasis was placed on the analysis of experimental systems and their limitations with respect to their usefulness to predict the in vivo situation. The available literature confirms the need to study particle characteristics in an environment that simulates the situation encountered in biological systems. Phenomena such as protein binding and opsonization are of prime importance since they may have a strong impact on cellular internalization, biodistribution, and immunogenicity of nanoparticles in vitro and in vivo. Extrapolation from in vitro results to the in vivo situation in the whole organism remains a challenge. However, improved understanding of physicochemical properties of engineered nanoparticles and their influence on biological systems facilitates the design of nanomaterials that are safe, well tolerated, and suitable for diagnostic or therapeutic use in humans.
Introduction
Engineered nanomaterials (ENMs) are defined as materials composed of particles in an unbound state, or as an aggregate or agglomerate with one or more external dimensions in the size range from 1 nm to 100 nm.Citation1 Since active cellular uptake and tissue translocation of ENMs have been described for particles larger than 100 nm,Citation2,Citation3 we included literature reports on ENMs up to a size of 300 nm. There are many examples of clinical uses of ENMs. The majority of ENMs used as therapeutics on the market and in late clinical studies have diameters above 100 nm.Citation4 Small particles with a size of less than 2 nm show passive uptake into erythrocytes.Citation27 However, uptake mechanisms of such very small particles will not be discussed in this review. Due to their small size, ENMs have unique properties (ie, optical, thermal, catalytic, and biological) compared to larger particles.Citation5,Citation6 During the last two decades, ENMs with tailored physicochemical properties have emerged in different fields of our daily life. They are used for a variety of applications, such as color pigments, solar cells, and waste water treatment. Furthermore, ENMs are found in consumer products that may be in contact with the human organism, eg, food packaging, shampoos, sunscreens, and toothpastes. Thus, regulatory agencies are faced with new materials for which no nano-specific safety standards have been established. Moreover, products containing ENMs are often not declared since formal requirements are lacking.Citation5 The ingredients of ENMs tend to be listed as chemicals or micronized substances, and information about the ENMs’ content in the product may be missing. Little is known on how ENMs interact with the environment, including animals and human beings.Citation7 When used in a physiological environment, ENMs are faced with biological fluids, phospholipid membranes, clearing mechanisms, and harsh intracellular conditions. Due to their distinct physicochemical properties, ENMs interact in a different way with living cells as compared to dissolved molecules. It is a challenge to predict the mechanism of uptake in relation to one specific physicochemical property. highlights the differences between ENMs and small molecules with regard to their physical and chemical properties, cellular uptake mechanisms, intracellular fate, and toxic effects.
Figure 1 Interactions of cells with small molecules and nanoparticles.
Notes: Schematic representation of a eukaryotic cell and its interaction with nanoparticles (left part of picture) and small molecules (right part of picture). Interactions with nanoparticles are preceded by active cellular uptake leading to intracellular accumulation. Acute effects induced by small molecules are a consequence of both active and passive cell membrane permeation. Endocytosis leads to uptake of particles into endosomes (EN) and lysosomes (LY), followed by lysosomal degradation. Endosomal escape may lead to accumulation of particles in the cytoplasm or within mitochondria (Mito).
Abbreviations: EN, endosomes; LY, lysosomes; Mito, mitochondria; P-gp, P-glycoprotein.
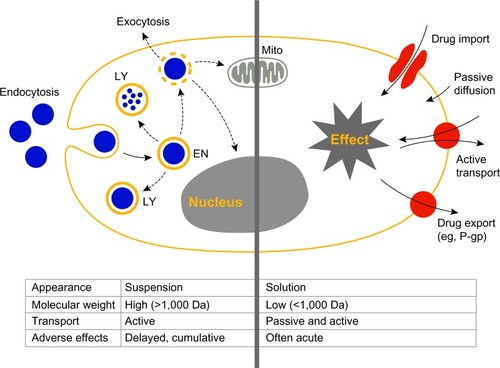
Small molecules are defined as compounds with a molecular weight of less than 1,000 Da. It is generally believed that lipophilic molecules below this threshold are able to penetrate cell membranes by passive diffusion. They have the potential to be taken up actively as well as passively by cells and to overcome cellular barriers within the body including the blood–brain barrier.Citation8,Citation9 In contrast, ENMs and macromolecules are mostly unable to diffuse passively into a living cell. They are colloidally dispersed and therefore require an active transport process for their uptake by target cells.Citation10,Citation11 Furthermore, ENMs are characterized by a high surface area to volume ratio as well as different geometries and surface characteristics. Particles of the same material can differ in shape, size, and porosity; whereas a molecule is a well-defined system.Citation12 The state of dispersion and the variable size and shape of ENMs induces different uptake mechanisms for the same material.
The present review focuses on interactions of ENMs with biological systems on a cellular level (ie, mechanisms of cellular uptake and intracellular accumulation) and on the level of the whole organism (ie, circulation, distribution, and elimination). These interactions are a function of the intrinsic physicochemical properties of ENMs. An additional factor is protein binding. Protein adsorption onto the surface of an ENM leads to the formation of a protein corona and changes properties such as size or surface charge dramatically.Citation13–Citation15 We reviewed published experimental procedures since the handling of ENMs is often a challenge, leading to statistical variability and artifacts.
General considerations
The state of dispersion of ENMs depends on their surface properties and the medium composition. Uptake studies should be performed with nonagglomerated ENMs. Agglomeration occurs by material interaction (ie, association of ENMs into clusters) or material-protein interaction. The resulting agglomerates sediment according to Stoke’s law. The rate of agglomeration should be studied prior to in vitro uptake studies in the correspondent medium, for example, with dynamic light scattering (DLS). However, when complex biological media are involved (as in vivo), DLS is unsuited for studying agglomeration kinetics since blood components may interfere, fluorescence single particle-tracking (fSPT) may be an alternative method to be used under these conditions.Citation16
Since the majority of ENM uptake studies use fluorescence as a tracking signal, it is crucial to minimize dye leakage from the ENM.Citation10 Approaches to test dye leakage are centrifugation, sodiumdodecyl sulfate polyacrylamide gel electrophoresis (PAGE), or fluorescence correlation spectroscopy (FCS).Citation17–Citation19 Special care should be taken when choosing an appropriate dye since lipophilic dyes may partition from the particulate structure into the lipid membranes of the cell. To circumvent dye leakage, intrinsic fluorescence of nanoparticles is an attractive alternative, as has been described for gold.Citation20,Citation21
To study the entry route of a specific ENM, physical and pharmacological blockers may be used. All methods used to induce a physical or pharmacological block of uptake pathways have certain limitations. Most pharmacological inhibitors are not specific and may influence alternative internalization routes and the actin cytoskeleton.Citation22 Pharmacological inhibitors interfere with vital cell functions and are therefore intrinsically toxic. Experiments have to be designed to prevent toxic effects, such as the complete disruption of all actin-dependent processes by actin-interfering agents. Moreover, compensatory routes of uptake may be activated in target cells upon inhibition of specific transport pathways. Finally, it should be taken into account that different cell lines have different uptake strategies. This impedes extrapolation of experimental results from one cell line to another. A recent review by Iversen et al summarizes the pharmacological blocking strategies and highlights the pitfalls of these reagents.Citation23 It should be mentioned that genetically modified cells offer an interesting alternative to pharmacological intervention.Citation24
The influence of plasma protein binding on opsonization
The properties of ENMs have the potential to modulate biological interactions between particles and target cells by different molecular mechanisms.Citation6,Citation25 Adsorption of biomolecules to surfaces influences the interactions at the nano-bio interface.Citation26–Citation28 In this way, bound proteins and biomolecules form a dynamic protein corona shaping the biological identity of the ENM. The composition of the protein corona varies over time due to continuous protein association and dissociation.Citation29,Citation30 Surface properties of ENMs will influence the composition of the protein corona. Hydrophobic ENMs easily adsorb proteins whereas hydrophilic ones are less prone to protein binding.Citation31 Therefore, hydrophobic ENMs agglomerate readily and interact with other hydrophobic residues of proteins or peptides (eg, blood or membrane proteins), thus promoting internalization.Citation30 Equally, positively charged ENMs adsorb different sets of proteins on their surface than negatively charged ones, elucidating distinct cellular interactions.Citation32 This influences the mode of cell entry, biodistribution, and biocompatibility of ENMs. Interestingly, ENMs with identical chemical composition but different size may attract different proteins, thereby giving rise to different biological identities.Citation33 An ENM in blood will have a different biological identity than the same ENM in body fluid and is therefore processed differently. The protein corona, in turn, modifies certain properties of the original ENM, such as surface charge and size. For example, highly positively charged nanoparticles with a positive zeta-potential may change their apparent charge to negative in cell-culture medium.Citation34 These factors should be carefully considered when using ENMs as drug delivery devices, as the targeting ability of functionalized ENMs may be shielded by adsorbed proteins.Citation35
Cellular uptake of nanoparticles
The phospholipid membrane of cells regulates the transport of molecules into the cells, thereby representing a universal barrier protecting fragile intracellular structures from extracellular materials (). To enter the cell, polar or charged biomolecules, such as amino acids, nucleosides, or glucose, require active transport systems involving proteins or ion channels. Many macromolecules are actively taken up by cells via endocytosis, which is a vesicular transport mechanism.Citation36,Citation37 Because ENMs may exist in the size range of proteins, eg, the hydrodynamic radius of an IgG antibody is close to 5 nm,Citation38 they are able to interact with the cellular machinery in a similar way to macromolecules.Citation10,Citation25,Citation26 Experimental studies revealed that most ENMs are actively incorporated into the cell via different endocytic pathways () comprising phagocytosis (“cell-eating”) and pinocytosis (“cell-drinking”). The latter pathway can be divided into clathrin-mediated endocytosis (CME), caveolae-mediated endocytosis (CvME), and alternative routes.Citation39,Citation40 The resulting transport vesicles differ with respect to the composition of the protein coat of the engulfed vesicle, size of the detached vesicle, and fate of the ingested cargo. ENMs have the potential to access cells by all entry portals depending on their size, shape, chemical composition, and surface modification. As stated above, it remains a challenge to correlate a given ENM property with an uptake route. In the following, we discuss the main cellular entry routes (ie, phagocytosis and pinocytosis) to establish a tentative link between nanoscale characteristics of ENMs and specific mechanisms of cellular uptake.
