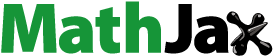
Abstract
A drug model photosensitizer–conjugated upconversion nanoparticles nanocomplex was explored for application in near-infrared photodynamic therapy. As near-infrared penetrates deeper into the tissue, the model is useful for the application of photodynamic therapy in deeper tissue. The nanocomplex that was synthesized had low polydispersity, and the upconversion nanoparticle was covalently conjugated with the photosensitizer. The robust bond could prevent the undesired premature release of photosensitizer and also enhance the singlet-oxygen generation. Singlet-oxygen generation rate from this nanocomplex was evaluated in solution. The photodynamic therapy effect was assessed with MCF-7 cells in two different methods, 3-(4,5-dimethylth-iazol-2-yl)-2,5-diphenyltetrazolium bromide (MTT) assay and live/dead assay. The assay results showed that promising efficacy (>90%) can be achieved with a low concentration (50 μg mL−1) of this nanocomplex and mild dosage (7 mW cm−2) of near-infrared laser treatment.
Introduction
Photodynamic therapy (PDT) has gained wide interest among current superficial cancer treatment options such as surgery, radiotherapy, and chemotherapy, as it is minimally invasive compared with the other treatment options.Citation1–Citation4 For example, both surgery and radiation may damage adjacent normal tissues. Surgery needs a certain period of time for wound recovery, and chemotherapy is a highly cytotoxic treatment that affects tissues and organs. PDT is a procedure that utilizes light-active photosensitizers to convert cellular oxygen to toxic reactive oxygen species to kill tumor cells.Citation4–Citation6 Therefore, the therapy can be highly localized to minimize systemic side effects.Citation7 As the photosensitizers are not consumed in PDT, repeatable treatment can be achieved with one administration. However, conventional PDT is limited to superficial tumors because the current efficient photosensitizers (chlorin,Citation8 zinc phthalocyanine,Citation9–Citation11 porphyrin,Citation12 and texaphyrinCitation13) need UV or visible light for the activation, which has limited penetration depth in tissue. Near-infrared (NIR) light has a greater penetration depth in tissue than UV-visible light.Citation14,Citation15 Photosensitizers such as indocyanine green (absorption at 800 to 810 nm)Citation16,Citation17 and aluminum sulfophthalocyanine (790 nm)Citation18 that absorb the NIR directly have been used in PDT, but they are less effective as the yields of the triplet state appear low compared with other photosensitizers used in PDT.Citation16,Citation19,Citation20 Upconversion nanoparticles (UCNs) provide alternative options to perform NIR PDT. UCNs are inorganic luminescent materials made from lanthanide elements.Citation21,Citation22 UCNs absorb NIR light but emit UV-visible light, which can be used as a transducer to perform NIR PDT with a wide range of clinically approved photosensitizers for deep-seated solid-organ tumors.Citation23 PDT assisted with UCNs has several advantages.Citation11,Citation24 First, the treatment can reach deep tissues because NIR light is used to excite UCNs and further activate photosensitizers. UCNs exhibit high chemical stability and photostability, with less decomposition in tissue and do not show photobleaching or photoblinking effect.Citation25 Second, UCNs serve as both light transducers and photosensitizer carriersCitation26 by attaching the photosensitizers on it and delivering them to the site. Third, a wide range of photosensitizers can be carried by UCNs regardless of the hydrophobicity. UCNs can easily be modified so that the photosensitizers can be attached to UCNs by physical or chemical methods. Finally, the fate of the photosensitizers can be imaged at the same time, without autofluorescence and high signal-to-noise ratio, as biological tissues usually cannot convert shorter wavelength light.
After Zhang et alCitation27 developed a method to coat UCNs with a silica shell, into which zinc-phthalocyanine photosensitizers were loaded, a number of researchers have adopted this method for photosensitizer loading on UCNs.Citation28–Citation31 This strategy demands certain efforts in materials fabrication and may lead to the premature release of photosensitizers. Recently, Wang et alCitation32 encapsulated both the photosensitizer (zinc phthalocyanine) and UCNs into OQPGA-PEG/RGD/TAT octadecyl-quaternized modified poly (γ-glutamic acid) (OQPGA)-polyethylene glycol (PEG)/arginylglycylaspartic acid (RGD, a tripeptide composed of L-arginine, glycine, and L-aspartic acid)/trans-activating transcriptional activator (TAT) lipid micelles for performing PDT. In a very recent work, Park et alCitation23 encapsulated PEGylated phospholipids and amine-functionalized UCNs to trap chlorin e6 (Ce6) in hydrophobic phospholipid for PDT. Covalent coupling of photosensitizer molecules appears to be a better option. Liu et alCitation33 described a covalent bonding strategy to link a photosensitizer molecule, rose bengal, onto UCNPs by conjugating carboxyl groups on hyaluronic acid–modified rose bengal to UCNs via amide bonds.
In this work, we synthesized UCNs made from NaYF4:Yb,Tm and conjugated with Ce6, a clinically approved photosensitizer.Citation13,Citation34–Citation36 To demonstrate that the UCN assisted PDT, Ce6 will be covalently conjugated to UCNs. As compared to the other methods used for photosensitizer delivery, such as encapsulation in polymerCitation32 and silicaCitation28 and electrostatic attachment,Citation37 a covalent bond is more robust for the delivery of the photosensitizers to the target site and to prevent premature release. The results on MCF-7 cells showed that effective photodynamic therapeutic effects after NIR irradiation and imaging can be done simultaneously.
