Abstract
Introduction
Invasive medical devices are used in treating millions of patients each day. Bacterial adherence to their surface is an early step in biofilm formation that may lead to infection, health complications, longer hospital stays, and death. Prevention of bacterial adherence and biofilm development continues to be a major healthcare challenge. Accordingly, there is a pressing need to improve the anti-microbial properties of medical devices.
Materials and Methods
Polydimethylsiloxane (PDMS) was doped with halloysite nanotubes (HNTs), and the PDMS-HNT composite surfaces were coated with PDMS-b-polyethylene oxide (PEO) and antibacterials. The composite material properties were examined using SEM, energy dispersive spectroscopy, water contact angle measurements, tensile testing, UV-Vis spectroscopy, and thermal gravimetric analysis. The antibacterial potential of the PDMS-HNT composites was compared to commercial urinary catheters using cultures of E. coli and S. aureus. Fibrinogen adsorption studies were also performed on the PDMS-HNT-PEO composites.
Results
HNT addition increased drug load during solvent swelling without reducing material strength. The hydrophilic properties provided by PEO were maintained after HNT addition, and the composites displayed protein-repelling properties. Additionally, composites showed superiority over commercial catheters at inhibiting bacterial growth.
Conclusion
PDMS-HNT composites showed superiority regarding their efficacy at inhibiting bacterial growth, in comparison to commercial antibacterial catheters. Our data suggest that PDMS-HNT composites have potential as a coating material for anti-bacterial invasive devices and in the prevention of institutional-acquired infections.
Introduction
Invasive medical devices circumvent the normal defense mechanism of the skin or mucous membranes and provide ideal environments where pathogens can proliferate from the patient’s immune defenses.Citation1 In addition, these devices provide a convenient vehicle for further microbial colonization or infection, transfer of pathogens from one part of the body to another, from health care worker to patient, from patient to health care worker, or from patient and health care worker to others.Citation1–Citation4
A key causal factor in these infections is the surface adsorption of serum proteins. Adsorbed serum proteins facilitate bacterial adhesion and proliferation on the surface of the device leading to biofilm formation.Citation5,Citation6 A biofilm is a multicellular community of microbes that forms on a solid surface or at a liquid–air interface.Citation7,Citation8 In a biofilm, microbes are densely packed within a self-assembled extracellular matrix that provides protection for resident bacteria from various environmental agents. In a very resistant biofilm, antibiotic resistance is higher, which increases bacterial pathogenicityCitation8–Citation10 and is the major reason that biofilm formation is responsible for a host of periprosthetic infections.Citation5,Citation9–Citation11 The standard treatment for severe postimplant infection is implant removal, the surrounding tissue cleaned of infection, and then, a second invasive device is implanted.Citation5,Citation6 For dental and orthopedic implants, revision arthroplasties and increased hospital stays can cost hundreds of thousands of dollars for a single patient, additional postsurgical complications and pain, reduced immune response, significant lost time from work, and altered and restricted lifestyles. Furthermore, catheter-associated urinary tract infections alone are the most common health care-associated infection worldwide and have an estimated total cost in the USA that ranges from $340 million to $450 million annually.Citation7 Accordingly, there is a pressing need to mitigate bacterial colonization by equipping the surfaces of biomedical devices and implants with features that alter surface chemistry and topography, thus generating a surface that is unfavorable for bacterial attachment.Citation4–Citation6
There has been a considerable research effort targeting the prevention of postsurgical infection after device insertion.Citation13–Citation16 The antimicrobial properties of antibiotics are well documented in the scientific literature, as well as the uses of silicones as catheter materials.Citation9–Citation12 Numerous attempts have been made to fabricate antimicrobial/antifouling coatings and composites for implantable medical devices, especially for the catheter market.Citation12,Citation13–Citation15 One approach to prevent biofilm formation is to coat the surface with bactericidal/bacteriostatic substances.Citation13–Citation15 Antibiotics have been employed to impregnate catheters, and titanium implants have been employed to prevent biofilm formation.Citation16,Citation17 Transitional metals incorporated into implant materials have been shown to possess antimicrobial activity.Citation18,Citation19 It is theorized that transition metals disrupt respiration and electron transport systems upon absorption into bacterial and fungal cells.Citation18–Citation20 Many of these products, however, have not been fully effective in clinical settings, and hospital-acquired infections derived from implantable medical devices remain the leading cause of long-term care infection.Citation21–Citation23
Various polymers have been studied as coatings for drug delivery including the polyurethanes, polyvinyl alcohol, polyacrylic acid, polyamide, polyvinyl pyrrolidone, polylactide, polyanhydrides, and polydimethylsiloxane (PDMS).Citation24–Citation26,Citation67,Citation68 PDMS is available commercially as a two-part kit with an elastomer base and a cross-linking agent. It is used in a wide range of biomedical applications as it possesses many attractive properties, including its ease of fabrication, hydrophobic nature, physiological inertness, biocompatibility, low toxicity, good thermal properties, and cross-linking capability.Citation26,Citation27 PDMS and its hydrophobic nature, however, suffer from serious biofouling problems due to protein adsorption, thus limiting its practical use. A significant effort has been directed toward the development of antifouling and antimicrobial PDMS coatings using physical and chemical methods to create a hydrophilic PDMS surface with an antifouling capability.Citation28–Citation32 Physical methods modify protein adsorption onto the PDMS surface via hydrophobic or electrostatic interactions or surface activation using oxygen plasma, ozone, or ultraviolet (UV) light.Citation28,Citation29,Citation31 The duration of antifouling of PDMS using physical modification is, however, temporary with the duration varying with treatment, and the surface undergoes hydrophobic recovery sometime after initial treatment.Citation30,Citation33,Citation34 In contrast, chemical modification of the PDMS surface forms covalent bonds between a PDMS substrate and coating materials, thus imparting stability and an antifouling property of longer duration.Citation25,Citation27,Citation28
The production of hydrophilic silicone coatings with antibacterial and antibiofouling properties has great potential for use in contact lenses, dialysis membranes, bladder and central venous catheters, and so on.Citation26–Citation28 Halloysite nanotubes (HNTs) are naturally occurring clay nanotubes mined from abundant mineral deposits making it an easily accessible nanomaterial. [28-30] HNT is structured as a two-layered aluminosilicate and has a predominantly hollow nanotubular structure in the submicron range.Citation35,Citation36 HNTs typically display an inner diameter between 15 and 50 nm, an outer diameter from 30 to 50 nm, and a length between 100 and 2000 nm.Citation35–Citation37 HNTs also present a large surface area and can be loaded and coated with a variety of materials, such as drugs, metals, and biomacromolecules.Citation38–Citation40 HNTs provide sustained release of loaded drugs, which increases the effectiveness of the drug without increasing strength. Drugs of smaller molecular size are trapped within the inner lumen of the HNT, and drugs with a larger molecular size can attach to the outer surface. Furthermore, the natural HNTs are much cheaper and more accessible, when compared to carbon nanotubes, offering great commercial potential.Citation41 HNTs have been shown to be cytocompatible in several cell types (with concentrations up to 0.1 mg/mL) including chondrocytes, dermal fibroblasts, osteoblasts, and stem cells cultured on HNT nanofilms or within HNT–hydrogel composites.Citation42–Citation44 All cell types proliferated and expressed specific marker proteins, showing that they maintained their cellular phenotype. A recently completed biocompatibility study in a rat dermal model showed that HNTs do not provoke a local cytotoxic response or a host immune response.Citation45
As HNTs have been shown to exhibit very low cytotoxicity and high levels of biocompatibility, they represent an ideal candidate for developing new drug delivery systems.