Figure 2 Known pathways of cellular uptake of nanoparticles.
Notes: Uptake of nanoparticles by eukaryotic cells is an active process. Endocytotic pathways include phagocytosis, and pinocytosis.
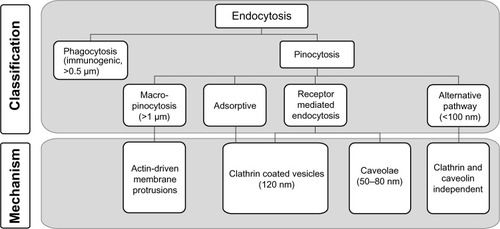
Phagocytosis
Phagocytosis is performed predominantly by specialized cells of the immune system (ie, macrophages, monocytes, neutrophils, and dendritic cells), to remove particles larger than 500 nm from the organism, mainly through the mode of a receptor-mediated process ().Citation41,Citation42 Particles are recognized by small proteins (opsonins) including immunoglobulins type G or M, complement fragments (C3, C5), or blood serum proteins such as fibronectin and laminin.Citation43 This process promotes the specific binding of protein-coated particles to internalizing receptors on the cell plasma membrane, ie, Fc receptors of the immunoglobulin superfamily or complement receptors.Citation41 Other receptors involved in the uptake of ENMs are the mannose/fucose receptorCitation44,Citation45 as well as the scavenger receptor,Citation46 where the involvement of the latter one may depend on the cell line used. The receptor-ligand interaction triggers a signal cascade in the target cell resulting in actin assembly and formation of a cell surface extension that zippers around the particle, engulfing it in an intracellular vesicle with a diameter of approximately 0.5 to 1 μm.Citation42 These vesicles, or phagosomes, mature by several fission and fusion events with late endosomes and lysosomes, resulting in the formation of phagolysosomes. Internalized particles are subsequently degraded, and the receptors are cycled back to the cell surface. The rate of these successive events depends greatly on the ingested particle and typically lasts from 30 minutes to several hours.Citation47 Although phagocytosis is generally thought to be involved in the uptake of larger particles, ie, particles sized >500 nm, recent reports document the phagocytic uptake of considerably smaller particles.Citation48–Citation50 The phagocytic uptake route seems to be rather unspecific since it depends on opsonization. ENMs taken up as agglomerate tend to be less easily degraded by the host as they can be detected in macrophages for several months, thus bearing a risk of long-term toxicity.Citation51,Citation52
Pinocytosis
Small particles ranging from a few nanometers to several hundred nanometers are generally taken up by pinocytosis (ie, fluid-phase uptake) occurring in almost all cell types (). There are four modes of pinocytosis, ie, macropinocytosis, adsorptive and receptor-mediated endocytosis, and alternative routes of uptake.Citation36 With macropinocytosis, large amounts of external fluid are taken up nonspecifically. ENMs located near the plasma membrane are thereby coincidentally internalized. During adsorptive pinocytosis, ENMs interact in a nonspecific manner with generic complementary binding sites on the cell surface. In contrast to macropinocytosis and adsorptive pinocytosis, RME is highly specific since it depends on the interaction between a ligand (eg, insulin or transferrin) and its complementary receptor on the surface of a target cell. RME is mediated predominantly either via the clathrin pathway or the caveolin pathway, depending on the specific nanoparticle-receptor interaction.Citation23,Citation53 Thus, several distinct pinocytic entry portals exist, depending on the type of interaction with the plasma membrane, size of incorporated vesicles, and type of proteins involved, eg, clathrin, caveolin, or receptors.Citation36 Thereby, the size of endocytic vesicles of approximately 60 nm (caveolae) and 120 nm (clathrin-coated vesicles) imposes limitations with respect to the maximum size of ENMs entering these pathways. Different mechanisms of pinocytic uptake into cells are discussed in more detail below.
Macropinocytosis
During macropinocytosis, ENMs with a size of >1 μm are taken up nonspecifically. This process is stimulated, for example, by growth factors interacting with receptor tyrosine kinases.Citation54 Activation of the signaling cascade results in the formation of actin-driven circular membrane protrusions that collapse onto the membrane and fuse with it. This generates uncoated endocytic vesicles with a size of 1 μm. These macropinosomes are of irregular shape and are handled by the endosomal/lysosomal route.Citation36 Macropinocytosis contributes to the internalization of larger ENMs, albeit in a rather unspecific manner and often in conjunction with other entry mechanism.Citation11,Citation55 The capacity of this uptake pathway for ENMs is very high, suggesting a possibility for pharmaceutical delivery.Citation56
Clathrin-mediated endocytosis
CME is considered to be the “classical” and best characterized route of cellular entry.Citation37 In polarized cells such as endothelial or epithelial cells, transport is initialized at the apical membrane domain.Citation36,Citation57 ENMs with a size of 120–150 nm are internalized within clathrin-coated vesicles entering the endosomal/lysosomal trafficking route.Citation58,Citation59 The upper size limit reported for particles entering the cell via this pathway is 200 nm.Citation55 CME is either adsorptiveCitation14 or receptor-mediated.Citation60 Cationic particles or proteins bind nonspecifically to the negatively charged cell surface.Citation61–Citation63 This triggers adsorptive CME. In contrast, the receptor-mediated process is highly selective and specific. Receptor ligands being internalized by this pathway include low-density lipoprotein (LDL), transferrin, growth factors, and insulin.Citation14
Caveolin-mediated endocytosis
CvME is the most prominent clathrin-independent uptake mechanism. This pathway is most pronounced in endothelial cells on the basolateral side, where it is an important uptake route for ENMs.Citation36,Citation64 The caveola is a small, flask-like structure, with a diameter of about 50–80 nm that is coated with caveolin-1.Citation65 These vesicles invaginate with the help of dynamin from hydrophobic membrane domains, which are rich in cholesterol and glycosphingolipids.Citation66 Caveolae are stable plasma membrane-associated structures.Citation67,Citation68 However, they can be induced to bud off by interaction with pathogens such as SV40 virus,Citation69 cholera toxin subunit B, or Shiga toxin.Citation70 With respect to ENMs, small particles seem to be transported more efficiently. For example, uptake of nanoparticles of 20 and 40 nm in size was demonstrated to be 5–10 times faster than that of nanoparticles sized 100 nm.Citation64,Citation71 Larger particles (>500 nm) appear to be taken up only in exceptional cases.Citation55 However, it is possible that ENM associating with the membrane may cover enough surface and colocalize by chance with certain markers like the caveolar marker. This does not mean that the uptake is actively dependent on caveolae.