Materials and methods
Synthesis of NaYF4:20%Yb,0.3%Tm UCNs
All chemicals were purchased from Sigma-Aldrich (Singapore) and used without further purification. NaYF4:Yb,0.3%Tm nanocrystals were synthesized following reported protocolsCitation16,Citation19 with modification. YCl3 (0.8 mmol), YbCl3 (0.2 mmol), and TmCl3 (0.003 mmol) were mixed with 6 mL of oleic acid and 15 mL of 1-octadecene. The mixture was heated to 150°C to form a homogeneous solution, and then cooled down to room temperature. A solution of 4 mmol NH4F and 2.5 mmol NaOH in 10 mL of methanol was added to the above solution and stirred for 30 minutes. Subsequently, the solution was slowly heated to remove the methanol. To completely remove methanol and remaining water, vacuum was applied for 10 minutes. The solution was then heated to 300°C for 1.5 hours under an argon atmosphere. The nanoparticles were precipitated by centrifugation with acetone and washed thrice with ethanol/water (1:1 v/v). Finally, UCNs were dispersed in cyclohexane for subsequent use.
Functionalization of UCNs with amino groups
Amino groups were grafted on UCNs by modified Stöber method with (3-aminopropyl) triethoxysilane (APTES). A volume of 0.25 mL of Igepal CO-520, 4 mL of cyclohexane, and 1 mL of 0.02 M UCNs in cyclohexane were added in a bottle followed by sonication. Ammonia (0.04 mL, 33 wt%) was then added to the bottle. It was shaken vigorously to form a transparent emulsion. Five microliters of tetraethyl orthosilicate was added, and the bottle was shaken at 60 rpm for 24 hours. Five microliters of APTES was next added to the solution followed by shaking at 60 rpm for 24 hours. The silica-coated UCNs were precipitated by centrifugation with ethanol, washed twice with ethanol/water (1:1 v/v), and finally stored in deionised (DI) water.
Conjugation of Ce6 to amino-modified UCNs
Ce6 was covalently conjugated to UCNs with the aid of ethylcarbodiimide hydrochloride (EDC) and N-hydroxysuccinimide (NHS). Amino group–modified UCNs (0.15 mmol) were dispersed in 8 mL of DI water followed by sonication for 20 minutes. One microliter of 0.2 mg mL−1 NHS and 1 mL of 0.3 mg mL−1 1-(3-dimethylaminopropyl)-3-EDC were added to the UCN with vigorous shaking for 30 minutes. Subsequently, 3.5 mg of Ce6 was added to the activated nanoparticles and shaken at 4°C for 24 hours. The nanoparticles were washed twice with water by centrifugation at 5,000 rpm for 10 minutes. Finally, the UCNs-Ce6 conjugates were resuspended in 8 mL of DI water.
Singlet-oxygen generation from UCN-Ce6 in aqueous solution
The UCN-Ce6 nanoparticles (3.7 mg mL−1) were dispersed in 996 μL of air-equilibrated UV water. The stock solution of singlet-oxygen sensor green (SOSG, Invitrogen) was diluted to a final concentration of 5 mM. The solution requires protection of the SOSG from light during the experiments. Four microliters of the freshly prepared SOSG stock solution was added to the above UCN-Ce6 aqueous solution followed by vortexing the solution. The solution was then placed in the holder of a spectrophotometer equipped with continuous, tunable-wavelength UV-visible lamp and continuous-wave 980 nm laser. The spectrum was recorded with 488-nm excitation after irradiation with 1.5-mA, 980 nm laser irradiation for 15 seconds up to 1 hour. The 980 nm laser is turned off when recording SOSG emission at 488 nm. The spectra of UCN-Ce6 were recorded with newly prepared sample with the same amount of SOSG with 980 nm excitation.
Cell viability by MTT assay
Cell viability was tested with human breast adenocarcinoma cell line MCF-7 cancer cells using the established colorimetric MTT assay for quantification. Cells were seeded at 2.0×104 cells cm−2 in the 96-well plates for quantitative cytotoxicity experiments and cultured as a monolayer. Cells were incubated with the culture medium containing varying concentrations of nanoparticles in five replicates at 37°C in a humidified atmosphere containing 5% CO2. After 24 hours of incubation, the cells were washed with 1× phosphate-buffered saline. Hundred microliters of 0.1 mg mL−1 of MTT was added to each well, and the cells were incubated for 4 hours. The solution was then gently removed and 100 μL of dimethylsulfoxide (DMSO) was added to dissolve the purple formazan crystals from viable cells. The optical density of the purple formazan crystals was determined at 570 nm with a reference at 630 nm by an absorbance microplate reader (Infinite® 200 PRO, Tecan). The viability is calculated by the optical density of the samples divided by that of control cells without nanoparticle treatment and presented as percentage viability of the control cells.
Confocal imaging of PDT effect of UCN-Ce6 on MCF-7 cells
UCNs and cells co-stained by fluorescein diacetate (FDA)/propidium iodide (PI) were visualized by a confocal laser scanning microscope (Nikon C1 Confocal, Nikon Inc., Tokyo, Japan) specially fitted with a continuous-wave, 980 nm laser excitation source (Opto-Link Corp., Hong Kong). The 980 nm laser was used to excite UCN to trigger PDT and observe upconversion (UC) cell image. Excitation at 488 nm was used to obtain FDA/PI fluorescent cell image of live/dead assay. All the confocal images were taken with a 20× objective lens.
Live/dead assay of MCF-7 cells for PDT effect of UCN-Ce6
MCF-7 cells were incubated with UCN-Ce6 (50 μg mL−1 and 100 μg mL−1) for 3 hours at 37°C, 5% CO2. In parallel, 50 μg mL−1 and 100 μg mL−1 amino group-grafted silica-coated UCNs were used as negative control. Noninternalized nanoparticles were washed away with phosphate-buffered saline twice. To assess cell viability, 60 μL of FDA (1 μg mL−1) and PI (2 μg mL−1) mixture were added to each well for 10 minutes before confocal imaging. Excitation wavelength of 488 nm was used, and emissions were collected at 513 nm and 617 nm. Samples were irradiated with NIR laser (980 nm) at 5-minute intervals for 20 minutes to study the effects of UPCN on cells.