Here, we describe the fabrication of antibacterial and hydrophilic medical-grade PDMS substrates infused with the hydrophilic polyethylene oxide (PEO) and nitrofurantoin. The fabrication of the PDMS–HNT nanocomposites used bulk modification and solvent swelling/deswelling methods. Nanocomposites coated with PEO–coblock (b)–PDMS were studied with scanning electron microscopy (SEM) and water contact angle measurements. Pore size, tensile strength, thermal gravimetric analysis (TGA), and UV-visible spectroscopy were used to study implant material properties. The silicone/HNT nanocomposite’s potential for bacterial growth inhibition was assessed through an agar disc diffusion assay and lysogeny broth (LB) tests against Escherichia coli. The results showed that the addition of HNTs increased the drug load during solvent swelling without reducing material strength, and the hydrophilic properties provided by PEO were also maintained. In addition, PDMS–HNT composites were effective at inhibiting bacterial growth when compared to commercial catheters.
Materials
PDMS–PEO, methyl-terminated (molecular weight [MW] =600), was acquired from Polysciences Inc. (Warrington, PA, USA), and liquid silicone rubber 40 – 10:1, implant-grade, was purchased from Applied Silicone Corporation (Santa Paula, CA, USA). Translucent silicone rubber AM 128T was purchased from AeroMarine Products (San Diego, CA, USA). A rubber tensile die cutter was purchased from Jinan Hensgrand Instrument Co. (Jinan, China). Antibacterial intermittent catheters and silver-coated Foley catheter were purchased from leading commercial antibacterial catheters. Micro BCA Protein Assay Kit, 100-mm Mueller–Hinton agar plates, and Mueller–Hinton liquid broth were purchased from Thermo Fisher Scientific (Waltham, MA, USA). Nitrofurantoin (Nitro; 100 mg) susceptibility test discs were purchased from Becton Dickinson and Company (Franklin Lakes, NJ, USA). Halloysite nanoclay, LB powder growth medium, Mueller–Hinton broth and agar, Nitro crystalline, E. coli ATCC®11775™ 1000 colony-forming units (CFUs), Staphylococcus aureus ATCC 6538™ 50 CFUs, methylene blue, chloroform (high-performance liquid chromatography [HPLC]-grade), absolute ethanol, acetone (HPLC-grade), and bovine fibrinogen (≥70% protein basis) were purchased from Sigma-Aldrich (St. Louis, MO, USA).
Methods
Preparing PDMS–HNT composites
The fabrication of the PDMS–HNT nanocomposites was done using bulk modification and solvent swelling/deswelling methods. Medical-grade rubber polymers designed to meet the US Food and Drug Administration and International Organization for Standardization (ISO) 10993 biocompatibility requirements with Good Manufacturing Practice-based manufacturing and ISO 9001:2009-certified quality systems to support regulatory submissions for Class II and III medical devices were used for the nanocomposite fabrication. Implant-grade silicone samples were thoroughly mixed with HNTs (10% wt./wt.) and with a platinum curing agent (10:1). The uncured PDMS–HNT composites were poured into flat polystyrene Petri dishes and stored in a refrigerator at ≤8°C for 24 hours to remove trapped air. The samples were then cured in an oven at 60°C for 24 hours and were cut into circular discs (0.60 mm diameter and 0.2 mm thickness) using a sterile hole punch. A PDMS hydrophilic surface modification method was adapted from the method of Dr Harshil Dhruv.Citation47,Citation48 The samples were submerged in chloroform for 24 hours to remove residual cross-linker and unreacted monomers and then dried in an oven at 60°C for 24 hours. Next, the samples were placed in chloroform solutions with amphiphilic block copolymer PDMS–b–PEO (0%–5% [V/V]) for 24 hours, dried in an oven at 60°C for 24 hours, and then thoroughly rinsed with ethanol and dried.
For antibacterial in vitro studies, the treated PDMS–HNT composites were then placed in Nitro–acetone (5.1 mg/mL) for 24 hours and air-dried (PDMS–HNT–PEO–Nitro) to create a fully loaded PDMS substrate with an external drug crystallization layer. For enhanced visualization of the drug loading, PDMS and PDMS–HNT samples (200 mg) were immersed in methylene blue–acetone (0.7 mg/mL, [λ=661 nm]) solutions for 24 hours and monitored with a NanoDrop™ 2000c UV-Vis Spectrophotometer (Thermo Fisher Scientific).
SEM
A Hitachi S-4800 field emission scanning electron microscope (Chiyoda, Tokyo, Japan) was used to examine the nanocomposite surface topographies. PDMS–HNT composites treated with PDMS–b–PEO (0%–5% [V/V]) were adhered to a conductive adhesive tape and placed onto a stage for viewing. A gold sputter coating (4 nm) was applied to the surfaces with a 208 HR Metal Sputter Coater by Cressington Scientific Instruments (Watford, UK). Tensile-fractured PDMS and PDMS–HNT samples were also prepared for SEM viewing. The prepared stages were placed into the sample chamber and viewed with 1.0–2.0 kV. Energy-dispersive spectroscopy (EDS) elemental data analysis was also used to monitor the PDMS and PDMS–HNT composite surfaces.