The intracellular trafficking routes of caveolae are discussed controversially. While nonacidic, nondigestive pathways are favored, an additional link between caveolae and lysosomal routes for degradation cannot be excluded.Citation65,Citation70,Citation72 In addition, the so-called caveosomes (previously supposed to represent a special type of caveolar endosome) were shown to be an artifact created by overexpression of caveolin or caveolin mutants.Citation73 Pathogens escape from normal degradation in lysosomes and are directly transported to the Golgi and/or endoplasmic reticulum.Citation74 Thus, pathogens and ENMs can bypass lysosomal degradation.Citation75
Alternative routes of uptake
More recently, several endocytic routes that do not fit into the categories described above have emerged. Many of them are clathrin- and caveolin-independent. These routes depend on specific regulation by proteins such as Ras homolog family member A, ADP-ribosylation factor 6, or the cell division control protein 42 homolog, Cdc42.Citation37,Citation68 Although these mechanisms are still poorly understood, available data suggest that particles larger than 100 nm are internalized via these routes.Citation66
Characteristics of ENMs influencing cellular uptake
The size of ENMs is not the only criterion that predicts the mechanism of ENM uptake. Nonetheless, trends based on particle size exist and are summarized in . Nanoparticles with a diameter of 50 nm are more efficiently internalized by cells than smaller (about 15–30 nm) or larger (about 70–240 nm) particles.Citation58,Citation76 Nanoparticles with a diameter of 30–50 nm efficiently recruit and interact with membrane receptors and are subsequently taken up by receptor-mediated endocytosis.Citation77
Besides size, ENM shape is an important factor. Spherical ENMs are taken up much faster and more efficiently than rod-shaped ENMs, presumably due to the longer membrane wrapping time required for the longer rod-shaped particles.Citation78,Citation79 This notion is supported by in vivo experiments in rodents, where intravenous (IV)-injected elongated polymer micelles (filomicelles) remained in the circulation 10 times longer than spherical ENMs.Citation80 However, controversial findings were obtained using different materials. For example, Gratton et alCitation71 investigated hydrogel particle uptake into HeLa cells. The highest internalization rate was found for particles with an aspect ratio of three. ENMs seemed to use all internalization routes simultaneously.Citation72 Recent findings suggest that silica rods with an aspect ratio of 2.1 to 2.5 are taken up to a higher extent into HeLa cells than their spherical counterparts. In addition, uptake of rods with higher aspect ratios was marginal.Citation81 Tissue macrophages struggle to incorporate long and rigid fibers into phagosomes, thereby releasing harmful oxygen radicals and hydrolytic enzymes, causing chronic inflammation.Citation82 Similarly, the needle-like structure of carbon nanotubes may penetrate biological membranes, inflicting mechanical damage. These controversial results suggest that additional factors promote cellular uptake besides ENM size and shape.
Surface functionalities (eg, surface charge and functional groups) mediate interactions between ENMs and the cell surface. Positively charged particles interact strongly with the slightly anionic plasma membrane. They are taken up more readilyCitation83 or may disrupt plasma membrane integrity.Citation84 Positively charged ENMs are taken up via adsorptive mediated pinocytosis, whereas negatively charged ENMs use alternative uptake routes.Citation85 Nonionic particles tend not to interact with the cell membrane, as demonstrated for the polymer polyethylene glycol (PEG).Citation86 Nanoparticles can be functionalized with a plethora of ligands such as antibodies, peptides, or sugars. Their density, spatial distribution, and molecular weight plays an important role in determining the fate of ENMs in biological systems.Citation87 In addition, the chain length of chemical linkers like PEG, which are used to attach ligands to the surface of nanoparticles, may affect delivery to target cells.Citation88
Finally, specific cell types may interact with identical ENMs differently.Citation23,Citation89 It has been shown, for example, that macropinocytosis or phagocytosis is absent in brain capillary endothelial cells.Citation8 Red blood cells are known to be incapable of endocytosis. Furthermore, recent studies revealed an influence of the cell cycle phase on the cell’s capacity to take up ENMs.Citation90
Intracellular fate and endosomal escape
Once ENMs have been taken up by target cells, they are directed to the endosomal/lysosomal pathway in most instances. The intracellular vesicles either gradually mature (acidify) to late endosomes through multiple fission and fusion events, or they are recycled back to the cellular surface as trafficking endosomes ().Citation91 ENMs entrapped in late endosomes are likely to proceed to lysosomes where they are degraded. These compartments harbor proteases, hydrolases, and other enzymes promoting ENM degradation.Citation92 However, some ENMs (in particular positively charged, basic nanoparticles) are capable of escaping the endosome. This phenomenon has previously been described as the “proton sponge effect”.Citation25,Citation93 Osmotic swelling of the organelles leads to their rupture, as shown in the case of polyethylene imine.Citation93,Citation94 To implement drug delivery strategies, endosomal/lysosomal escape can be induced actively. pH-sensitive fusiogenic liposomes, for example, contain synthetic sterols and phospholipids, which undergo phase transition upon protonation at low pH. This results in the conversion of the hexagonal to lamellar structure of the liposomal membrane, thus disrupting the endosomal/lysosomal membrane.Citation95–Citation97 Similar effects can be induced using pH-sensitive fusiogenic peptides (eg, amphiphilic peptides with repetitive GALA sequences) in combination with cationic liposomes.Citation98 Other ENMs (eg, certain types of carbon nanotubes) penetrate the vesicle (or cell) membrane directly and enter the cytosol.Citation99 Once in the cytosol, ENMs may induce the production of reactive oxygen species and inflict oxidative stress.Citation100 In addition, potentially toxic interactions with other cellular organelles, such as mitochondria and the cell nucleus, may occur.Citation2 Effects that may be harmful for a healthy cell are desired in tumor cells, where an endosomal escape is needed to deliver a specific nanoparticulate drug to its intracellular compartment of action.
Methods to determine nanoparticle uptake
Widely used methods to study cellular uptake of ENMs are flow cytometry and microscopy. While flow cytometry allows for an efficient, fast, and quantitative assessment of particle uptake, microscopy provides qualitative insight into the subcellular localization of particles by analyzing small sample volumes. Flow cytometry as a quantitative approach to measure uptake cannot distinguish between externally attached and fully internalized ENM. Interaction with the cell surface can be studied experimentally if cellular uptake is inhibited. For example, endocytotic pathways can be blocked using pharmacological inhibitors.Citation22,Citation101 Alternatively, cells can be incubated at lowered temperatures to interrupt endocytic processing of particles. Temperatures around 20°C prevent progression of particles from early to late endosomes.Citation102 Further temperature lowering to 4°C, for example, blocks all energy-dependent processes.Citation103 Consequently, signals from fluorescent ENMs located at the surface of cells or within specific intracellular vesicles or organelles can, for example, be detected quantitatively by flow cytometry or qualitatively by confocal scanning laser microscopy.Citation104,Citation105 Intracellular localization of particles can be further confirmed using the quenching agent, trypan blue. This dye quenches signals from fluorescent dyes such as fluorescein isothiocyanate. Since trypan blue does not penetrate the membrane, only extracellular signals of free or surface-bound dye molecules are quenched.Citation106
Another quantitative approach is induced coupled plasma mass spectroscopy (ICP-MS). ICP-MS is a powerful tool for intracellular quantification of electron-dense materials and is a sensitive (ie, nanogram range) method for detecting elements with the exception of H, C, O, N, F, S, and inert gases. Due to this limitation, “soft” nanoparticles, such as liposomes, polymers, or dendrimers, are not detected. Additionally, ICP-MS is not able to distinguish between dissolved ions and nanoparticles. However, loading nanoparticles with a heavy metal may make the use of ICP-MS possible in such cases, as long as the physicochemical properties of the particles are not changed by the loading procedure.Citation107
Alternatively, confocal microscopy provides information on the three-dimensional structure of objects. Fluorescence colocalization studies give insight into intracellular trafficking after fixation of cells or by live-cell imaging. The latter technique relies on highly photostable fluorophores but avoids artifacts introduced by fixation reagents such as paraformaldehyde.Citation108 Subcellular fractionation can give additional insight into partitioning of ENMs inside the cell. Transmission electron microscopy is used to confirm subcellular particle localization with high resolution. This method allows quantitative assessments, but the procedure is time-consuming.Citation109,Citation110
It is advisable to use transmission electron microscopy in combination with energy-dispersive X-ray spectroscopy (EDX) to confirm the presence of nanoparticles.Citation111 Brandenberger et al studied intracellular particle distribution using quantum dots as reference material.Citation112 In this study, EDX was used to confirm the identity of quantum dots based on X-ray emission spectra of the elements Cd and S. This method seems to be particularly useful for identifying very small (5–10 nm) particles.Citation113
The biological fate of ENMs
In previous sections, we discussed interactions of ENMs with biological systems on a cellular level. This section focuses on in vivo kinetic properties of ENMs, addressing processes related to circulation, distribution, degradation, and excretion. There are different routes of administration including pulmonal,Citation114 dermal,Citation115,Citation116 oral,Citation117,Citation118 and IV routes.Citation119 However, this review will focus only on the IV route in healthy subjects. illustrates technical hurdles, challenges, and the different steps taken by ENMs before and during interaction with the living organism.
