CRISPR: Clustered regularly interspaced short palindromic repeat.
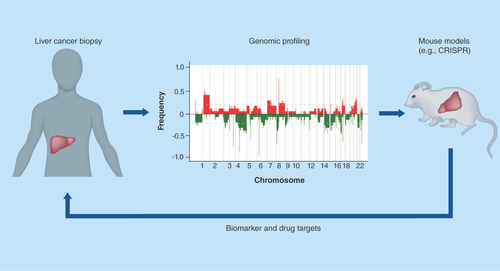
“The CRISPR-Cas9 system will allow us to rapidly develop models to validate numerous candidate genes uncovered by functional genomics, which ultimately allows us to address the unmet clinical need.”
Liver cancer is a highly heterogeneous genetic disease, with tumor cells frequently harboring a combination of numerous point mutations, translocations and chromosome gains and losses [Citation1,Citation2]. Likewise, the clinical presentation of hepatocellular carcinoma (HCC), a major histological type of primary liver cancer, is also heterogeneous. Its diagnostic, prognostic and treatment assessment is complicated by both tumor biology and compromised liver function due to underlying chronic inflammatory liver diseases such as fibrosis and cirrhosis [Citation3]. Different etiological factors such as viral hepatitis, chemical carcinogens, excess alcohol intake, obesity and cigarette smoking are major contributors of chronic liver disease and liver cancer heterogeneity. This feature poses an enormous challenge to liver cancer management [Citation4].
“Different etiological factors such as viral hepatitis, chemical carcinogens, excess alcohol intake, obesity and cigarette smoking are major contributors of chronic liver disease and liver cancer heterogeneity.”
Precision cancer models needed
To understand the effects of various cancer genes, precise models that incorporate both genomic changes in tumor cells and appropriate hepatic milieu are needed. Recent genomic profiling studies have allowed the identification of many candidate driver genes for HCC. However, considerable genomic variation bottlenecks mechanistic and therapeutic research. There is an enormous challenge to effectively rank, triage and evaluate all of the candidate driver genes as drug targets. While mouse tumor models are powerful tools to study cancer biology and to facilitate bench-to-bedside research, unlike their human counterparts, mouse livers are highly sensitive to chemicals or gene-targeting-induced neoplastic transformation. There are considerable differences between humans and rodents when comparing liver carcinogenesis [Citation5]. Consistently, while numerous genetically modified mouse models for HCC are currently available to study molecular mechanisms of hepatocarcinogenesis, genomic profiling studies have revealed that not all mouse models are relevant to human HCC [Citation6]. These studies highlight the importance to search for mouse models most clinically relevant to human liver cancer.
A critical question remains as to which mouse models are most relevant to human HCC. In this editorial, we will describe approaches to the construction and utility of various mouse models of human liver cancer, including carcinogen, germline, transposon and ex vivo models. The recent development of the CRISPR-Cas9 system, a powerful tool for efficient and precise genome editing, is transforming the process by which we construct mouse models. We will explain how techniques such as CRISPR-Cas9 have been used to efficiently generate mouse models of liver cancer. We will highlight the progress and challenges of such approaches, and how these models can be used to both understand progression of liver tumors and identify new strategies for cancer treatment. Establishing precision liver cancer mouse models will provide a rapid avenue for functional cancer genomics and pave the way for precision cancer medicine.
“…techniques such as CRISPR-Cas9 have been used to efficiently generate mouse models of liver cancer.”
Cancer cell-centric models
Investigating the function of cancer genes and identifying valid therapeutic targets in vivo represent a bottleneck because of the difficulty of constructing mouse models [Citation3]. Although many recurrent mutated genes have been identified in liver cancer by genome profiling, this knowledge has not fully transformed clinical care of patients. Current mouse models of liver cancer are available, but they each come with pros and cons ().
Chemically induced mouse models are useful to mimic the etiological role of carcinogens in human liver cancer. For example, diethylnitrosamine is a carcinogen that has been intraperitoneally injected into approximately 12-days-old mice (5 mg/kg) and the mice developed HCC around 44 weeks of age [Citation7]. However, the lack of control of genetic mutations limits the ability of models like this to fully recapitulate the genetics of human liver cancer.
Germline mouse models, including transgenic and knockout mice, have been widely used to study liver cancer. For example, mice harboring the tetracycline-inducible human MET oncogene developed liver cancer at 12 months [Citation8]. Yet while this method is ultimately effective, the cost and time required for generating traditional germline model remains major disadvantage to this approach.
To bypass the need to make germline models, transposon mouse models have been widely used to model genetic mutations in mouse livers [Citation9]. Hydrodynamic system can deliver sleeping beauty transposons to stably express activated oncogenes or to inhibit tumor suppressor genes by RNAi. Although this model has disadvantages such as random integration of transposons and overexpression of oncogenes above their endogenous levels, transposon mouse models can rapidly investigate combinations of genetic events in liver cancer.