Water contact angle measurements
OCA-15 plus by DataPhysics (San Jose, CA, USA) was used to capture water contact angle images and contact angle measurements; 1 µL of deionized water was pipetted onto the controls, and experimental samples were then imaged and measured at 20 and 200 seconds. Each contact angle reported here was the mean of at least three independent measurements.
Mechanical and thermal testing
Translucent PDMS and PDMS–10% HNT sheets were cut with a barbell-shaped ISO die cutter in triplicates and examined with an ADMET tensile tester (Norwood, MA, USA). Each sample placed in the testing clamps was stretched until failure and the maximum strength peaks were recorded. In addition, tensile fracture points of the samples were monitored with SEM/EDS.
Cured PDMS and PDMS (1%–10% HNTs wt./wt.) discs (0.60 mm diameter and 0.2 mm thickness) were analyzed by TGA to determine the effect of HNT concentration on the thermal degradation of PDMS using a Universal V4.5A (TA Instruments, New Castle, DE, USA).
Surface protein adsorption
A NanoDrop 2000c Spectrophotometer (Thermo Fisher Scientific) was used to monitor the protein adsorption properties of the modified PDMS–HNT composites with bovine fibrinogen. All samples were preequilibrated in phosphate-buffered saline (PBS; pH =7.4) for 24 hours. A bovine fibrinogen solution was prepared in PBS (pH =7.4; 1 mg/mL), and the samples were placed in the protein solution for 4 hours on a rocker. The samples were then thoroughly washed with PBS and sonicated in PBS (1% sodium dodecyl sulfate) solution for 30 minutes to desorb protein from surfaces. The product protocol for Micro BCA Protein Assay Kit was followed, and absorbance was monitored for each set of samples in triplicates.
Antibacterial testing
E. coli and S. aureus Vitroids™ were used to create 0.5 McFarland standard bacterial suspensions. Mueller–Hinton agar plates and broth were used to validate the antibacterial properties of PDMS–HNT–PEO–Nitro-loaded composites, controls, and commercial antibacterial catheters. The samples of each set were compared in triplicates after 24 hours of incubation at 37°C. Bacterial zones of inhibition (ZOIs) were monitored with a digital caliper, and the optical densities of the broths were monitored with a Genesys™ 20 Visible Spectrophotometer (λ=600 nm; Thermo Fisher Scientific).
Results
Surface morphology
SEM was used to study the surface morphology of unmodified and modified PDMS composites as a means for obtaining topographical information about surface features. Unmodified PDMS showed a fine granular surface that was uniform across the surface. The addition of different concentrations of PDMS–b–PEO into PDMS showed changes in surface roughness with a gradual increase in the graininess of the surface with percent increase in PEO addition (). PDMS loaded with 10% HNTs (wt./wt.) and coated with different concentrations of PDMS–b–PEO also showed a gradual increase in surface roughness for PDMS–HNT with the sequential addition of PDMS–b–PEO concentrations during solvent swelling although the surfaces had more coarse granules in contrast to the appearance of unmodified PDMS ().
Figure 1 SEM micrographs of PDMS coated with different concentrations of PDMS–b–PEO. (A) PDMS–0% PEO, (B) PDMS–1% PEO, (C) PDMS–2.5% PEO, and (D) PDMS–5% PEO. Surface roughness appeared to increase with the sequential increase of PDMS–b–PEO concentrations.
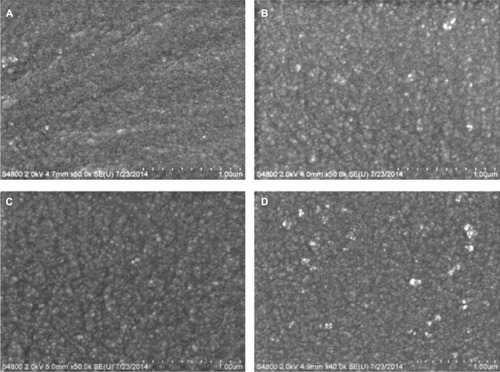
Figure 2 SEM micrographs of PDMS loaded with HNTs 10% (wt./wt.) and coated with different concentrations of PDMS–b–PEO. (A) PDMS–HNT–0% PEO, (B) PDMS–HNT–1% PEO, (C) PDMS–HNT–2.5% PEO, and (D) PDMS–HNT–5% PEO. Similar surface characteristics were observed with the HNT-loaded PDMS versions. Surface roughness appeared to increase with the sequential addition of PDMS–b–PEO.
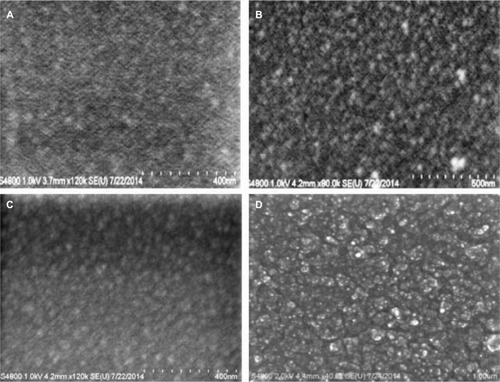
Water contact angles >100° were observed for the untreated PDMS and PDMS–HNT composites. The PDMS and PDMS–HNT composites treated with PDMS–b–PEO showed a significant reduction in contact angle measurements, as contact angles for both versions reached <10° ( and ). Previous studies and patentsCitation25–Citation27 have explored hydrophilic PDMS surface modifications; however, no research has been conducted with the addition of HNTs. These data confirmed that PDMS–HNT (up to 10% wt./wt.) maintains its hydrophilic properties when treated with PDMS–b–PEO.
Figure 3 Water contact angle images of treated and untreated PDMS. (A) 103.3° at 20 seconds on PDMS, (B) 97.7° at 200 seconds on PDMS, (C) 8.2° at 20 seconds on PDMS–PEO, and (D) 3.9° at 200 seconds on PDMS–PEO. Images displayed that the PEO additive significantly altered the PDMS surface wettability.