Figure 3 Experimental challenges and hurdles.
Notes: Specific physicochemical properties of ENMs may lead to technical challenges and artifacts in experimental systems. Particle agglomeration (A) reduces dosing accuracy or may lead to embolism after IV injection. Plasma-protein binding and opsonization of nanoparticles (B) may trigger a humoral immune response. Interaction of nanoparticles with cells of the MPS leads to accelerated plasma clearance (C). Accumulation of particles at a defined target site (D) might be impeded by their premature degradation and elimination (E).
Abbreviations: ENMs, engineered nanomaterials; IV, intravenous; MPS, mononuclear phagocytic system.
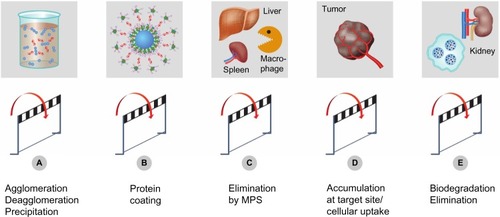
Circulation of ENMs
The state of dispersion in both the injected solution and the blood stream has to be defined, since agglomerates or precipitated material in the syringe may lead to dose variability (). Advanced pharmaceutical technologies are needed to stabilize nanosuspensions during storage and administration.Citation120 Size is an important parameter regarding circulation and distribution within the organism. In the blood stream, agglomerates may cause embolism with a potentially fatal outcome. Agglomerated particles have a tendency to accumulate after IV administration within the lung since venous blood is directed from the right heart ventricle to this organ. In vivo studies in the rat using polystyrene particles demonstrated passive accumulation in the lung for particles with a size exceeding a threshold of 10 μm.Citation121 Thus, findings of lung targeting of ENMs may be indicative of “accidental” trapping of agglomerates.Citation122 As mentioned above, phagocytosis of ENMs is an additional factor limiting prolonged circulation of particles bigger than 0.5 μm (). On the other hand, small ENMs (<5 nm to 10 nm) are cleared by kidney glomerular filtration.Citation123,Citation124
Opsonization and immunologic responses
When injected into the blood stream, foreign materials encounter different blood constituents such as red blood cells, white blood cells, platelets, and a variety of proteins. ENMs are known to interact with both proteinsCitation26 and cells.Citation125 Protein binding and opsonization are processes that change the surface properties of ENMs (). ENMs used for imaging or as a drug delivery system should be assessed with regard to their potential to cause hemolysis, thrombocyte aggregation, and complement activation.Citation47,Citation126 Hemolysis has been described for rigid materials such as silica nanoparticlesCitation127 or for soft nanoparticles such as liposomes.Citation128 Due to protein binding, these measurements should be done in presence of plasma proteins.Citation129
Protein coating of an ENM is a highly dynamic process, starting immediately upon IV injection.Citation130 The resulting protein corona is complex and varies depending on the size,Citation13 hydrophobicity/surface charge,Citation30 and shapeCitation131 of the particles. In the first instance, readily available proteins such as albumin are adsorbed onto the ENM surface, but may be replaced by other proteins (eg, lipoproteins or opsonins) over time, depending on the surface structure of the ENMs. Prediction of the extent of protein binding to ENM remains a challenge. Recent findings indicate that uncharged ENMs are opsonized more slowly than charged ENMs. The size of ENMs influences the binding of opsonins to spherical particles. Due to the higher curvature, smaller ENMs (<20 nm) will attract fewer opsonins than bigger ENMs (>200 nm). This explains why agglomerates are cleared by the mononuclear phagocytic system (MPS) more easily. The same is true for hydrophobic ENMs.Citation132 Recent studies revealed a strong shape dependency in relation with MPS recognition. Duan and LiCitation133 stated that an ellipsoidal ENM can be attacked by a macrophage in two different ways: when the macrophage attaches to the pointed end, the ENM will be internalized; however, when it attaches on the fat surface of the ellipsoid, it just spreads on the ENM and prolongs its circulation. This observation is in agreement with the findings of Arnida et al who found that gold nanorods were less recognized by the MPS compared to their spherical counterparts.Citation134
The question arises as to how ENMs can be designed to specifically adsorb certain proteins or avoid their adsorption. The best known strategy to diminish protein adsorption is by masking the particle surface with PEG. This hydrophilic, biocompatible, and nontoxic polymer is used to minimize interactions of macromolecules, eg, cytokinesCitation135 and nanoparticles, ie, liposomal carriersCitation101 with phagocytic cells of the immune system. The protective properties of PEG as a function of PEG chain length and PEG surface density were reviewed by Li and Huang.Citation136 Nevertheless, PEG is not able to fully prevent protein adsorption or opsonization. For example, for 5 nm gold nanoparticles, the protective effect of PEG diminishes within hours.Citation137 In addition, PEG may elicit an immunological response resulting in an accelerated blood clearance of ENMs. It has been observed that repeatedly injected PEGylated liposomal formulations are markedly less efficacious, probably because of anti-PEG antibody formation.Citation138 As an alternative to PEGylation, ENMs can be coated with amino acids such as lysine or cysteine. Such a mixed-charge monolayer-coating prevented protein adsorption in fetal bovine serum to 5 nm gold nanoparticles.Citation139 In summary, a hydrophilic coating (eg, PEG) and a neutral charge (expressed as the zeta potential in the correspondent medium) can minimize particle recognition by the immune system. Targeting strategies are often implemented using PEG-coated ENMs with surface ligands (such as folic acid, cell adhesion molecules, or transferrinCitation42) in order to enhance cellular uptake into target tissues and to avoid exposure of healthy cells. By this approach, it is crucial to maintain the stealth properties of the PEG, despite the bound ligands, to avoid interactions with cells of the immune system.Citation101
Distribution
ENMs are distributed throughout the body via the blood stream and extravasate this transport system according to their size. Extravasation of ENMs is restricted to specific tissues since the presence of tight junctions prevents ENMs larger than 2 nm to leave the circulation.Citation140 The fenestrations of blood vessels vary from organ to organ and can have different ranges in different species.Citation140 Moreover, the state of disease is changing the fenestration size,Citation141 with typical size for tumors ranging from 200–780 nm in mice.Citation142 ENMs with sizes ranging from 150–300 nm are mainly found in the liver and spleen, whereas smaller counterparts extravasate into the bone marrow. ENMs are cleared from the circulation in different organs. As depicted in , they are often trapped in the liver and spleen as these organs host the largest concentration of tissue resident macrophages (ie, MPS cells such as hepatic Kupffer cells). Glomerular filtration eliminates ENMs with a hydrodynamic diameter of <5 nm to 10 nm. Nonetheless, the relationship between the physicochemical properties and pharmacokinetic behavior of ENMs is poorly understood. Interpretation of experimental data is difficult since a plethora of different materials, excipients, and animal species are used. However, trends were identified for selected materials such as gold nanoparticles of different sizes and surface characteristics.Citation143,Citation144 Increased liver accumulation was found for particles with a size of ≥5 nm as compared to particles with a size of 1.4 nm. Particle size-dependent accumulation was found in no other organ than the liver. However, elevated levels of charged (positive and negative) gold nanoparticles were found in the spleen. In another study, PEG-coated gold nanoparticles with a size of 20–80 nm were investigated.Citation145 The length of the PEG chains was 2,000–5,000 Da. PEGylated