Determination of power density of 980 nm laser on confocal microscope for live/dead assay
As the laser on the confocal microscope first passes through the objective lens before reaching the sample, the laser beam size will be different if different magnification is used. Therefore, the power density depends on the objective lens used for image taking. All the live/dead assay confocal images were taken with 20× objective lens, so laser power density on the confocal microscope can be determined by taking FDA/PI-stained cell imaging under higher magnifications. In detail, 100 mg mL−1 UCN-Ce6 was incubated with MCF-7 cells for 4 hours. Noninternalized UCN-Ce6 was washed with phosphate-buffered saline twice. Subsequently, 100 μL of DMEM culture medium was added to the cells at 37°C. Then 60 μL of FDA (1 μg mL−1) and PI (2 μg mL−1) mixture was added to the cells and shaken gently to make a homogeneous solution for cell uptake. After 10 minutes, the cell plate was placed on the confocal platform. The NIR scanning channel was enabled through 20× objective lens and shut off after 10 minutes. The confocal image was first taken with 20× objective lens and then with 10× and 4× lenses without moving the position of the cell culture plate. By measuring the area with dead cells in red, and the power with a power meter at the position of cell culture plate placed, the power density used for the scan was 191 mW cm−2.
Instrumentation
Transmission electron microscope (TEM) images were taken with a Philips EM300 TEM operating at an accelerating voltage of 300 kV. TEM samples were obtained by dropping the nanoparticle dispersion on a 300-mesh formvar film–coated copper grid. UCN was dispersed in cyclohexane, while UCN-SiO2-NH2 and UCN-SiO2-Ce6 were dispersed in DI water. A Bruker GADDS D8 Discover diffractometer with Cu Kα radiation (λ=1.5418 Å) was used to obtain X-ray diffraction patterns of the dry powder of UCN samples. A SpectraPro 2150i fluorescence spectrometer equipped with continuous-tuning, excitation-wavelength UV-visible lamp and 980 nm NIR laser was used to acquire both emission spectra at 488 nm excitation and upconverted emission spectra at 980 nm. Samples for the photoluminescence were made by dispersing UCN and UCN-SiO2-NH2 in cyclohexane and DI water, respectively. The spectra was normalized to emission peak at 453 nm. Fourier-transform infrared (FTIR) spectra were recorded with a Shimadzu IR Prestige-21 spectrometer by grinding freeze-dried samples with KBr in a pellet. 1H nuclear magnetic resonance (NMR) spectra were recorded on a Bruker AV-400 NMR spectrometer at 400 MHz by dispersing the freeze-dried Ce6, UCN-SiO2-NH2, and UCN-SiO2-Ce6 in D2O at room temperature. The acquisition time was set to 32 rounds with a pulse repetition time of 2.0 seconds. Live/dead assay with FDA/PI staining on MCF-7 cells was visualized by excitation at 488 nm using a confocal laser scanning microscope (Nikon C1 Confocal, Nikon Inc.). It is specially equipped with a continuous-wave, 980 nm laser excitation source (Opto-Link Corp.) used to obtain UC images. All the images were in 256×256 pixels.
Results and discussion
Synthesis and characterization of UCN-Ce6 hybrid nanoparticles
NaYF4:Yb,Tm UCNs were synthesized in 1-octadecene with oleic acid as capping agent. Then, a thin layer of amino group–functionalized silica was coated on UCNs with tetraethyl orthosilicate and APTES using the modified Stöber method,Citation28,Citation38 as shown in . Ce6 was covalently conjugated to UCNs via the amino groups on UCNs and carboxylic acid on Ce6 using EDC-NHS coupling, a well-established method to covalently conjugate carboxyl group and amino group with NHS and 1-(3-dimethylaminopropyl)-3-EDC. Ce6 is a high-quantum yield photosensitizer with singlet-oxygen (1O2) production efficiency of 0.64 at pH 7–8.Citation39,Citation40 After UCN-Ce6 nanocomplex was internalized into a cell, electrons of Ce6 will be promoted to the excited state upon 980 nm NIR irradiation. This is shown by the emission peak (330–360 nm) of UCNs. Subsequently, the excitation energy will be transferred to ground-state dioxygen (O2) resulting in electrophilic singlet-oxygen (1O2) formation and electrons of the photosensitizer returning to the ground state. 1O2 can react with electron-rich compounds such as lipids,Citation41 amino acids,Citation42 guanine moiety of nucleosides, oligonucleotides, and isolated and cellular DNA.Citation43,Citation44 The short lifetime of 1O2 (half-life time 4 μs in H2O)Citation45 ensures the high specificity of the treatment, as 1O2 cannot diffuse far away from the target site within its active period.
Figure 1 Schematic showing the process for preparing NaYF4:Yb,Tm UCN-Ce6 for photodynamic therapy and the structure of Ce6.
Abbreviations: APTES, (3-aminopropyl) triethoxysilane; Ce6, chlorin e6; EDC, ethylcarbodiimide hydrochloride; NHS, N-hydroxysuccinimide; NIR, near-infrared; TEOS, tetraethyl orthosilicate; UCN, upconversion nanoparticle.