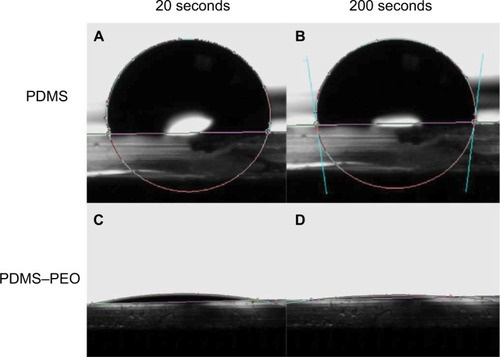
Figure 4 Water contact angle images on treated and untreated PDMS loaded with 10% HNTs (wt./wt.). (A) 103.8° at 20 seconds on PDMS–HNT, (B) 90.8° at 200 seconds on PDMS–HNT, (C) 15.8° at 20 seconds on PDMS–HNT–5% PEO, and (D) 8.7° at 200 seconds on PDMS–HNT–5% PEO. Hydrophilic properties were observed for the PDMS–HNT–PEO composites and showed that wettability was maintained with the addition of HNTs.
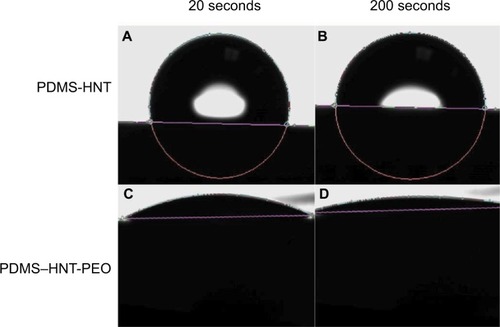
Strength test and thermal properties
The PDMS- and HNT-loaded composites cut with ISO rubber tensile die cutters showed similar maximum peak loads >60 N when measured by ADMET tensile tests. The PDMS–10% HNT average peak load was 6.98 kg, while the normal PDMS average was 6.33 kg (). The HNT-infused polymer demonstrated similar mechanical properties, suggesting a good dispersion of HNTs and slightly enhanced mechanical properties. SEM and EDS was used to study the tensile-fractured PDMS and PDMS–HNT surfaces. Under SEM, the normal PDMS showed very smooth or clean fractures, while the PDMS–10% HNT showed rough and jagged fracture patterns. The HNTs appeared to act as a cross-linking “ceramic skeleton” for the PDMS (). EDS results showed an increase in the silica and the presence of aluminum, which was from the presence of HNTs on the surface ().
Figure 5 SEM micrographs of tensile-fractured PDMS and PDMS–HNT surfaces. (A, B) PDMS, (C, D) PDMS–10% HNT. Different fracture patterns were noticed for PDMS and PDMS–10% HNT. Normal PDMS appeared to fracture smoothly, while the HNT-loaded versions displayed rougher fracturing patterns.
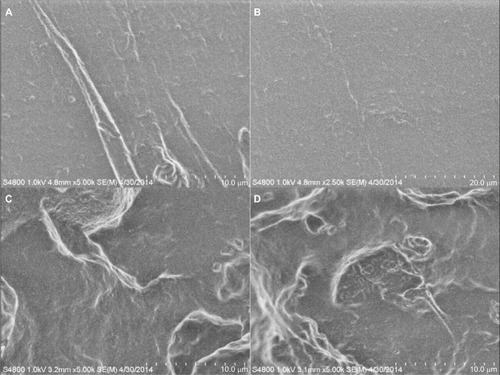
Figure 6 Images of energy-dispersive spectroscopic elemental data analysis for (left) PDMS and (right) PDMS–10% HNT. Spectra showed an increase in silica content and the presence of aluminum from the HNTs.
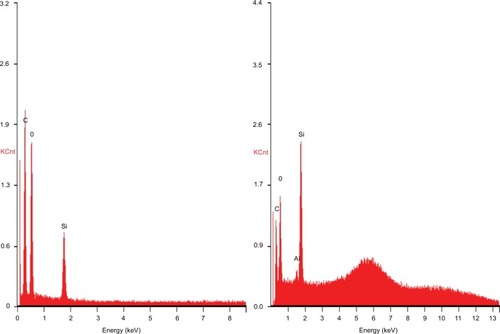
Table 1 Peak loads measured during tensile test for PDMS and PDMS–10% HNT composites at 5 MPa
The results of the TGA indicated a relationship between percent weight loss (weight %) versus temperature (°C) at different concentrations of HNTs in the formulation. It was concluded from the graphs () that when the concentration of HNTs was increased in the sample, thermal degradation temperature was decreased and the rate of weight loss was increased. indicates a relationship of the rate of weight loss of the formulation containing different concentrations of HNTs as a function of temperature. Furthermore, the dw/dt for the sample with 10% HNTs was highest which is 0.0015 at a temperature of 525°C while samples with low concentration of HNTs (7.5%, 5%, 2.5%, 1% and 0%) suggesting that there is a decrease in the rate of weight loss at different temperatures in descending order ().
Figure 7 (A)Thermal gravimetric analysis on PDMS and (B) PDMS with 1%–10% HNT addition.
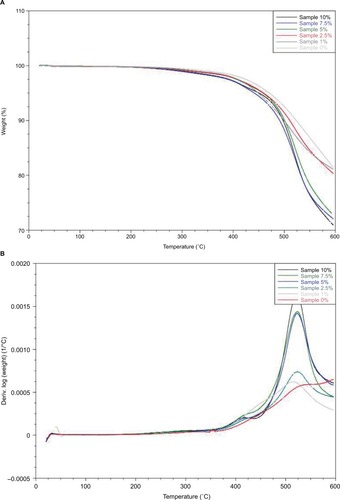
Drug loading/coating and in vitro studies
Methylene blue, a model drug, was used to visualize drug loading during solvent swelling. The PDMS–10% HNT composites showed superior drug loading capability when compared to the normal PDMS (). This is the first time that HNTs have been shown to increase the total drug load content in cured polymers, during solvent swelling methods. PDMS–10% HNT composites could load over double the amount of antibiotics and model drug content when compared to normal PDMS. During solvent swelling with drugs, the PDMS–HNT composites appeared to have higher adsorption rates and were speculated to have increased the PDMS average pore size. HNTs naturally display adsorptive properties and appeared to have contributed to the increased drug content loaded within the PDMS networks. In addition, drug crystallization layers were formed on the surfaces during the evaporation of the solvents. The fabrication process allowed for a combination of an outer drug layer and fully drug-infused polymer nanocomposite.
