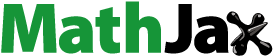
Abstract
In the last 10 years, biodegradable aliphatic polyesters, such as poly(lactic-co-glycolic acid) (PLGA), have attracted increasing attention for their use as scaffold materials in bone tissue engineering because their degradation products can be removed by natural metabolic pathways. However, one main concern with the use of these specific polymers is that their degradation products reduce local pH, which in turn induces an inflammatory reaction and damages bone cell health at the implant site. Thus, the objective of the present in vitro study was to investigate the degradation behavior of PLGA when added with dispersed titania nanoparticles. The results of this study provided the first evidence that the increased dispersion of nanophase titania in PLGA decreased the harmful change in pH normal for PLGA degradation. Moreover, previous studies have demonstrated that the increased dispersion of titania nanoparticles into PLGA significantly improved osteoblast (bone-forming cell) functions (such as adhesion, collagen synthesis, alkaline phosphatase activity, and calcium-containing minerals deposition). In this manner, nanophase titania–PLGA composites may be promising scaffold materials for more effective orthopedic tissue engineering applications.
Introduction
The use of biodegradable polyesters in orthopedic devices for proper fixation of long bone fractures was first clinically implemented in Finland in 1984 (CitationRokkanen et al 1985). It was demonstrated that the exact union of the fracture fixed by poly(lactic-co-glycolic acid) (PLGA) was 5 days faster than the fracture fixed by conventional metallic implants (CitationRokkanen et al 1985). Since the 1990s, the applications of poly(glycolic acid) (PGA), poly(lactic acid) (PLA), and their copolymers (PLGA) in bone tissue engineering have been investigated extensively (CitationGunatillake and Adhikari 2003). Importantly, these polymers (PLA, PGA, and PLGAs) have been approved by the US Food and Drug Administration (FDA) for certain human clinical applications because their degradation products are nontoxic, endogenous natural metabolites, and are eventually eliminated from the body in the form of carbon dioxide and water (CitationRatner 2004).
However, one main concern for the use of these polymers in bone tissue engineering is that their intermediate degradation products (specifically, lactic acid and/or glycolic acid) by non-enzymatic hydrolysis of ester bonds in their backbone reduces the local pH, which in turn induces an inflammatory reaction and damages bone cell health at the implant site. Moreover, the rapid drop of pH in vivo may accelerate the polymer’s degradation rate, thus, resulting in premature loss of mechanical properties before new bone formation occurs. Therefore, it is critical to decrease the adverse effects of PLGA degradation (specifically, pH drop) before this biomaterial can be used more effectively in orthopedic applications.
In this light, it has been reported that ceramic particles (such as Bioglass® and hydroxyapatite) used as additives to PLGA can provide a pH buffering effect at the polymer surface and, thus, avoid an unfavorable environment for new bone growth (CitationZhang and Ma 2000; CitationMaquet et al 2004). Specifically, such studies have shown that the pH of the media containing PLGA–hydroxyapatite foams dropped 73% less than the pH of the media containing control PLGA foams after 50 days of incubation. In addition, the pH of the media containing PLGA–Bioglass® foams dropped 3% less than the pH of the media containing control PLGA foams after 30 days of incubation. However, the influence of titania nanoparticle addition and its dispersion status on the degradation kinetics of PLGA have not been completed so far. Titania is a key orthopedic implant material since it is the oxide that readily forms on the widely implanted titanium. It is hypothesized that the addition of titania nanoparticles to PLGA may decrease the degradation rate of PLGA by interfering with water and acidic oligomer diffusion and, thus, decreasing harmful pH variations during degradation.
Therefore, to provide a complete story of the usefulness of nanophase titania/PLGA composites to improve orthopedic implant effectiveness, the objective of the present study was to investigate the degradation behavior of PLGA as influenced by the dispersion of titania nanoparticles.