gold nanoparticles were not recognized by macrophages. The PEG-5,000 coated gold particles with a size of 20 nm accumulated in solid tumors of experimental animals to an extent of 6.5% of the injected dose. Most of the remaining particles accumulated in other organs, mainly liver and spleen. Xie et al investigated the influence of the size of silica nanoparticles on their biodistribution.Citation146 After 30 days, they found silica particles trapped in the lungs, liver, and spleen and observed signs of liver injury. Compared to small silica particles (20 nm), larger particles (80 nm) were cleared faster from the body. A recent study investigated the difference in biodistribution of solid silica nanoparticles, mesoporous silica nanoparticles, and rod-shaped silica nanoparticles.Citation147 All particle types had a positively charged surface and showed extensive distribution to liver and spleen. Thus, most particles were recognized and eliminated by phagocytic macrophages. Porous particles with an aspect ratio of eight preferentially accumulated in the lung, whereas the nonporous particles were less prevalent in the lung. Amine modified silica nanoparticles reduced the affinity to lungs and kidneys. Silica nanoparticles were degraded and excreted via the hepatobiliary and renal routes. It has been reported that elongated fibers such as filomicellesCitation80 or gold nanorodsCitation134 display a longer circulation time since they are able to align with the blood flow. Compared to their spherical counterparts, rods are less preferentially taken up by macrophages, which reduce accumulation in MPS organs like the liver and spleen. Furthermore, gold nanorods had a higher accumulation in tumor tissue compared to spherical gold nanoparticles.Citation134
ENMs can be designed in a way to promote or avoid interactions with specific tissues or organs. The topic of active drug targeting was recently reviewed by Moghimi et al.Citation148 With such targeting strategies, ENMs can be designed and used for diagnostic imaging purposes or to deliver drugs to diseased tissues such as solid tumors (). The passive accumulation in the tumor is due to the enhanced permeability and retention effect, which is present in some tumors and dictates the maximum size for ENMs to extravasate into tumor tissue. This phenomenon is discussed in more detail by Jain.Citation149
Degradation and excretion
The term “metabolism” as defined in classical pharmacokinetics is not suitable for ENMs. In this section, the term “degradation” will be used instead to collectively cover multiple processes such as erosion, deagglomeration, disintegration, dissolution, or chemical degradation of particles. Available excretion and degradation studies solely included single administration of ENMs at a certain concentration. Accumulation effects after multiple dosing and bioaccumulation have only been studied in zebra fish.Citation150 Chemical reactivity and composition of the shell and core materials play an important role in degradation. ENMs known to “safely” degrade are porous silica nanoparticlesCitation20 and iron oxide particles.Citation151 Degradation of silica nanoparticles leads to the formation of silicic acid, which is excreted via feces and urine.Citation152 Due to the high specific surface area of the mesoporous material, hydrolysis of the silica network is a fast process.Citation153 Metal oxides including iron oxides are transformed by metallothionein that is abundantly expressed in liver and kidney.Citation154 Levy et al used two different methods to trace biodegradation of super paramagnetic iron oxide nanoparticles (SPIONs).Citation155 Upon degradation, these iron species lose their paramagnetic behavior and are transformed to ferritin. Thus, intact particles can be identified and traced due to their magnetic properties. However, degradation is a slow process. After two months, paramagnetic iron was still present in macrophages. Over prolonged time, the storage form of iron (ie, nonparamagnetic species) was more prevalent. The authors hypothesized that degradation of SPIONs took place in the acidic lysosomal compartment. It remains to be elucidated if these mechanisms of degradation might apply to other nanomaterials including other metal oxide materials with poor solubility. ENMs, like polymeric particles, have been shown to degrade by hydrolysis or enzymatic digestion in vitro.Citation156 Similar effects were observed in vivo.Citation157
Materials with poor solubility may remain in the organism over several weeks to months. When considering the use of a specific ENM as a drug delivery tool, its biodegradation and excretion pathways have to be known. Upon multiple dosing, ENMs may accumulate in MPS organs and cause severe damage. For example, Ye et al have studied long-term effects of quantum dots containing Cd-Se in rhesus monkeys.Citation158 In vivo, acute toxicity of theses nanoparticles was very low. However, chemical analysis after 90 days revealed that more than 90% of the injected Cd dose remained in the animals’ organs. In view of the limited availability of data, much more work needs to be done in the field of nanosafety.
Conclusion
ENMs have emerged in different fields of our daily life. However, their interaction with biological systems and their biological fate remain incompletely understood. It is therefore important to elucidate molecular mechanisms involved in cellular binding, uptake, and processing of ENMs. This knowledge is needed to design novel pharmaceutical applications for ENMs such as, for example, drug delivery and drug targeting strategies. By the same token, optimized ENM design may help to avoid unwanted interactions and toxicity, thereby making human use of novel materials possible.
The interaction of ENMs with complex biological systems, such as the human body, is still poorly understood. In vitro characterization of ENMs may help to obtain a mechanistic insight into their behavior in a biological environment (). This includes information on chemical properties and reactivity as well as particle dynamics in biological fluids. Studies have shown that protein binding may alter the properties of ENM surfaces influencing cellular binding and uptake. Interactions with the immune system depend on ENM size, geometry, and surface charge. However, this information cannot be easily extrapolated to any given cell line or even to another ENM with the same surface properties but different core materials. We propose to combine in vitro systems with ex vivo models such as lung models,Citation159 cell coculture systems, chicken egg models harboring xenografted tumors,Citation50 and placenta models.Citation3 These tools will be instrumental when designing nanomaterials with favorable pharmacokinetic properties and low intrinsic toxicity.Citation160 In any case, ENMs should be designed to be biocompatible and biodegradable to prevent their accumulation in the human body and limit their long-term toxic effects upon chronic exposure. Combining this knowledge about ENMs with smart drug delivery and drug targeting strategies will lead to innovative diagnostic and therapeutic applications.
Figure 4 Extrapolation from in vitro data to the in vivo situation.
Notes: In vitro experimental systems can be used to characterize nanoparticles with respect to their chemical composition and physicochemical properties. Cell-culture-based experimental systems can be used to study molecular mechanisms of cellular uptake and intracellular processing of particles. However, additional information is needed to address questions related to the in vivo behavior of nanomaterials and their interaction with complex biological systems. In particular, the prediction of pharmacokinetic parameters remains a challenge.
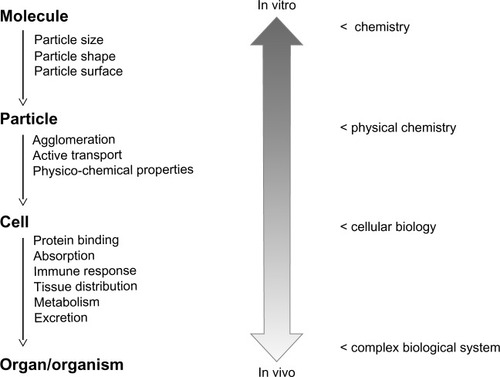
Acknowledgments
The present work was supported by grants from the “Freie Akademische Gesellschaft (FAG) Basel” and the Swiss Centre for Applied Human Toxicology (SCAHT). We thank Dr Silvia Rogers for editorial assistance.
Disclosure
The authors report no conflicts of interest in this work.