Figure 8 (A) Graph showing total drug load content for PDMS and PDMS–10% HNT after solvent swelling. (B) Image of PDMS (left) and PDMS–10% HNT (right) after solvent swelling in methylene blue–acetone solutions. This is the first time that HNTs have been shown to increase the total drug loading content in cured polymers using a solvent swelling method.
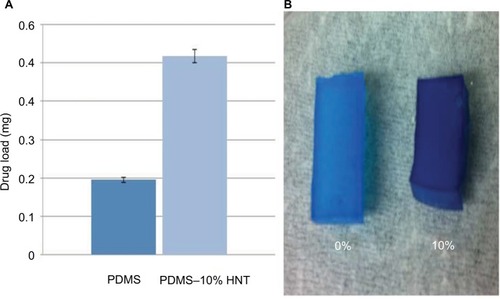
The PDMS–HNT–PEO composites were shown to reduce fibrinogen adsorption when compared to normal implant-grade PDMS. Micro BCA protein assays showed that total protein content was reduced on the composite surfaces when PDMS–b–PEO concentrations increased on the composite surfaces (). The results showed that PDMS–HNT–PEO composites could reduce surface protein content and offer additional drug loading/coating applications.
Table 2 Protein adsorption on PDMS and PDMS–10% HNT composites. Total protein content assay (Micro BCA protein assay). Reduction in surface-adsorbed fibrinogen was observed with PEO-treated PDMS–HNT surfaces
PDMS–HNT–PEO composites loaded and coated with Nitro showed competitive antibacterial performance when compared with commercial antibacterial urinary PDMS catheters (–; and ). The antibiotic- and HNT-loaded PDMS substrates showed short-term in vitro antibacterial properties against gram-negative and gram-positive bacteria. For Mueller–Hinton agar assays, the 100% PDMS catheters, silver catheter, and controls showed no zone of inhibition against gram-negative bacteria (; ). Mueller–Hinton broth tests showed that all samples displayed some inhibitory effects on bacteria growth. PDMS–HNT–PEO–Nitro and drug-coated catheters were shown to be the most effective at inhibiting both bacteria types in both agar and broth assays (–).
Figure 9 Images of Mueller–Hinton agar disc diffusion assays against Escherichia coli at 24 hours. (A) antibacterial catheter, (B) silver-coated catheter, (C) PDMS–HNT–PEO–nitrofurantoin, (D) 100% PDMS catheter, (E) PDMS–HNT–PEO, and (F) standard nitrofurantoin disc (100 mg).
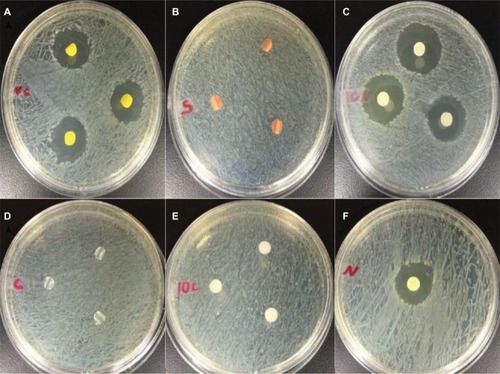
Figure 10 Images of Mueller–Hinton agar disc diffusion assay against Staphylococcus aureus at 24 hours. (A) antibacterial catheter, (B) silver-coated catheter, (C) PDMS–HNT–PEO–nitrofurantoin, (D) 100% PDMS catheter, (E) PDMS–HNT–PEO, and (F) standard nitrofurantoin disc (100 mg).
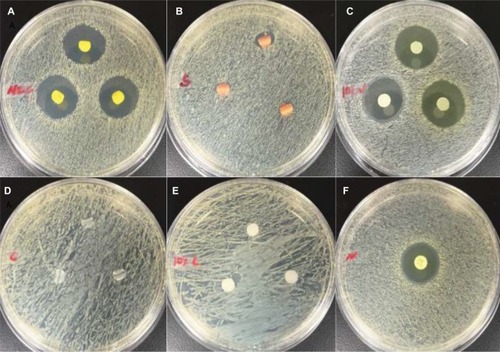
Figure 11 Images of Mueller–Hinton broth assays against Escherichia coli and Staphylococcus aureus at 24 hours. (1) E. coli, (2) S. aureus: (A1) Broth, (B1) control E. coli, (C1) 100% PDMS catheter, (D1) silver-coated catheter, (E1) antibacterial catheter, (F1) PDMS–HNT–PEO–nitrofurantoin, (A2) Broth, (B2) control S. aureus, (C2) 100% PDMS catheter, (D2) silver-coated catheter, (E2) antibacterial catheter, (F2) PDMS–HNT–PEO–nitrofurantoin.
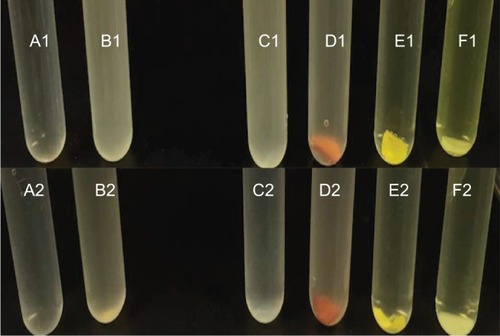
Figure 12 A graphic representation of untreated and treated PDMS surfaces and the biological effects in vitro.