Materials and methods
Materials Preparation
PLGA pellets (50/50 wt % poly(dl-lactic/glycolic acid); Polysciences, Warrington, PA, USA) were dissolved in chloroform while nanophase titania powder (Nanophase Technologies, Romeoville, IL, USA) was then added to the PLGA solution to give a 30/70 ceramic/polymer weight ratio. The composite mixture was then sonicated using a W-380 sonicator (Heat Systems - Ultrasonics, Farmingdale, NY, USA) with output power settings at 118.75 W, 166.25 W, 213.75 W, and 332.5 W (termed PTC25, PTC35, PTC45, and PTC70 respectively). After sonication, the suspension was cast into a Teflon petri dish, evaporated in air at room temperature for 24 hours and dried in an air vacuum chamber at room temperature for 48 hours. The schematic procedure for nanophase titania–PLGA composite fabrication is shown in .
Pure PLGA and nanophase titania compacts were used as controls. Nanophase titania compacts were prepared by dry pressing titania powders in a tool-steel die via a uniaxial pressing cycle from 0.6 to 3 GPa over a 10-minute period. The green (unsintered) titania compacts were then heated in air at a rate of 10°C/minute from room temperature to a final temperature of 600°C, sintered at 600°C for 2 hours, and were cooled down at the same rate as the heating rate. These compacts were termed sintered titania compacts (TCS).
Material characterization
Surface topographies of the nanophase titania/PLGA composites were characterized according to standard scanning electron microscopy techniques using a JEOL JSM-840 Scanning Electron Microscope (SEM) at a 5 kV accelerating voltage. Substrates were sputter-coated with a thin layer of gold-palladium using a Hummer I Sputter Coater (Technics, Alexandria, VA, USA) in a 100-millitorr vacuum argon environment for 3 minutes with 0.01 Amp of current. SEM images taken at 15 kX magnification were used to determine differences in topography and titania surface coverage of the nanophase titania–PLGA composites.
Degradation experiments
For degradation experiments, 12 specimens with dimensions of 10 mm × 10 mm × 3 mm were used for each composite and control sample. These specimens were divided into 3 sets according to incubation times. Initial dry substrates of interest were weighed (W0) and sterilized. Then, all the substrates were immersed into phosphate buffered saline (PBS; a solution containing 8 g NaCl, 0.2 g KCl, 1.5 g Na2HPO4, and 0.2 g KH2PO4 in 1000 mL deionized water adjusted to a pH of 7.4; all chemicals from Sigma) and were incubated under standard cell culture conditions, that is, a 37°C, 5% CO2/95% air environment. Blank PBS, without substrates, was used as a reference. After 21, 28, and 35 days, specimens were removed from PBS, abundantly rinsed with deionized water to remove the soluble inorganic salt, and dried in an air vacuum chamber at room temperature for 48 hours to reach constant mass. At each time point, samples were weighed (Wt) and the percentage of weight loss (%WL) with respect to incubation time was calculated according to the following equation. In the results, the %WL was normalized to the amount of PLGA in the composites since titania does not degrade.
The pH of the supernatant buffer was monitored three times a week during the experiments using a Corning 430 pH meter (Corning Incorporated, Acton, MA, USA).
Statistical analysis
Numerical data were analyzed using standard analysis of variance (ANOVA) techniques followed by a global F test and a Student t test; statistical significance was considered at p<0.05.
Results
Material characterization
Titania particles of different agglomeration sizes were visible on the surface of the composites, as shown in . Scanning electron micrographs suggested that the distribution of ceramic particles was different on the surface of the composites depending on the sonication power utilized; specifically, there were more titania particles on the surface of each composite after sonication with higher power. Finer titania particles were also observed on the surface with increasing sonication powers. That is, larger ceramic agglomerations tended to break into smaller particles in the polymer solution after higher powers of sonication. Because of this, the amount of surface area occupied by titania increased on the surface of the composites with higher sonication powers. Specifically, 10.6%, 10.2%, and 10.1% compared with 5.7% of the surface area occupied was titania on the PTC70, PTC45, PTC30, and PTC25 composites, respectively. At higher sonication powers, titania particles became smaller and were more evenly dispersed in the PLGA matrix. However, there were no significant differences in terms of titania surface coverage for PTC45 and PTC70 compared with PTC35.