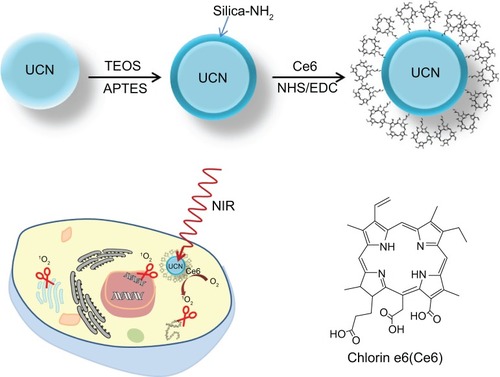
The morphology and size distribution of synthesized UCNs were observed under TEM. Before modification, the oleic acid–capped UCNs were well patterned on the TEM copper grid with uniform size (). X-ray diffraction pattern () showed that the nanoparticles were in hexagonal crystal phase, which matched perfectly with β-NaYF4.Citation46,Citation47 After silica coating, a thin layer of silica (2–3 nm) was coated on the surface of the UCNs (). As the surface of the UCN was rendered hydrophilic by the coating process, the nanoparticles cannot spread well on the formvar film–coated TEM grid as they were coated before. This is attributed to the hydrophobicity difference between the nanoparticles and the TEM grid film–promoted nanoparticle aggregation resulting in clustered nanoparticles on the copper grids as opposed to their former dispersed condition in solution. Furthermore, Ce6 conjugation appeared not to change the nanoparticle size by much as it is only a small molecule (). The successful coating of silica with amino groups and conjugation of Ce6 were also confirmed with FTIR. The wide absorption bands between 820 and 1,290 cm−1 showed superimposition of various SiO2 peaks and Si–OH bonding. The absorption peaks at 1,080 cm−1 (νas[SiOSi]) and 670 cm−1 (νs[SiOSi])Citation48 in upper curve of confirm the silica coating on UCNs. The peak around 1,550 cm−1 (NH2 scissoring)Citation49 confirmed the amino group grafted on the UCNs. After the conjugation of Ce6, this peak disappeared and Si–OH peak at 1,550 cm−1 weakened. This indicated that the EDC-NHS chemistry was successful as most of the NH2 groups had been utilized to react with Ce6 carboxyl groups. The broad peak around 3,500 cm−1 can be assigned to O–H groups,Citation50 which may come from the remaining moisture present in the samples and hydrogen bonding contributions. As a complementary confirmation of UCN-Ce6 conjugation, 1H-NMR was also carried out in D2O. The 1H-NMR spectra showed the presence of ethoxy groups: CH3 peaks at 1.05 ppm (labeled c in ) and CH2 of silanols at 3.7 ppm (labeled a in ).Citation48 Active hydrogen–deuterium exchange of proton in D2O solvent with protons in amino groups made signals from NH2 groups (δ=1.78 ppm) unintensive. After Ce6 was conjugated to the nanoparticles, CH3 peaks (δ=1.05 ppm) from APTES remained, and the triplet peak from CH3 in Ce6 (labeled d in ) can be observed in the spectra. All the results confirmed that Ce6 had conjugated to the amino-modified UCNs.
Figure 2 (A–F) Transmission electron microscope images of NaYF4:Yb,Tm UCN, amino group–modified silica–coated UCN and Ce6-conjugated UCN. (G) X-ray diffraction pattern of NaYF4:Yb,Tm UCN. (H) FTIR for UCN-SiO2-NH2, Ce6, and UCN-SiO2-Ce6.
Abbreviations: Ce6, chlorin e6; FTIR, Fourier-transform infrared; UCN, upconversion nanoparticle.
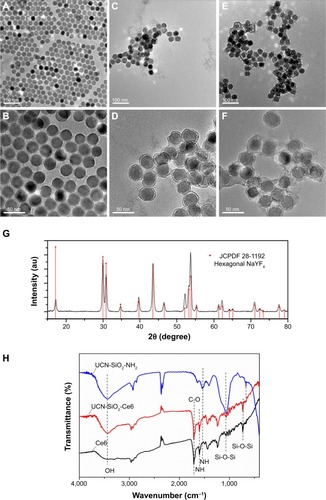
Singlet-oxygen generation by UCN-Ce6 complex
As the singlet-oxygen (1O2) generation by UCN-Ce6 in the proposed NIR PDT relies on the energy transfer from UCN to Ce6, the generation of 1O2 can be indirectly monitored by emission quenching of UCN and emission spectra of 1O2 indicators. First, the UCN emission at 980 nm excitation was recorded as initial reference (). After Ce6 conjugation, significant quenching of the UCN emission peak excited at 980 nm was observed in the range of 330–360 nm, which corresponds to the absorbance range of Ce6. This showed that the emission energy from UCN was absorbed by Ce6 further supporting the earlier results that the UCN and Ce6 were conjugated together in a close proximity where the energy transfer is able to happen.Citation51,Citation52 Meanwhile, SOSG, which emits green fluorescence around 504–525 nm in the presence of 1O2, was used as an indicator to monitor the generation of 1O2. This indicator is highly selective for singlet oxygen, the intensity of which is proportional to the amount of 1O2 generation. One milliliter of air-equilibrated UCN-Ce6 UV water solution was mixed with 4 μL of SOSG in methanol to make a homogeneous solution. As the concentration of 1O2 is proportional to the emission of SOSG, 1O2 detection in UCN-Ce6 aqueous solution can be monitored by recording the emission of SOSG at 488 nm excitation after certain time (from 15 seconds to 1 hour) of 980 nm irradiation. shows that SOSG emission signal increased by prolonging the NIR irradiation time, which indicated that the 1O2 amount in the aqueous solution kept increasing under NIR irradiation. In contrast, the random mixture of UCN, Ce6, and SOSG in air-equilibrated water did not show significant fluorescence with NIR irradiation up to 1 hour (), indicating no 1O2 generation in this situation. This further confirmed that Ce6 was covalently conjugated to UCN, and a random mixture cannot efficiently induce 1O2 generation.
Figure 3 (A) Emission spectra of silica-coated UCN (UCN-SiO2-NH2, dotted curve, λex=980 nm) and UCN-Ce6 (solid curve, λex=980 nm). (B) Integration of SOSG emission spectra of UCN-Ce6 solution (λex=488 nm) with respect to time. (C) Emission spectra of SOSG (λex=488 nm).
Abbreviations: Ce6, chlorin e6; PL, photoluminescence; SOSG, singlet-oxygen sensor green; UCN, upconversion nanoparticle.