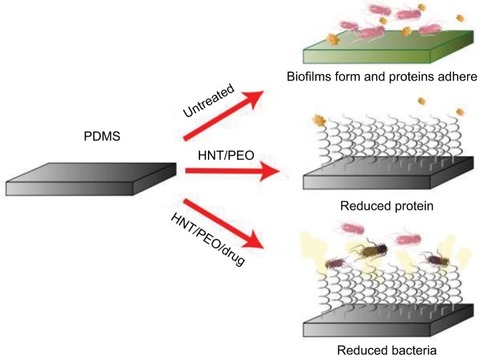
Table 3 Mueller–Hinton agar ZOI results for commercial catheters and PDMS–HNT–PEO–Nitro at 24 hrs
Table 4 Mueller–Hinton nutrient broth absorbance results for commercial catheters and PDMS–HNT–PEO–Nitro at 24 hours
Discussion
Catheter-associated urinary tract infection is among the more common health care-associated infections associated with PDMS-based medical devices and causes a major economic burden for patients and hospitals.Citation1,Citation2 PDMS is widely used in a variety of medical devices due to its biocompatibility, low toxicity, optical transparency, elastomeric properties, ease of fabrication, and low manufacturing costs.Citation28,Citation46 PDMS has proven to be a valuable biomaterial, but its hydrophobicity limits its potential for use in biomedical applications, and untreated PDMS can lead to protein adsorption on its surface, leading to bacterial adhesion.Citation1–Citation3,Citation28,Citation49,Citation50 Bacterial adherence and subsequent proliferation are an early step in biofilm formation, increased bacterial proliferation, colony formation, and subsequent bacterial encapsulation within a biofilm.Citation1,Citation9,Citation50,Citation51 The biofilm provides protection for resident bacteria from various environment agents, increases bacterial pathogenicity, and is directly responsible for a host of periprosthetic infectionsCitation9,Citation11 and virtually all urinary tract infections.Citation12 For the patient, this can cause additional complications including longer hospital stays, additional surgeries, increased medical costs, and perhaps death.Citation6–Citation8
Surface modification of PDMS has included both physical and chemical methods designed to render the PDMS surface hydrophilic; however, most research has been directed toward microfluidic or biosensor applications.Citation48,Citation51,Citation52 The fabrication of antifouling PDMS surfaces was done typically using hydrophobic or electrostatic interactions and surface activation by the physical treatment of oxygen plasma, ozone, or UV light.Citation26,Citation27,Citation48 Other studies have used chemical methods to form an antimicrobial surface including antibiotic and silver absorption,Citation50,Citation53 antibacterial coatings,Citation54 peptide coatings,Citation55 and copper impregnation.Citation18 Most of these surface modification methods fail to completely inhibit bacterial growth due to their inability to provide a sustained antibacterial effect.Citation56 This is typically due to the release of antimicrobials with short half-lives and antibiotic release that is less than maximal, or the antibiotics quickly degrade in the implant microenvironment. The rapid (and often excessive) release of antimicrobials also raises a major health concern by assisting in the growth and emergence of antibiotic-/fungal-resistant microbial strains.Citation18 There remains a critical need for a surface modification technique for invasive medical devices which creates a surface that is antifouling and possesses a sustained antimicrobial capability.Citation48,Citation56,Citation57
Toward this end, we fabricated a nanocomposite using the solvent swelling/deswelling method, a dipping strategy that uses polymers in water–solvent–antimicrobial agent mixtures, and bulk modification methods. Through these techniques, an antimicrobial agent and antibiofouling agents were incorporated into the PDMS/HNT matrix. Modified and unmodified PDMS surfaces showed gradual changes in surface roughness with increases in PEO and HNT contents. PDMS–HNT composites also displayed protein-repelling properties. Functionalizing silicone with PEO is a validated method for imparting a hydrophilic domain.Citation58 The use of the b-polymer (PDMS–b–PEO 600 MW) may have allowed the PEO chains to accumulate on the composite’s outermost surface. PEO chains are easily hydrated, and the rubber–water interfacial interactions may have been altered during processing.Citation58,Citation59 Covalent grafting of PEO to silicone surfaces has been shown to provide a long-term chemical stability without altering the bulk properties of the substrate.Citation60,Citation61
Water contact angle measurements confirmed the hydrophilic properties of the PDMS–b–PEO coatings on both PEO–PDMS and PDMS–10% HNT versions. Similar patterns of wettability were observed for both antimicrobial PEO-PDMS treated and untreated PEO–PDMS versions. The results also showed that HNT addition increased drug load during solvent swelling without reducing material strength properties. This finding agrees with other studies that have shown that the addition of HNTs to many polymers measurably improves their material properties. Improved fracture behavior, yield, and tensile strength of epoxy composites were reported, and these improvements were with the dissipation of the impact energy due to the embedded HNTs.Citation62,Citation63 Similar results were obtained on composites of HNTs with styrene–butadiene rubber.Citation64 The addition of 4% HNTs increased the tensile strength of HNT–gelatin composites by 50%, while the elastic modulus increased threefold.Citation65 A significant improvement in the thermal, tensile, and adhesive properties of poly(methyl methacrylate) bone cements was achieved after HNT addition, and the addition of 10% HNT by weight effectively reduced polymerization temperature to 40°C, thus reducing the potential of thermal necrosis for surrounding bone tissue.Citation39
We compared the bacterial growth inhibiting properties of our composites with standard, commercial-grade PDMS catheters. Silver-containing commercial-grade catheters showed little ability to inhibit the growth of E. coli and S. aureus, based on the results from the growth inhibition studies. In comparison, our method showed to be effective at inhibiting bacterial growth against both bacterial forms.
HNTs were added to PDMS by simple mixing, PEO and drugs were added using solvent swelling methods, and the resulting nanocomposite produced a material with antimicrobial or antibiofouling properties (Figure 13). The advantages over existing coating systems are the amalgamation of the materials infused and the effects associated, which include antibacterial effects, antiprotein biofouling, and hydrophilic properties. Commercial antibacterial materials have been previously studied with various microorganisms. The mean ZOIs reported for silver- and iodine-impregnated materials against S. aureus and E. coli are comparable to PDMS–HNT composites and hold potential in antimicrobial applications.Citation66 The outer PEO–drug layer and drug-infused PDMS–HNT network may offer a new strategy for the prevention of bacterial adherence on catheters, offering HNT/rubber nanocomposites that will offer a safe, long-lasting, and anti-infective implantable medical device. In addition, the PDMS–b–PEO hydrophilic nanocoatings may limit friction and prevent inflammation associated with medical device insertion and use. Lengthier inhibition and surface biofilm studies, plus additional in vivo work, are still needed to fully validate these composites. Additional “green” polymer processing techniques may be explored with HNT/polymers, such as supercritical carbon dioxide. Processing polymer that melts with supercritical carbon dioxide is a common method, which may act as an ecofriendly processing alternative with controllable features, such as porosity.Citation69,Citation70 Overall, this study demonstrated some of the potential medical applications and uses of HNTs as nano-additives for polymer enhancement. This type of PDMS composite may offer potential for the prevention of institutional-acquired (hospital or nursing home) infections. Furthermore, this type of nanocomposite polymer coating can be applied to existing PDMS catheter surfaces with customized thicknesses or drug formulations for a diverse set of applications.