References
- BleekerEAde JongWHGeertsmaREConsiderations on the EU definition of a nanomaterial: science to support policy makingRegul Toxicol Pharmacol201365111912523200793
- Rothen-RutishauserBMühlfeldCBlankFMussoCGehrPTranslocation of particles and inflammatory responses after exposure to fine particles and nanoparticles in an epithelial airway modelPart Fibre Toxicol20074917894871
- WickPMalekAManserPBarrier capacity of human placenta for nanosized materialsEnviron Health Perspect2010118343243620064770
- ChangHIYehM-KClinical development of liposome-based drugs: formulation, characterization, and therapeutic efficacyInt J Nanomedicine20127496022275822
- KesslerREngineered nanoparticles in consumer products: understanding a new ingredientEnviron Health Perspect20111193a120a12521356630
- OberdörsterGMaynardADonaldsonKPrinciples for characterizing the potential human health effects from exposure to nanomaterials: elements of a screening strategyPart Fibre Toxicol20052816209704
- SomCWickPKrugHNowackBEnvironmental and health effects of nanomaterials in nanotextiles and façade coatingsEnviron Int20113761131114221397331
- PardridgeWMTransport of small molecules through the blood-brain barrier: biology and methodologyAdv Drug Deliv Rev1995151–3536
- PardridgeWMDrug transport across the blood-brain barrierJ Cereb Blood Flow Metab201232111959197222929442
- SalvatiAAbergCdos SantosTExperimental and theoretical comparison of intracellular import of polymeric nanoparticles and small molecules: toward models of uptake kineticsNanomedicine20117681882621453790
- ShaperoKFenaroliFLynchICottellDCSalvatiADawsonKATime and space resolved uptake study of silica nanoparticles by human cellsMol Biosyst20117237137820877915
- KrugHFWickPNanotoxicology: an interdisciplinary challengeAngew Chem Int Ed Engl20115061260127821290492
- JanschMStumpfPGrafCRühlEMüllerRHAdsorption kinetics of plasma proteins on ultrasmall superparamagnetic iron oxide (USPIO) nanoparticlesInt J Pharm20124281–212513322342465
- LesniakACampbellAMonopoliMPLynchISalvatiADawsonKASerum heat inactivation affects protein corona composition and nanoparticle uptakeBiomaterials201031369511951821059466
- MonopoliMPAbergCSalvatiADawsonKABiomolecular coronas provide the biological identity of nanosized materialsNat Nanotechnol201271277978623212421
- FilipeVPooleRKutscherMForierKBraeckmansKJiskootWFluorescence single particle tracking for the characterization of submicron protein aggregates in biological fluids and complex formulationsPharm Res20112851112112021298328
- MagdeDElsonEWebbWWThermodynamic Fluctuations in a Reacting System – Measurement by Fluorescence Correlation SpectroscopyPhys Rev Lett19722911705708
- OnacaOHughesDWBalasubramanianVGrzelakowskiMMeierWPalivanCGSOD antioxidant nanoreactors: influence of block copolymer composition on the nanoreactor efficiencyMacromol Biosci201010553153820112239
- OwHLarsonDRSrivastavaMBairdBAWebbWWWiesnerUBright and stable core-shell fluorescent silica nanoparticlesNano Lett20045111311715792423
- HeXNieHWangKTanWWuXZhangPIn vivo study of biodistribution and urinary excretion of surface-modified silica nanoparticlesAnal Chem200880249597960319007246
- LemelleAVekslerBKozhevnikovISAkchurinGGPiletskySAMeglinskiIApplication of gold nanoparticles as contrast agents in confocal laser scanning microscopyLaser Phys Lett2009617175
- IvanovAIPharmacological inhibition of endocytic pathways: is it specific enough to be useful?Methods Mol Biol2008440153318369934
- IversenTGSkotlandTSandvigKEndocytosis and intracellular transport of nanoparticles: Present knowledge and need for future studiesNano Today201162176185
- SmithPJGiroudMWigginsHLCellular entry of nanoparticles via serum sensitive clathrin-mediated endocytosis, and plasma membrane permeabilizationInt J Nanomedicine201272045205522619541
- NelAEMädlerLVelegolDUnderstanding biophysicochemical interactions at the nano-bio interfaceNat Mater20098754355719525947
- AggarwalPHallJBMcLelandCBDobrovolskaiaMAMcNeilSENanoparticle interaction with plasma proteins as it relates to particle biodistribution, biocompatibility and therapeutic efficacyAdv Drug Deliv Rev200961642843719376175
- WangTBaiJJiangXNienhausGUCellular uptake of nanoparticles by membrane penetration: a study combining confocal microscopy with FTIR spectroelectrochemistryACS Nano2012621251125922250809
- PrapainopKWitterDPWentworthPJrA chemical approach for cell-specific targeting of nanomaterials: small-molecule-initiated mis-folding of nanoparticle corona proteinsJ Am Chem Soc201213494100410322339401
- Darabi SahnehFScoglioCRiviereJDynamics of nanoparticle-protein corona complex formation: analytical results from population balance equationsPLoS ONE201385e6469023741371
- CedervallTLynchILindmanSUnderstanding the nanoparticle– protein corona using methods to quantify exchange rates and affinities of proteins for nanoparticlesProc Natl Acad Sci U S A200710472050205517267609
- EsmaeiliFGhahremaniMHEsmaeiliBKhoshayandMRAtyabiFDinarvandRPLGA nanoparticles of different surface properties: preparation and evaluation of their body distributionInt J Pharm20083491–224925517875373
- FleischerCCPayneCKNanoparticle surface charge mediates the cellular receptors used by protein-nanoparticle complexesJ Phys Chem B2012116308901890722774860
- ZhangHBurnumKELunaMLQuantitative proteomics analysis of adsorbed plasma proteins classifies nanoparticles with different surface properties and sizeProteomics201111234569457721956884
- LimbachLKWickPManserPGrassRNBruininkAStarkWJExposure of engineered nanoparticles to human lung epithelial cells: influence of chemical composition and catalytic activity on oxidative stressEnviron Sci Technol200741114158416317612205
- SalvatiAPitekASMonopoliMPTransferrin-functionalized nanoparticles lose their targeting capabilities when a biomolecule corona adsorbs on the surfaceNat Nanotechnol20138213714323334168
- ConnerSDSchmidSLRegulated portals of entry into the cellNature20034226927374412621426
- KumariSMgSMayorSEndocytosis unplugged: multiple ways to enter the cellCell Res201020325627520125123
- JøssangTFederJRosenqvistEPhoton correlation spectroscopy of human IgGJ Protein Chem1988721651713255367
- LühmannTRimannMBittermannAGHallHCellular uptake and intracellular pathways of PLL-g-PEG-DNA nanoparticlesBioconjug Chem20081991907191618717536
- MailänderVLandfesterKInteraction of nanoparticles with cellsBiomacromolecules20091092379240019637907
- AderemAUnderhillDMMechanisms of phagocytosis in macrophagesAnnu Rev Immunol19991759362310358769
- HillaireauHCouvreurPNanocarriers’ entry into the cell: relevance to drug deliveryCell Mol Life Sci200966172873289619499185
- OwensDE3rdPeppasNAOpsonization, biodistribution, and pharmacokinetics of polymeric nanoparticlesInt J Pharm200630719310216303268
- IracheJMSalmanHHGamazoCEspuelasSMannose-targeted systems for the delivery of therapeuticsExpert Opin Drug Deliv20085670372418532925
- PatelPCGiljohannDADanielWLZhengDPrigodichAEMirkinCAScavenger receptors mediate cellular uptake of polyvalent oligonucleotide-functionalized gold nanoparticlesBioconjug Chem201021122250225621070003
- WangHWuLReinhardBMScavenger receptor mediated endocytosis of silver nanoparticles into J774A.1 macrophages is heterogeneousACS Nano2012687122713222799499
- DobrovolskaiaMAMcNeilSEImmunological properties of engineered nanomaterialsNat Nanotechnol20072846947818654343
- FrançaAAggarwalPBarsovEVKozlovSVDobrovolskaiaMAGonzález-FernándezÁMacrophage scavenger receptor A mediates the uptake of gold colloids by macrophages in vitroNanomedicine (Lond)2011671175118821675859
- KrpetićZPortaFCanevaEDal SantoVScarìGPhagocytosis of biocompatible gold nanoparticlesLangmuir20102618147991480520795674
- LunovOSyrovetsTLoosCDifferential uptake of functionalized polystyrene nanoparticles by human macrophages and a monocytic cell lineACS Nano2011531657166921344890
- WangXXiaTNtimSADispersal state of multiwalled carbon nanotubes elicits profibrogenic cellular responses that correlate with fibrogenesis biomarkers and fibrosis in the murine lungACS Nano20115129772978722047207
- ZhuMTFengWYWangYParticokinetics and extrapulmonary translocation of intratracheally instilled ferric oxide nanoparticles in rats and the potential health risk assessmentToxicol Sci2009107234235119023088
- VercauterenDRejmanJMartensTFDemeesterJDe SmedtSCBraeckmansKOn the cellular processing of non-viral nanomedicines for nucleic acid delivery: mechanisms and methodsJ Control Release2012161256658122613879
- MercerJHeleniusAVirus entry by macropinocytosisNat Cell Biol200911551052019404330