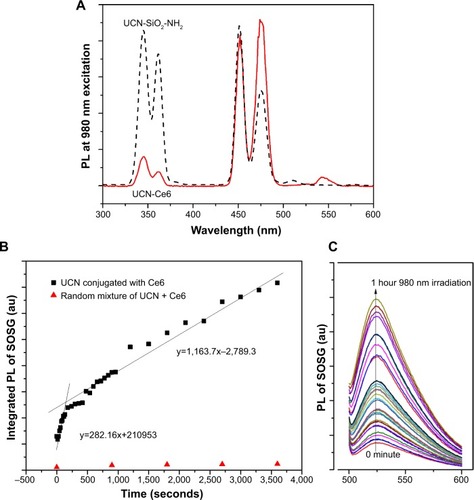
To investigate the 1O2 generation with time, the emission spectrum of SOSG was integrated and plotted against time in . This curve shows that the rate of 1O2 generation can be divided into two first-order linear ranges with irradiation time. The 1O2 generation was faster in the first 5 minutes because of the use of air-saturated water at the beginning of the measurement. The initial concentration of oxygen in water was higher for faster 1O2 generation. As the consumption of the oxygen molecules in the solution reached a relatively lower level, the oxygen absorption by water and consumption maintained equilibrium.Citation53 Then the 1O2 generation followed linear relation finally. It was reported that the generation of 1O2 was of first order with respect to irradiation time up to 100 minutes if no quenchers of the photosensitizer were present.Citation54 A similar linear relation of 1O2 generation with time was also observed in air-saturated alcohols.Citation55 During the 1O2 detection, no quencher was present, and none of the parameters were changed except the dissolved oxygen in solution. Therefore, it is reasonable that the 1O2 amount is following the first-order relation with respect to time.
In vitro photodynamic effect of UCN-Ce6
Cytotoxicity of UCN-Ce6
To make sure the UCN-Ce6 can be safely used for PDT, cytotoxicity was assessed with the MCF-7 cell line without NIR irradiation. Different concentration of UCN-Ce6 ranging from 12.5 μg mL−1 to 400 μg mL−1 was incubated with MCF-7 cells for 24 hours. The cell viability was measured by the MTT assay. The results () showed that the cells, in the concentration that we used in this experiment (50 μg mL−1), were more than 90% viable. This indicates that the toxicity of the UCN-Ce6 complex was very low in the effective concentration range.
Figure 4 (A) Cell viability of MCF-7 cells treated with different concentrations of UCN-Ce6 using a typical MTT assay without NIR irradiation or in the dark. (B) Cell viability of MCF-7 cells treated with 50 μg mL−1 UCN-Ce6 under different powers of NIR for 10 minutes.
Abbreviations: Ce6, chlorin e6; MTT, 3-(4,5-dimethylthiazol-2-yl)-2,5-diphenyltetrazolium bromide; NIR, near-infrared; UCN, upconversion nanoparticle.
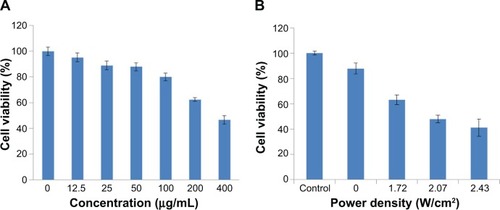
In vitro photodynamic study
The in vitro PDT effect was evaluated by two methods: (1) MTT assay after treatment with UCN-Ce6–incubated cells with NIR and (2) direct observation of the cell viability under confocal microscope.
For the MTT method, four groups of MCF-7 cells, triplicates for each group, were incubated with 50 μg mL−1 of UCN-Ce6 nanocomplex. The internalization of UCN-Ce6 nanocomplex was confirmed by the UC image with blue emission from UCNs in . Subsequently, the cells were exposed under 980 nm NIR laser for 10 minutes at various power densities. The viability of the treated cells was assessed by MTT assay later. showed that more cells were killed when higher power was applied. The results showed that the 50 μg mL−1 dose was sufficient for PDT with relatively low dose of laser irradiation. In the clinical trial, the optimal choice is with lower dosage of both drug and laser treatment as long as lesions can be removed. Therefore, it is promising that 50 μg mL−1 of UCN-Ce6 nanocomplex with 10 minutes of mild laser treatment could kill half of the cells.
Figure 5 Confocal fluorescent microscopy images of MCF-7 cells incubated with 50 μg mL−1 UCN-Ce6 counterstained with fluorescein diacetate/propidium iodide after 980 nm NIR irradiation (191 mW cm−2).
Abbreviations: Ce6, chlorin e6; NIR, near-infrared; UCN, upconversion nanoparticle.
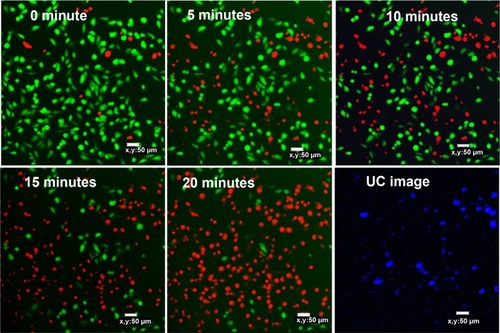
The second method used to evaluate the PDT effect on cells was live/dead assay with confocal microscope. Two fluorescent dyes, FDA and PI, were used for staining the live and dead cells, respectively. FDA is an acetylated derivative of fluorescein, the acetyl groups enable it to passively diffuse via phospholipid bilayer. It is nonfluorescent until it is converted to fluorescein by esterases in cytoplasm. Thus, the live cells show green fluorescence when FDA is present. PI is a very weak red fluorescent dye, which can be excited 488 nm. PI cannot enter cells through cell membrane of live cells, but its fluorescence can be enhanced 20- to 30-fold after it bound to nucleic acids. So if the cells are stained with FDA and PI together, strong red fluorescence is observed and simultaneously green fluorescence disappears when the cell is dead.Citation56
After staining MCF-7 cells with FDA/PI, confocal images were taken at 5-minute intervals with 980 nm irradiation excited with 488 nm laser. showed that cells gradually died with the extension of the NIR irradiation time. After 20 minutes of NIR irradiation, more than 90% cells were dead. The power-dependent test with MTT assay showed that the same efficacy can be achieved by higher power of NIR irradiation in a shorter time (10 minutes). This treatment is highly specific and confined to the cells stained with UCN but irradiated with 980 nm light, which is reflected by comparing the confocal images between UC image and 20-minute irradiation in . As the image resolution is lower for longer wavelength light with the same NA (numerical aperture) for the same objective lens (EquationEquation 1(1) ),Citation57 UCN emission recorded in the image was much less than the actual, so there were UCN-Ce6 present even though these were not shown in the UC image. As the lifetime of singlet oxygen is in the millisecond range, only the cells that have treated the UCN-Ce6 can be targeted.