Figure 2 Representative SEM micrographs of nanophase titania–PLGA composites: PTC25, PTC35, PTC45, and PTC70; and control materials: PLGA and TCS. Original magnification: 15 kX; magnification bars at the bottom-right corner: 1 μm.
Abbreviations: PLGA, poly(lactic-co-glycolic acid); TCS, sintered titania compacts.
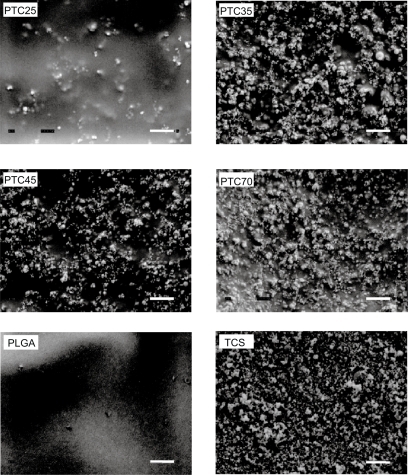
Decreased degradation for nanophase titania–PLGA composites
Results of the degradation study showed that the weight loss percentage of pure PLGA was the greatest among all the substrates tested after respective days (). As expected, no weight loss was observed on titania compacts. Specifically, the weight loss of PTC35, PTC45, and PTC70 was approximately 20% less than pure PLGA at 21 days of incubation; 30% less than pure PLGA at 28 days of incubation; and 50% less than pure PLGA after 35 days of incubation. Among all the composites, the weight loss of PTC25 was greater than the others at 35 days of incubation. This indicated that the dispersion status of nanophase titania in PLGA played an important role in decreasing the degradation behavior of these nanocomposite.
Figure 3 Percentage weight loss for PTC25, PTC35, PTC45, PTC70, PLGA, and TCS incubated in PBS under standard incubation conditions. Values are mean ± SEM; n = 3; *p<0.05 compared with PLGA at respective days; #p<0.05 compared to all the composites at respective days; **p<0.05 compared with PTC25 at respective days; +p<0.05 compared with respective materials at earlier days.
Abbreviations: PLGA, poly(lactic-co-glycolic acid); TCS, sintered titania compacts.
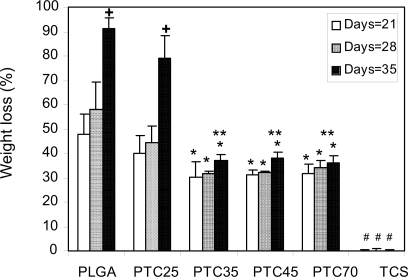
Moreover, the buffering effect of titania particles towards PLGA weight loss was more significant after longer time periods of incubation; this correlated to the pH buffering effect of titania particles. Specifically, the pH drop was less than 16% for all the composites during the first 21 days of incubation while it was 19% for pure PLGA (). During 22 to 35 days of incubation, the pH drop for all the composites and PLGA was faster than the first 3 weeks of incubation. Specifically, the pH drop was 70% for pure PLGA while it was 68% for PTC25, 56% for PTC35, and only 43% for PTC70 after 35 days of incubation.
Discussion and conclusions
The PLGA degradation process involves three steps that begin at the outer perimeter of the scaffolds and moves gradually into the interior, followed by catastrophic disintegration (CitationWise 2000). In step 1, water diffuses into the polymer chain and hydrolytic random chain scission of ester bonds begins. Since it undergoes hydrolytic cleavage, PLGA has more predictable degradation rates in vivo than polymers whose degradation is mediated predominantly by enzymes; this is because the levels of enzymatic activity may vary widely not only among different patients but also among different implantation sites. But the availability of water is virtually constant in all soft/hard tissues and varies little from patient to patient. In step 2, the molecular weight decreases and low-molecular-weight oligomers in the inner part of the matrix begin to diffuse out of the thinning outer layer. At this stage, an acidic environment is formed. When the molecular weight of these oligomers is low enough to allow solubilization in the medium, weight loss begins. In the final step 3, a polymer shell remains after the oligomers solubilize and slow degradation of the shell takes place. Degradation of PLGA demonstrates random scission mode under normal conditions (ie, in water or phosphate buffer medium of pH 7.4 at 37°C), while unzipping mode (chain-end scission) under harsh conditions (such as high acidity, high temperature, or high energy radiation) (CitationShih 1995).