Acknowledgments
The authors would like to thank the National Aeronautics and Space Administration/Louisiana Space Consortium (Mills), the Louisiana Governor’s Biotechnology Initiative (Mills), the Louisiana Biomedical Research Network (Girono), and the Louisiana Board of Regents OPT-IN program (Mills) for funding this project.
Disclosure
The authors report no conflicts of interest in this work.
References
- ParsekMRSinghPKBacterial biofilms: an emerging link to disease pathogenesisAnnu Rev Microbiol20035767770114527295
- ImamuraYChandraJMukherjeePKFusarium and Candida albicans biofilms of soft contact lenses: model development, influence of lens type, and susceptibility to lens care solutionsAntimicrob Agents Chemother20085217118217999966
- MarshPDMoterADevineDADental plaque biofilms: communities, conflict and controlPeriodontol 2000201155163521134226
- DarouicheROTreatment of infections associated with surgical implantsN Engl J Med20043501422142915070792
- PulidoLGhanemEJoshiAPurtillJJParviziJPeriprosthetic joint infection: the incidence, timing, and predisposing factorsClin Orthop Relat Res20084661710171518421542
- MagillSSHellingerWCohenJPrevalence of healthcare-associated infections in acute care facilitiesInfect Control Hosp Epidemiol20123328329122314066
- ScottRDThe Direct Medical Costs of Healthcare-Associated Infections in U.S. Hospitals and the Benefits of PreventionAtlanta, GADivision of Healthcare Quality Promotion National Center for Preparedness, Detection, and Control of Infectious2009CS200891-A
- PercivalSLSulemanLVuottoCDonelliGHealthcare-associated infections, medical devices and biofilms: risk, tolerance and controlJ Med Microbiol20156432333425670813
- DonlanMBiofilm formation: a clinically relevant microbiological processClin Infect Dis2001331387139211565080
- VasilevKCookJGriesserHJAntibacterial surfaces for biomedical devicesExpert Rev Med Devices2009655356719751126
- PavithraDDobleMBiofilm formation, bacterial adhesion and host response on polymeric implants – issues and preventionBiomed Mater2008303400318689922
- BehzadiPBehzadiEYazdanbodHAghapourRAkbari CheshmehMSalehian OrmanDA survey on urinary tract infections associated with the three most common uropathogenic bacteriaMaedica (Buchar)2010511111521977133
- SiddiqDMDarouicheRONew strategies to prevent catheter-associated urinary tract infectionsNat Rev Urol2012930531422508462
- McConnellSAGubbinsPOAnaissieEJDo antimicrobial-impregnated central venous catheters prevent catheter-related bloodstream infection?Clin Infect Dis200337657212830410
- HockenhullJCDwanKMSmithGWThe clinical effectiveness of central venous catheters treated with anti-infective agents in preventing catheter-related bloodstream infections: a systematic reviewCrit Care Med20093770271219114884
- HoffmanLRD’ArgenioDAMacCossMJZhangZJonesRAMillerSIAminoglycoside antibiotics induce bacterial biofilm formationNature20054361171117516121184
- Carmona-RibeiroAMBarbassaLde MeloLDAntimicrobial Biomimetics. Biomimetic Based ApplicationsCavrakMRijeka, CroatiaInTech2011
- McLeanRHussainAASayerMVincentPJHughesDJSmithTJAntibacterial activity of multilayer silver-copper surface films on catheter materialCan J Microbiol1993398958998242490
- RaiMYadavAGadeASilver nanoparticles as a new generation of antimicrobialsBiotechnol Adv200927768318854209
- AhamedMARAzarudeenRSKaniNMAntimicrobial applications of transition metal complexes of benzothiazole based terpolymer: synthesis, characterization, and effect on bacterial and fungal strainsBioinorg Chem Appl2014201416
- TreterJMacedoAJCatheters: a suitable surface for biofilm formationMéndez-VilasAScience Against Microbial Pathogens: Communicating Current Research and Technological AdvancesBadajoz, SpainFormatex Research Centre2011835842
- MackDDaviesAPHarrisLGChapter 2 Staphylococcus epidermidis in biomaterial-associated infectionsMoriartyFZaatSAJBusscherHJBiomaterials Associated Infection: Immunological Aspects 25 and Antimicrobial StrategiesNew YorkSpringer Science + Business Media2013
- SrivastavaAYadavTSharmaSNayakAKumariAAMishraNPolymers in drug deliveryJ Biosci Med201646984
- ShaikMRKorsapatiMPanatiDPolymers in controlled drug delivery systemsInt J Pharm Sci20122112116
- MuhkerjeeNMittelmannMWAntimicrobial coatings and other surface modifications for infection preventionGlockerDARanadeSVMedical Coatings and Deposition TechnologiesBeverly, MAScrivener Publishing LLC201629
- MashakARahimiASilicone polymers in controlled drug delivery systems: a reviewIran Polym J200918279295
- ZhangHChaioMAnti-fouling coatings of poly(dimethylsiloxane) devices for biological and biomedical applicationsJ Med Biol Eng20153514315525960703
- AbbasiFMirzadehHKatbabAAModification of polysiloxane polymers for biomedical applications: a reviewPolym Int20015012791287
- AbbasiFMirzadehHSimjooMHydrophilic interpenetrating polymer networks of poly(dimethyl siloxane) (PDMS) as biomaterial for cochlear implantsJ Biomater Sci Polym Ed20061734135516689019
- WongIHoCMSurface molecular property modifications for poly(dimethylsiloxane) (PDMS) based microfluidic devicesMicrofluid Nanofluidics2009729130620357909
- NuriogluAGCatarinaAEstevesCde WithGNon-toxic, non-biocide-release antifouling coatings based on molecular structure design for marine applicationsJ Mater Chem B2015365476570
- ZhouJKhodakovDAEllisAVVoelckerNHSurface modification for PDMS-based microfluidic devicesElectrophoresis2012338910422128067
- TuQWangJCZhangYSurface modification of poly(dimethylsiloxane) and its applications in microfluidics-based biological analysisRev Anal Chem201231177192