On the other hand, the control samples for observing the toxicity of 980 nm irradiation, MCF-7 cells stained with FDA/PI exposed to 980 nm irradiation following the same protocol as described previously, did not show significant change after 20 minutes of treatment (). Another negative control, MCF-7 cells counterstained with FDA/PI and 50 ppm UCN-SiO2-NH2 exposed to 980 nm irradiation for 20 minutes, did not show significant cell death due to the treatment ().
Conclusion
In this work, we synthesized a new drug model photosensitizer conjugated to UCN nanocomplex. This is used for PDT by utilizing NIR as a trigger for deep tissue treatment of cancers. The photosensitizer was covalently conjugated to the UCNs. The nanocomplex was small and displayed high monodispersity in its size. The robust bond prevents the undesired premature release of photosensitizer. Singlet-oxygen generation rate from this nanocomplex was evaluated in solution, which will be useful to decide the PDT treatment time. The PDT effect was assessed in vitro in cells using two different methods. From the results, it can be seen that the NIR PDT with UCN-Ce6 is a highly specific and targeted treatment option. Promising efficacy can be achieved with low concentration and mild dosage of this nanocomplex. By adjusting the laser power and UCN-Ce6 dosage, the efficacy of this nanocomplex can be fine-tuned. With further development, the clinical NIR PDT can be explored for wider applications for cancer treated in vivo.
Author contributions
Xian Jun Loh, Qing Qing Dou, and Enyi Ye conceived the research idea. Qing Qing Dou performed experiments and drafted the manuscript. Choon Peng Teng performed the cell experiments and participated in the writing of the paper. Xian Jun Loh finalized the manuscript. All authors contributed toward data analysis, drafting, and revising the paper and agree to be accountable for all aspects of the work.
Acknowledgments
The authors acknowledge the financial support (grant IMRE/13-2P0806) and technical support from the Institute of Materials Research and Engineering, National University of Singapore.
Supplementary materials
Figure S1 NMR of amino group–modified silica–coated UCN (UCN-SiO2-NH2), Ce6, and Ce6-conjugated UCN (UCN-SiO2-Ce6).
Notes: Peaks given as a, b, and c, reflect the proton peaks corresponding to the positions shown in the molecular structure given in the inset of the NMR spectra.
Abbreviations: APTES, (3-aminopropyl) triethoxysilane; Ce6, chlorin e6; NMR, nuclear magnetic resonance; UCN, upconversion nanoparticle.
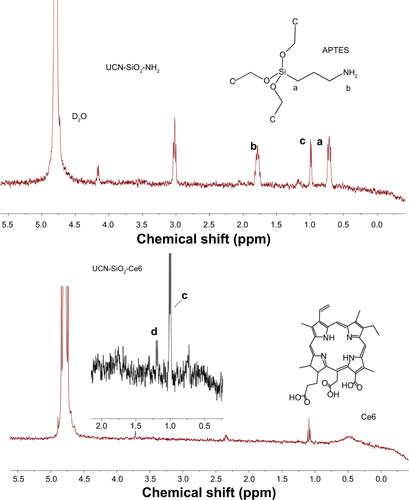
Figure S2 Effect of 980 nm irradiation without nanoparticles.
Notes: (A) Control (mixture of UCN, Ce6, SOSG) did not show any significant signal at 525 nm under NIR irradiation up to 60 minutes. (B) Fluorescence emission of SOSG from UCN-conjugated Ce6 nanocomplex in aqueous solution.
Abbreviations: Ce6, chlorin e6; NIR, near-infrared; SOSG, singlet-oxygen sensor green; UCN, upconversion nanoparticle.

Figure S3 Confocal images of MCF-7 cells incubated with 100 mg mL−1 UCN-Ce6 for up to 50 minutes of NIR irradiation, to determine the exposure area of NIR irradiation.
Abbreviations: Ce6, chlorin e6; NIR, near-infrared; UCN, upconversion nanoparticle.
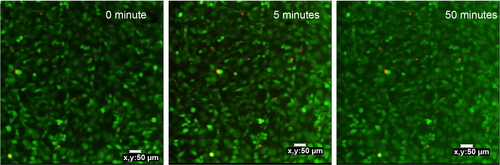
Figure S4 Effect of 980 nm irradiation with 50 mg mL−1 UCN-SIO2 nanoparticles.
Notes: Confocal image of MCF-7 cells stained with fluorescein diacetate/propidium iodide exposed under 980 nm irradiation for up to 20 minutes as negative controls.
Abbreviation: UC, upconversion.