This study provided the first evidence that nanophase titania dispersed in PLGA can improve the structural stability, buffer the harmful pH variations, and decrease the weight loss of the scaffolds. The possible reasons are that titania nanoparticles dispersed in PLGA may hinder water diffusion into the polymer chain and, thus, slow down hydrolytic cleavage; moreover, titania nanoparticles may interfere with the diffusion of intermediate degradation products (oligomers) out of the polymer, thus, slowing down the pH drop. The buffering effect of titania particles on pH was more significant at the later stage of the degradation, that is, after 21 days of incubation. At the early stage of degradation, the pH reduction might be attributed to the residual monomers and oligomers in the original PLGA. The significant pH drop started when the molecular chain was small enough to be able to diffuse out of the scaffold into the PBS solution. Data demonstrated that the dispersion of nanophase titania in PLGA decreased the degradation rate of PLGA which may be favorable to new bone growth.
Moreover, previous studies have demonstrated that the increased dispersion of titania nanoparticles into PLGA significantly enhanced osteoblast (bone-forming cell) functions (such as adhesion, collagen synthesis, alkaline phosphatase activity, and calcium-containing minerals deposition) (CitationLiu et al 2005a, Citationb). Mechanical advantages of combining nanophase titania into PLGA have also been reported (CitationMcManus et al 2005). Specifically, the bending modulus of composites of PLA with 50 wt. % nanophase (<100 nm) titania was significantly greater than respective composite formulations with conventional coarser grained titania. That is, compared to a bending modulus of 60 ± 3 MPa for plain PLA and 870 ± 30 MPa for conventional titania/PLA composites, the bending modulus of nanophase titania–PLA composites was 1960 ± 250 MPa, which was in the same order of magnitude as trabecular bone. In this manner, nanophase titania/PLGA composites may be promising scaffold materials for more effective orthopedic tissue engineering applications.
Acknowledgement
The authors would like to thank the National Science Foundation for funding.
References
- GunatillakePAAdhikariR2003Biodegradable synthetic polymers for tissue engineeringEur Cell Mater511614562275
- LiuHSlamovichEBWebsterTJ2005aImproved dispersion of nanophase titania in PLGA enhances osteoblast adhesionCeramic Transactions, Ceramic Nanomaterials and Nanotechnology III - Proceedings of the 106th Annual Meeting of the American Ceramic Society15924755
- LiuHSlamovichEBWebsterTJ2005bIncreased osteoblast functions on nanophase titania dispersed in poly-lactic-co-glycolic acid compositesNanotechnology16S6018
- MaquetVBoccacciniARPravataL2004Porous poly(alpha-hydroxyacid)/Bioglass® composite scaffolds for bone tissue engineering. I: preparation and in vitro characterizationBiomaterials2541859415046908
- McManusAJDoremusRHSiegelRW2005Evaluation of cytocompatibility and bending modulus of nanoceramic/polymer compositesJ Biomed Mater Res72A98106
- RatnerBD2004Biomaterials science: an introduction to materials in medicineBostonElsevierAcademic Press677911526
- RokkanenPBostmanOVainionpaaS1985Biodegradable implants in fracture fixation: early results of treatment of fractures of the ankleLancet3258443142242861365
- ShihC1995A graphical method for the determination of the mode of hydrolysis of biodegradable polymersPharm Res12122036608786985
- WiseDL2000Biomaterials and Bioengineering HandbookNew YorkMarcel Dekker14155
- ZhangRMaPX2000Degradation behavior of porous poly (α-hydroxy acids)/hydroxyapatite composite scaffoldsPolymer Prepr4116189