- RejmanJOberleVZuhornISHoekstraDSize-dependent internalization of particles via the pathways of clathrin- and caveolae-mediated endocytosisBiochem J2004377Pt 115916914505488
- RimaWSanceyLAloyM-TInternalization pathways into cancer cells of gadolinium-based radiosensitizing nanoparticlesBiomaterials201334118119523046756
- SandvigKPustSSkotlandTvan DeursBClathrin-independent endocytosis: mechanisms and functionCurr Opin Cell Biol201123441342021466956
- ChithraniBDGhazaniAAChanWCWDetermining the size and shape dependence of gold nanoparticle uptake into mammalian cellsNano Lett20066466266816608261
- Harush-FrenkelORozenturEBenitaSAltschulerYSurface charge of nanoparticles determines their endocytic and transcytotic pathway in polarized MDCK cellsBiomacromolecules20089243544318189360
- BickelUYoshikawaTPardridgeWMDelivery of peptides and proteins through the blood-brain barrierAdv Drug Deliv Rev2001461–324727911259843
- HerveFGhineaNScherrmannJ-MCNS delivery via adsorptive transcytosisAAPS J200810345547218726697
- PredescuSAPredescuDNMalikABMolecular determinants of endothelial transcytosis and their role in endothelial permeabilityAm J Physiol Lung Cell Mol Physiol20072934L823L84217644753
- ThomsenLBLichotaJEskehaveTNBrain delivery systems via mechanism independent of receptor-mediated endocytosis and adsorptive-mediated endocytosisCurr Pharm Biotechnol201213122349235423016641
- WangZTiruppathiCMinshallRDMalikABSize and dynamics of caveolae studied using nanoparticles in living endothelial cellsACS Nano20093124110411619919048
- PartonRGSimonsKThe multiple faces of caveolaeNat Rev Mol Cell Biol20078318519417318224
- MayorSPaganoREPathways of clathrin-independent endocytosisNat Rev Mol Cell Biol20078860361217609668
- HommelgaardAMRoepstorffKVilhardtFTorgersenMLSandvigKvan DeursBCaveolae: stable membrane domains with a potential for internalizationTraffic20056972072416101676
- HowesMTKirkhamMRichesJClathrin-independent carriers form a high capacity endocytic sorting system at the leading edge of migrating cellsJ Cell Biol2010190467569120713605
- PelkmansLPüntenerDHeleniusALocal actin polymerization and dynamin recruitment in SV40-induced internalization of caveolaeScience2002296556753553911964480
- HayerAStoeberMRitzDEngelSMeyerHHHeleniusACaveolin-1 is ubiquitinated and targeted to intralumenal vesicles in endolysosomes for degradationJ Cell Biol2010191361562921041450
- GrattonSERoppPAPohlhausPDThe effect of particle design on cellular internalization pathwaysProc Natl Acad Sci U S A200810533116131161818697944
- PelkmansLBürliTZerialMHeleniusACaveolin-stabilized membrane domains as multifunctional transport and sorting devices in endocytic membrane trafficCell2004118676778015369675
- PartonRGHowesMTRevisiting caveolin trafficking: the end of the caveosomeJ Cell Biol2010191343944121041440
- BengaliZReaJCSheaLDGene expression and internalization following vector adsorption to immobilized proteins: dependence on protein identity and densityJ Gene Med20079866867817533618
- RejmanJConeseMHoekstraDGene transfer by means of lipo-and polyplexes: role of clathrin and caveolae-mediated endocytosisJ Liposome Res200616323724716952878
- LuFWuSHHungYMouCYSize effect on cell uptake in well-suspended, uniform mesoporous silica nanoparticlesSmall20095121408141319296554
- AlbaneseATangPSChanWCThe effect of nanoparticle size, shape, and surface chemistry on biological systemsAnnu Rev Biomed Eng20121411622524388
- ChampionJAKatareYKMitragotriSParticle shape: a new design parameter for micro- and nanoscale drug delivery carriersJ Control Release20071211–23917544538
- FerrariMNanogeometry: beyond drug deliveryNat Nanotechnol20083313113218654480
- GengYDalhaimerPCaiSShape effects of filaments versus spherical particles in flow and drug deliveryNat Nanotechnol20072424925518654271
- MengHYangSLiZAspect ratio determines the quantity of mesoporous silica nanoparticle uptake by a small GTPase-dependent macropinocytosis mechanismACS Nano2011564434444721563770
- DonaldsonKMurphyFADuffinRPolandCAAsbestos, carbon nanotubes and the pleural mesothelium: a review of the hypothesis regarding the role of long fibre retention in the parietal pleura, inflammation and mesotheliomaPart Fibre Toxicol20107520307263
- QiuYLiuYWangLSurface chemistry and aspect ratio mediated cellular uptake of Au nanorodsBiomaterials201031307606761920656344
- LovrićJBazziHSCuieYFortinGRAWinnikFMMaysingerDDifferences in subcellular distribution and toxicity of green and red emitting CdTe quantum dotsJ Mol Med200583537738515688234
- DausendJMusyanovychADassMUptake mechanism of oppositely charged fluorescent nanoparticles in HeLa cellsMacromol Biosci20088121135114318698581
- WoodleMCLasicDDSterically stabilized liposomesBiochim Biophys Acta1992111321711991510996
- ElsabahyMWooleyKLDesign of polymeric nanoparticles for biomedical delivery applicationsChem Soc Rev20124172545256122334259
- CruzLJTackenPJFokkinkRFigdorCGThe influence of PEG chain length and targeting moiety on antibody-mediated delivery of nanoparticle vaccines to human dendritic cellsBiomaterials201132286791680321724247
- Dos SantosTVarelaJLynchISalvatiADawsonKAQuantitative assessment of the comparative nanoparticle-uptake efficiency of a range of cell linesSmall20117233341334922009913
- KimJAÅbergCSalvatiADawsonKARole of cell cycle on the cellular uptake and dilution of nanoparticles in a cell populationNat Nanotechnol201271626822056728
- SaftigPKlumpermanJLysosome biogenesis and lysosomal membrane proteins: trafficking meets functionNat Rev Mol Cell Biol200910962363519672277
- FischerHCHauckTSGómez-AristizábalAChanWCExploring primary liver macrophages for studying quantum dot interactions with biological systemsAdv Mater201022232520252420491094
- BoussifOZantaMABehrJPOptimized galenics improve in vitro gene transfer with cationic molecules up to 1000-foldGene Ther1996312107410808986433
- MooreNMSheppardCLBarbourTRSakiyama-ElbertSEThe effect of endosomal escape peptides on in vitro gene delivery of polyethylene glycol-based vehiclesJ Gene Med200810101134114918642401
- CaraccioloGCaminitiRDigmanMAGrattonESanchezSEfficient escape from endosomes determines the superior efficiency of multicomponent lipoplexesJ Phys Chem B2009113154995499719301832
- ChuCJDijkstraJLaiMZHongKSzokaFCEfficiency of cytoplasmic delivery by pH-sensitive liposomes to cells in culturePharm Res1990788248342172955
- ZuhornISBakowskyUPolushkinENonbilayer phase of lipoplex-membrane mixture determines endosomal escape of genetic cargo and transfection efficiencyMol Ther200511580181015851018
- KobayashiSNakaseIKawabataNCytosolic targeting of macromolecules using a pH-dependent fusogenic peptide in combination with cationic liposomesBioconjug Chem200920595395919388672
- MuQBroughtonDLYanBEndosomal Leakage and Nuclear Translocation of Multiwalled Carbon Nanotubes: Developing a Model for Cell UptakeNano Lett20099124370437519902917
- AshaRaniPVLow Kah MunGHandeMPValiyaveettilSCytotoxicity and genotoxicity of silver nanoparticles in human cellsACS Nano20093227929019236062
- HuwylerJDreweJKrähenbuhlSTumor targeting using liposomal antineoplastic drugsInt J Nanomedicine200831212918488413
- PunnonenELRyhänenKMarjomäkiVSAt reduced temperature, endocytic membrane traffic is blocked in multivesicular carrier endosomes in rat cardiac myocytesEur J Cell Biol19987543443529628320
- OgrisMWagnerESteinleinPA versatile assay to study cellular uptake of gene transfer complexes by flow cytometryBiochim Biophys Acta20001474223724310742604
- LeclercLBoudardDPourchezJQuantification of microsized fluorescent particles phagocytosis to a better knowledge of toxicity mechanismsInhal Toxicol201022131091110021047166
- YangHLouCXuMWuCMiyoshiHLiuYInvestigation of folate-conjugated fluorescent silica nanoparticles for targeting delivery to folate receptor-positive tumors and their internalization mechanismInt J Nanomedicine201162023203221976977
- NuutilaJLiliusE-MFlow cytometric quantitative determination of ingestion by phagocytes needs the distinguishing of overlapping populations of binding and ingesting cellsCytometry A20056529310215825183
- DrescherDGiesenCTraubHPanneUKneippJJakubowskiNQuantitative imaging of gold and silver nanoparticles in single eukaryotic cells by laser ablation ICP-MSAnal Chem201284229684968823121624
- BacallaoRSohrabSPhillipsCGuiding Principles of Specimen Preservation for Confocal Fluorescence MicroscopyPawleyJBHandbook of Biological Confocal Microscopy3rd edMadison, WISpringer Science+Business Media2006368380