- ZhouJEllisAVVoelckerNHRecent developments in PDMS surface modification for microfluidic devicesElectrophoresis20103121620039289
- LvovYMShchukinDGMöhwaldHPriceRRHalloysite clay nanotubes for controlled release of protective agentsACS Nano2008281482019206476
- VergaroVAbdullayevELvovYMCytocompatibility and uptake of halloysite clay nanotubesBiomacromolecules20101182082620170093
- ShchukinDGSukhorukovGBPriceRRLvovYMHalloysite nanotubes as biomimetic nanoreactorsSmall2005151051317193477
- VeerabadranNGNanoengineered Templates for Controlled Delivery of Bioactive CompoundsRuston, LALouisiana Tech University2008
- WeiWAbdllayevEHollisterAMillsDLvovYMClay nanotube/poly(methyl methacrylate) bone cement composites with sustained antibiotic releaseMacromol Mater Eng2012297645653
- AbdullayevEPriceRShchukinDLvovYHalloysite tubes as nanocontainers for anticorrosion coating with benzotriazoleAppl Mater Interfaces2009114371443
- AbdllayevELvovYMFunctional polymer clay nanotube composites with sustained release of chemical agentsProg Polym Sci20133816901719
- SunLBoyerCGrimesRMillsDKDrug coated clay nanoparticles for delivery of chemotherapeuticsCurr Nanosci201612207214
- ZhouWYGuoBLiuMLiaoRRabieABJiaDPoly(vinyl alcohol)/halloysite nanotubes bionanocomposite films: properties and in vitro osteoblasts and fibroblasts responseJ Biomed Mater Res A2010931574158720014291
- KarnikSHinesKMillsDKNanoenhanced hydrogel system with sustained release capabilitiesJ Biomed Mater Res A20141032416242625424733
- MillsDKBiocompatibility of halloysite clay nanotubes in a rat dermal model (87.4)FASEB J2014281 supple
- JeonSILeeJHAndradeJDDeGennesPGProtein–surface interactions in the presence of polyethylene oxideJ Colloid Interface Sci1991142149158
- DhruvHDControlling Nonspecific Adsorption of Proteins at Bio-Interfaces for Biosensor and Biomedical Applications [all graduate theses and dissertations]2009 Paper 276. Available from: http://digitalcommons.usu.edu/etd/276Accessed October 30, 2017
- SlepickaPKasalkovaNSSiegelJKolskaZBacakovaLSvorcikVNano-structured and functionalized surfaces for cytocompatibility improvement and bactericidal actionBiotech Adv20153311201129
- MaitzMFApplications of synthetic polymers in clinical medicineBiosurf Biotribol201513161176
- McConnellSAGubbinsPOAnaissieEJDo antimicrobial-impregnated central venous catheters prevent catheter-related bloodstream infection?Clin Infect Dis200337657212830410
- WuZHjortKSurface modification of PDMS by gradient-induced migration of embedded PluronicLab Chip200991500150319458853
- WuMHUrbanJPCuiZCuiZFDevelopment of PDMS microbioreactor with well-defined and homogenous culture environment for chondrocyte 3-D cultureBiomed Microdevices2006833134016917663
- KnetschMLWKooleLHNew strategies in the development of antimicrobial coatings: the example of increasing usage of silver and silver nanoparticlesPolymers20113340366
- HockenhullJCDwanKMSmithGWThe clinical effectiveness of central venous catheters treated with anti-infective agents in preventing catheter-related bloodstream infections: a systematic reviewCrit Care Med20093770271219114884
- LimKChuaRRBowHTambyahPAHadinotoKLeongSSDevelopment of a catheter functionalized by a polydopamine peptide coating with antimicrobial and antibiofilm propertiesActa Biomater20151512713825541344
- Carmona-RibeiroAMBarbassaLde MeloLDAntimicrobial Biomimetics. Biomimetic Based ApplicationsCavrakM2001 Available from: http://www.intechopen.com/books/biomimetic-based-applications/antimicrobial-biomimeticsAccessed October 30, 2017
- AllahverdiyevAMKonKVAbamorESBagirovaMRafailovichMCoping with antibiotic resistance: combining nanoparticles with antibiotics and other antimicrobial agentsExpert Rev Anti Infect Ther201191035105222029522
- ChenHBrookMAChenYSheardownHSurface properties of PEO-silicone composites: reducing protein adsorptionJ Biomat Sci Polym Ed200516531548
- YaoMFangJHydrophilic PEO-PDMS for microfluidic applicationsJ Micromech Microeng2012226
- ZhaoXSuYLiYZhangRZhaoJJiangZEngineering amphiphilic membranes surfaces based on PEO and PDMS segments for improved antifouling performancesJ Memb Sci2014450111123
- BhattacharyaAMisraBNGrafting: a versatile means to modify polymers. Techniques, factors, and applicationsProg Polym Sci200429767814
- UyamaYKatoKIkadaYSurface modification of polymers by graftingAdv Polym Sci1998137339
- YeYChenHWuJYeLHigh impact strength epoxy nanocomposites with natural nanotubesPolymer20074864266433
- GuoBChenFLeiYLiuXWanJJiaDStyrene-butadiene rubber/halloysite nanotubes nanocomposites modified by sorbic acidAppl Surf Sci200925573297336
- VoonHCBhatREasaAMLiongMTKarimAAEffect of addition of halloysite nanoclay and SiO2 nanoparticles on barrier and mechanical properties of bovine gelatin filmsFood Bioprocess Technol2012517661774
- BasterziYErsozGSaracGSariADemirkanFIn-vitro comparison of antimicrobial efficacy of various wound dressing materialsWounds20102216517025901495
- HeWZhangYLiJA novel surface structure consisting of contact-active antibacterial upper-layer and antifouling sub-layer derived from gemini quaternary ammonium salt polyurethanesSci Rep201663214027561546
- ZhangYHeXDingMAntibacterial and biocompatible cross-linked waterborne polyurethanes containing gemini quaternary ammonium saltsBiomacromolecules Epub201812
- NalawadeSPPicchioniFJanssenLPBMSupercritical carbon dioxide as a green solvent for processing polymer melts: processing aspects and applicationsProg Polym Sci2006311943
- ZhanSPHuangXZhaoQCA High Efficiency PDMS-Based Stabilizer for Dispersion Polymerization of L-lactide in Supercritical Carbon DioxideJ Macromol Sci A20135010701074