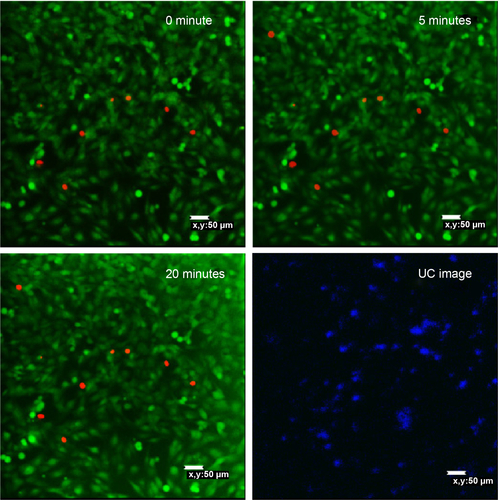
Figure S5 Confocal fluorescent microscopy images of MCF-7 cells incubated with 50 μg mL−1 UCN-Ce6 counterstained with fluorescein diacetate/propidium iodide after 980 nm NIR irradiation.
Note: This figure shows localised targeting effect based on the site of irradiation. Dotted yellow circles define the area which is exposed to 980 nm irradiation.
Abbreviations: Ce6, chlorin e6; NIR, near-infrared; UCN, upconversion nanoparticle.
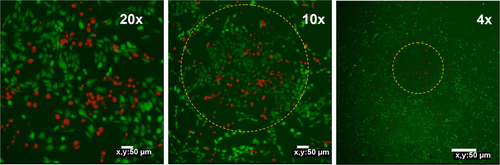
Disclosure
The authors report no conflicts of interest in this work.
References
- NguyenNTSchauerPLuketichJDMinimally invasive esophagectomy for Barrett’s esophagus with high-grade dysplasiaSurgery2000127328429010715983
- HirschbergHSpetalenSCarperSHolePTillungTMadsenSMinimally invasive photodynamic therapy (PDT) for ablation of experimental rat gliomaMinim Invasive Neurosurg2006490313514216921452
- GossnerLStolteMSrokaRPhotodynamic ablation of high-grade dysplasia and early cancer in Barrett’s esophagus by means of 5-aminolevulinic acidGastroenterology199811434484559496934
- DolmansDEFukumuraDJainRKPhotodynamic therapy for cancerNat Rev Cancer20033538038712724736
- RobertsonCEvansDHAbrahamseHPhotodynamic therapy (PDT): a short review on cellular mechanisms and cancer research applications for PDTJ Photochem Photobiol B20099611819406659
- BrownSBBrownEAWalkerIThe present and future role of photodynamic therapy in cancer treatmentLancet Oncol20045849750815288239
- CastanoAPDemidovaTNHamblinMRMechanisms in photodynamic therapy: part one – photosensitizers, photochemistry and cellular localizationPhotodiagnosis Photodyn Ther20041427929325048432
- SpikesJDNew trends in photobiology: chlorins as photosensitizers in biology and medicineJ Photochem Photobiol B1990632592742120404
- LukyanetsEAPhthalocyanines as photosensitizers in the photodynamic therapy of cancerJ Porphyrins Phthalocyanines1999306424432
- GuoHQianHIdrisNMZhangYSinglet oxygen-induced apoptosis of cancer cells using upconversion fluorescent nanoparticles as a carrier of photosensitizerNanomedicine20106348649520044035
- IdrisNMGnanasammandhanMKZhangJHoPCMahendranRZhangYIn vivo photodynamic therapy using upconversion nanoparticles as remote-controlled nanotransducersNat Med201218101580158522983397
- BonnettRPhotosensitizers of the porphyrin and phthalocyanine series for photodynamic therapyChem Soc Rev19952411933
- DettyMRGibsonSLWagnerSJCurrent clinical and preclinical photosensitizers for use in photodynamic therapyJ Med Chem200447163897391515267226
- HirschLRStaffordRJBanksonJANanoshell-mediated near-infrared thermal therapy of tumors under magnetic resonance guidanceProc Natl Acad Sci U S A200310023135491355414597719
- ZhouJSunYDuXXiongLHuHLiFDual-modality in vivo imaging using rare-earth nanocrystals with near-infrared to near-infrared (NIR-to-NIR) upconversion luminescence and magnetic resonance propertiesBiomaterials201031123287329520132982
- OmarGWilsonMNairSLethal photosensitization of wound-associated microbes using indocyanine green and near-infrared lightBMC Microbiol20088111118593460
- FunayamaTSakaneMAbeTOchiaiNPhotodynamic therapy with indocyanine green injection and near-infrared light irradiation has phototoxic effects and delays paralysis in spinal metastasisPhotomed Laser Surg2012301475322043821
- KoganBYButeninAVTorshinaNLKaliyaOLLukyanetsEAKoganEAAluminium sulphophthalocyanine as an NIR photosensitizer for PDTProc SPIE199935634
- BaumlerWAbelsCKarrerSPhoto-oxidative killing of human colonic cancer cells using indocyanine green and infrared lightBr J Cancer1999803–436036310408838
- AllisonRRDownieGHCuencaRHuX-HChildsCJHSibataCHPhotosensitizers in clinical PDTPhotodiagn Photodyn Therapy2004112742
- ChenGQiuHPrasadPNChenXUpconversion nanoparticles: design, nanochemistry, and applications in theranosticsChem Rev2014114105161521424605868
- HaaseMSchäferHUpconverting nanoparticlesAngew Chem Int Ed2011502658085829
- ParkYIKimHMKimJHTheranostic probe based on lanthanide-doped nanoparticles for simultaneous in vivo dual-modal imaging and photodynamic therapyAdv Mater201224425755576122915170
- WangCChengLLiuZDrug delivery with upconversion nanoparticles for multi-functional targeted cancer cell imaging and therapyBiomaterials20113241110112020965564
- WuSHanGMillironDJNon-blinking and photostable upconverted luminescence from single lanthanide-doped nanocrystalsProc Natl Acad Sci U S A200910627109171092119541601
- KonanYNGurnyRAllémannEState of the art in the delivery of photosensitizers for photodynamic therapyJ Photochem Photobiol B20026628910611897509
- ZhangPSteelantWKumarMScholfieldMVersatile photosensitizers for photodynamic therapy at infrared excitationJ Am Chem Soc2007129154526452717385866
- QianHSGuoHCHoPCLMahendranRZhangYMesoporous-Silica-coated up-conversion fluorescent nanoparticles for photodynamic therapySmall20095202285229019598161