- ElsaesserABarnesCAMcKerrGQuantification of nanoparticle uptake by cells using an unbiased sampling method and electron microscopyNanomedicine (Lond)2011671189119821929457
- MühlfeldCMayhewTMGehrPRothen-RutishauserBA novel quantitative method for analyzing the distributions of nanoparticles between different tissue and intracellular compartmentsJ Aerosol Med200720439540718158712
- SchmutzHRDetampelPBühlerTBüttlerAGygaxBHuwylerJIn vitro assessment of the formation of ceftriaxone-calcium precipitates in human plasmaJ Pharm Sci201110062300231021254064
- BrandenbergerCCliftMJDVanheckeDIntracellular imaging of nanoparticles: is it an elemental mistake to believe what you see?Part Fibre Toxicol201071520525241
- MühlfeldCBrandenbergerCUptake of nanoparticles by cells: do you know their number?Nanomedicine (Lond)20116711491151 author reply 1153–115421929454
- ChoiHSAshitateYLeeJHRapid Translocation of Nanoparticles from the Lung Airspaces to the BodyNat Biotechnol201028121300130321057497
- ShimJSeok KangHParkWSHanSHKimJChangISTransdermal delivery of mixnoxidil with block copolymer nanoparticlesJ Control Release200497347748415212879
- AggarwalNGoindiSPreparation and in vivo evaluation of solid lipid nanoparticles of griseofulvin for dermal useJ Biomed Nanotechnol20139456457623621015
- Des RieuxAFievezVGarinotMSchneiderYJPréatVNanoparticles as potential oral delivery systems of proteins and vaccines: a mechanistic approachJ Control Release2006116112717050027
- XuQZhangNQinWLiuJJiaZLiuHPreparation, in vitro and in vivo evaluation of budesonide loaded core/shell nanofibers as oral colonic drug delivery systemJ Nanosci Nanotechnol201313114915623646710
- MagerDEModyVXuCPhysiologically based pharmacokinetic model for composite nanodevices: effect of charge and size on in vivo dispositionPharm Res20122992534254222688900
- Petri-FinkASteitzBFinkaASalaklangJHofmannHEffect of cell media on polymer coated superparamagnetic iron oxide nanoparticles (SPIONs): colloidal stability, cytotoxicity, and cellular uptake studiesEur J Pharm Biopharm200868112913717881203
- KutscherHLChaoPDeshmukhMThreshold size for optimal passive pulmonary targeting and retention of rigid microparticles in ratsJ Control Release20101431313720043961
- AnejaMKGeigerJPHimmelARudolphCTargeted gene delivery to the lungExpert Opin Drug Deliv20096656758319450167
- ChoiHSLiuWMisraPRenal clearance of quantum dotsNat Biotechnol200725101165117017891134
- MaedaHThe enhanced permeability and retention (EPR) effect in tumor vasculature: the key role of tumor-selective macromolecular drug targetingAdv Enzyme Regul20014118920711384745
- ShahNBVercellottiGMWhiteJGFeganAWagnerCRBischofJCBlood-Nanoparticle Interactions and in Vivo Biodistribution: Impact of Surface PEG and Ligand PropertiesMol Pharm Epub7232012
- DobrovolskaiaMAClogstonJDNeunBWHallJBPatriAKMcNeilSEMethod for analysis of nanoparticle hemolytic properties in vitroNano Lett2008882180218718605701
- YuTMaluginAGhandehariHImpact of silica nanoparticle design on cellular toxicity and hemolytic activityACS Nano2011575717572821630682
- QuirionFSt-PierreSReduction of the in vitro hemolytic activity of soybean lecithin liposomes by treatment with a block copolymerBiophys Chem19914021291341883947
- PaulaAJMartinezDSTAraujo JúniorRTSouza FilhoAGAlvesOLSuppression of the hemolytic effect of mesoporous silica nanoparticles after protein corona interaction: independence of the surface microchemical environmentJ Braz Chem Soc2012231018071814
- LynchIDawsonKAProtein-nanoparticle interactionsNano Today200831–24047
- GasserMRothen-RutishauserBKrugHFThe adsorption of biomolecules to multi-walled carbon nanotubes is influenced by both pulmonary surfactant lipids and surface chemistryJ Nanobiotechnology201083121159192
- GessnerAWaiczRLieskeAPaulkeBRMäderKMüllerRNanoparticles with decreasing surface hydrophobicities: influence on plasma protein adsorptionInt J Pharm2000196224524910699728
- DuanXLiYPhysicochemical characteristics of nanoparticles affect circulation, biodistribution, cellular internalization, and traffickingSmall201399–101521153223019091
- ArnidaJanát-AmsburyMMRayAPetersonCMGhandehariHGeometry and surface characteristics of gold nanoparticles influence their biodistribution and uptake by macrophagesEur J Pharm Biopharm201177341742321093587
- BailonPPalleroniASchafferCARational design of a potent, long-lasting form of interferon: a 40 kDa branched polyethylene glycol-conjugated interferon α-2a for the treatment of hepatitis CBioconjug Chem200112219520211312680
- LiSDHuangLStealth nanoparticles: high density but sheddable PEG is a key for tumor targetingJ Control Release2010145317818120338200
- LipkaJSemmler-BehnkeMSperlingRABiodistribution of PEG-modified gold nanoparticles following intratracheal instillation and intravenous injectionBiomaterials201031256574658120542560
- IshiharaTTakedaMSakamotoHAccelerated blood clearance phenomenon upon repeated injection of PEG-modified PLA-nanoparticlesPharm Res200926102270227919633820
- MurthyAKStoverRJHardinWGCharged gold nanoparticles with essentially zero serum protein adsorption in undiluted fetal bovine serumJ Am Chem Soc2013135217799780223565806
- GarnettMCKallinteriPNanomedicines and nanotoxicology: some physiological principlesOccup Med (Lond)200656530731116868128
- SvistounovDWarrenAMcNerneyGPThe Relationship between fenestrations, sieve plates and rafts in liver sinusoidal endothelial cellsPLoS ONE201279e4613423029409
- GaumetMVargasAGurnyRDelieFNanoparticles for drug delivery: The need for precision in reporting particle size parametersEur J Pharm Biopharm20086911917826969
- LiuXHuangNLiHJinQJiJSurface and size effects on cell interaction of gold nanoparticles with both phagocytic and nonphagocytic cellsLangmuir201329299138914823815604
- HirnSSemmler-BehnkeMSchlehCParticle size-dependent and surface charge-dependent biodistribution of gold nanoparticles after intravenous administrationEur J Pharm Biopharm201177340741621195759
- ZhangGYangZLuWInfluence of anchoring ligands and particle size on the colloidal stability and in vivo biodistribution of polyethylene glycol-coated gold nanoparticles in tumor-xenografted miceBiomaterials200930101928193619131103
- XieGSunJZhongGShiLZhangDBiodistribution and toxicity of intravenously administered silica nanoparticles in miceArch Toxicol201084318319019936708
- HuangXLiLLiuTThe shape effect of mesoporous silica nanoparticles on biodistribution, clearance, and biocompatibility in vivoACS Nano2011575390539921634407
- MoghimiSMHunterACAndresenTLFactors controlling nanoparticle pharmacokinetics: an integrated analysis and perspectiveAnnu Rev Pharmacol Toxicol20125248150322035254
- JainRKNormalizing tumor microenvironment to treat cancer: bench to bedside to biomarkersJ Clin Oncol201331172205221823669226
- WangJZhuXZhangXDisruption of zebrafish (Danio rerio) reproduction upon chronic exposure to TiO2 nanoparticlesChemosphere201183446146721239038
- MahmoudiMHofmannHRothen-RutishauserBPetri-FinkAAssessing the in vitro and in vivo toxicity of superparamagnetic iron oxide nanoparticlesChem Rev201211242323233822216932
- ParkJHGuLvon MaltzahnGRuoslahtiEBhatiaSNSailorMJBiodegradable luminescent porous silicon nanoparticles for in vivo applicationsNat Mater20098433133619234444
- ChenKZhangJGuHDissolution from inside: a unique degradation behaviour of core–shell magnetic mesoporous silica nanoparticles and the effect of polyethyleneimine coatingJ Mater Chem201222412200522012
- CoylePPhilcoxJCCareyLCRofeAMMetallothionein: the multipurpose proteinCell Mol Life Sci200259462764712022471
- LevyMLucianiNAlloyeauDLong term in vivo biotransformation of iron oxide nanoparticlesBiomaterials201132163988399921392823
- AkagiTHigashiMKanekoTKidaTAkashiMHydrolytic and enzymatic degradation of nanoparticles based on amphiphilic poly(gamma-glutamic acid)-graft-L-phenylalanine copolymersBiomacromolecules20067129730316398528
- ParkKKimJHNamYSEffect of polymer molecular weight on the tumor targeting characteristics of self-assembled glycol chitosan nanoparticlesJ Control Release2007122330531417643545
- YeLYongKTLiuLA pilot study in non-human primates shows no adverse response to intravenous injection of quantum dotsNat Nanotechnol20127745345822609691
- WilkinsonKEkstrand-HammarströmBAhlinderLVisualization of custom-tailored iron oxide nanoparticles chemistry, uptake, and toxicityNanoscale20124237383739323070150
- SomCNowackBKrugHFWickPToward the Development of Decision Supporting Tools That Can Be Used for Safe Production and Use of NanomaterialsAcc Chem Res Epub10312012