- LimMELeeYLZhangYChuJJHPhotodynamic inactivation of viruses using upconversion nanoparticlesBiomaterials20123361912192022153019
- ZhaoZHanYLinCMultifunctional core – shell upconverting nanoparticles for imaging and photodynamic therapy of liver cancer cellsChem Asian J20127483022279027
- ShanJBudijonoSJHuGPegylated composite nanoparticles containing upconverting phosphors and meso-tetraphenyl porphine (TPP) for photodynamic therapyAdv Funct Mater2011211324882495
- WangHJShresthaRZhangYEncapsulation of photosensitizers and upconversion nanocrystals in lipid micelles for photodynamic therapyPart Part Syst Char2014312228235
- LiuKLiuXZengQCovalently assembled NIR nanoplatform for simultaneous fluorescence imaging and photodynamic therapy of cancer cellsACS Nano2012654054406222463487
- TriesscheijnMBaasPSchellensJHStewartFAPhotodynamic therapy in oncologyOncologist20061191034104417030646
- ChinWWLLauWKOHengPWSBhuvaneswariROlivoMFluorescence imaging and phototoxicity effects of new formulation of chlorin e6–polyvinylpyrrolidoneProc Natl Acad Sci U S A2006842103110
- Čunderlı´kováBGangeskarLMoanJAcid–base properties of chlorin e6: relation to cellular uptakeJ Photochem Photobiol B1999531–3819010672533
- PotvinPGLuyenPUBräckowJElectrostatic bubbles and supramolecular assistance of photosensitization by carboxylated Ru (II) complexesJ Am Chem Soc2003125164894490612696909
- Guerrero-MartínezAPérez-JusteJLiz-MarzánLMRecent progress on silica coating of nanoparticles and related nanomaterialsAdv Mater Deerfield2010221111821195
- FernandezJMBilginMDGrossweinerLISinglet oxygen generation by photodynamic agentsJ Photochem Photobiol B1997371–2131140
- RotomskisRValanciunaiteJSkripkaAComplexes of functionalized quantum dots and chlorin e6 in photodynamic therapyLith J Phys20135315768
- XIAOBoudreauMDZhouY-TYinJ-JFuPPUVB photoirradiation of aloe vera – formation of free radicals, singlet oxygen, superoxide, and induction of lipid peroxidationJ Food Drug Anal2011194165175
- DaviesMJSinglet oxygen-mediated damage to proteins and its consequencesBiochem Biophys Res Commun2003305376177012763058
- CadetJRavanatJLMartinezGRMedeirosMHGMascioPDSinglet oxygen oxidation of isolated and cellular DNA: product formation and mechanistic insightsPhotochem Photobiol20068251219122516808595
- Agnez-LimaLFMeloJTSilvaAEDNA damage by singlet oxygen and cellular protective mechanismsMutat Res201275111528
- JarviMTNiedreMJPattersonMSWilsonBCThe influence of oxygen depletion and photosensitizer triplet-state dynamics during photodynamic therapy on accurate singlet oxygen luminescence monitoring and analysis of treatment dose responsePhotochem Photobiol201187122323421143603
- LiZZhangYAn efficient and user-friendly method for the synthesis of hexagonal-phase NaYF 4:Yb, Er/Tm nanocrystals with controllable shape and upconversion fluorescenceNanotechnology2008193434560621730655
- LiZZhangYJiangSMulticolor core/shell-structured upconversion fluorescent nanoparticlesAdv Mater Deerfield2008202447654769
- Beganskiene˙ASirutkaitisVKurtinaitiene˙MJuškėnasRKareivaAFTIR, TEM and NMR investigations of Stöber silica nanoparticlesMater Sci (Medžiagotyra)200410287290
- LewisIRDanielNWGriffithsPRInterpretation of Raman spectra of nitro-containing explosive materials. Part I: group frequency and structural class membershipAppl Spectrosc1997511218541867
- ZecchinaABordigaSSpotoGSilicalite characterization. 2. IR spectroscopy of the inter0061ction of carbon monoxide with internal and external hydroxyl groupsJ Phys Chem1992961249914997
- LamAJSt-PierreFGongYImproving FRET dynamic range with bright green and red fluorescent proteinsNat Meth201291010051012
- TaraskaJWPuljungMCZagottaWNShort-distance probes for protein backbone structure based on energy transfer between bimane and transition metal ionsProc Natl Acad Sci U S A200910638162271623219805285
- SivéryABarrasABoukherroubRProduction rate and reactivity of singlet oxygen 1O2(1Δg) directly photoactivated at 1270 nm in lipid nanocapsules dispersed in waterJ Phys Chem2014118528852893
- KrishnaCMLionYRieszPA Study of 1O2 production by immoblilized sensitizer outside the solution. Measurement of 1O2 generationPhotochem Photobiol1987451163562573
- KrasnovskyAAJrRoumbalYVStrizhakovAARates of 1O2 (1Δg) production upon direct excitation of molecular oxygen by 1270 nm laser radiation in air-saturated alcohols and micellar aqueous dispersionsChem Phys Lett20084581–3195199
- BoydVCholewaOMPapasKKLimitations in the use of fluorescein diacetate/propidium iodide (FDA/PI) and cell permeable nucleic acid stains for viability measurements of isolated islets of LangerhansCurr Trends Biotechnol Pharm2008226620814586
- GustafssonMGNonlinear structured-illumination microscopy: wide-field fluorescence imaging with theoretically unlimited resolutionProc Natl Acad Sci U S A200510237130811308616141335