To directly introduce mutations in liver progenitor cells, ex vivo models using mouse embryonic liver progenitor cells and transplantation have been devised [Citation10,Citation11]. This type of mouse model is based on retroviral infection of oncogenes and RNAi-mediated knockdown of tumor suppressor genes. Although ex vivo models require labor-intensive surgical transplantation of progenitor cells, they provide rapid and flexible methods (such as synthetic lethal RNAi screens) to study genetic interactions and to identify new drug target genes [Citation12]. Both ex vivo and transposon models are compatible with high throughput screens [Citation13,Citation14].
Recently, CRISPR models have transformed the construction of mouse models. The development of CRISPR-Cas9 genome editing technology [Citation15,Citation16] now provides fast-to-design and easy-to-use gene editing, allowing rapid construction of disease mouse models. In germline CRISPR models, Cas9, a single-guide RNA and template DNA for homologous recombination can be coinjected into zygotes to introduce precise cancer mutations. This method can generate mouse strains that allow germline transmission and breeding of modified alleles.
Alternatively, somatic delivery of CRISPR is an effective approach to directly mutate target genes. Somatic genome editing has several important features that can complement germline mouse models: bypass embryonic lethality of some complete gene knockouts; achieve liver-specific mutagenesis; rapid delivery into specific strains to investigate the effects of genetic background and etiological factors; potential for rapid screening of candidate genes in vivo.
Although CRISPR models have limitations such as mosaic mice in the germline model, off-target effect and low homologous recombination efficiency [Citation17], this technique is faster and can model multiple mutations in one step. This feature is a key to facilitate the establishment of precision models relevant to human liver cancer.
A need to incorporate altered liver milieu into precision models
Technologies such as CRISPR allow us to rapidly generate animal models to help efficiently address complex diseases like HCC. However, because both viral (such as hepatitis) and environmental factors (such as obesity, fatty liver disease and alcohol abuse) play essential roles in liver cancer etiology, ideal mouse models should consider incorporating both chronically altered liver milieu and somatically altered cancer genes that contribute to liver cancer.
More than 80% of HCC are associated with hepatitis B virus (HBV) and hepatitis C virus (HCV) infection [Citation7]. Several mouse models are available for studying the effects of chronic infection of HBV and HCV. For example, HBV-related transgenic animals have been generated to mimic human chronic HBV-related chronic liver diseases [Citation18]. Similarly, hepatitis B virus X transgenic mice develop liver tumors after 13–24 months [Citation19]. A humanized mouse model to model HCV infection has also been established [Citation20]. Abusive alcohol consumption is another leading cause of liver damage and a contributive factor for cirrhosis and liver cancer [Citation21]. Several mouse models of chronic and binge ethanol feeding are available [Citation21]. Besides hepatitis and alcohol, a connection between obesity and liver cancer has been uncovered in recent years [Citation22]. Another exciting study has linked gut microbiome, high fat diet and liver metabolism to HCC development in mice although its pathophysiological relevance of human HCC remains determined [Citation23]. It is conceivable that one can use CRISPR somatic delivery to directly engineer cancer mutations in the liver of mice with various different etiological backgrounds. This will provide a shortcut to incorporate genetic changes into viral/nonviral mouse models compared with laborious crossing of germline HCC mice with these mice. This approach can investigate a combined effect of genetic mutations and viral/nonviral factors to accurately model liver cancer cases found in HBV-, HCV- or obesity-endemic countries. Thus, genetic mouse models such as the CRISPR-Cas9 system will be invaluable for the study of hepatocarcinogenesis.
“We envision using multiple mouse models to study liver cancer rather than one-size-fits-all since liver cancer is genetically heterogeneous, lacking driver genes amenable to targeted therapy.”
Headed for the future
While many cancer types show decreased mortality rate in the USA between 1990 and 2009, the mortality of liver cancer has increased >40% in the same period [Citation24], the highest increase of any other type of cancer. Therefore, new drug target genes are urgently needed to explore new therapeutic approaches for HCC. A wealth of data from recent genomic profiling studies has been collected about putative biomarkers and therapeutic targets. However, although animal models exist for human liver cancer, the ability to develop biologically relevant precision mouse models quickly and efficiently has prevented study of these genes. The CRISPR-Cas9 system will allow us to rapidly develop models to validate numerous candidate genes uncovered by functional genomics [Citation25], which ultimately allows us to address the unmet clinical need ().
We envision using multiple mouse models to study liver cancer rather than one-size-fits-all since liver cancer is genetically heterogeneous, lacking driver genes amenable to targeted therapy [Citation2,Citation26]. To bridge the gap between human and mouse biology, humanized mouse models [Citation27] such as transplanting primary human hepatocytes or human solid tumor tissue (patient derived xenografts) into immunodeficient mice will be another useful approach for studying human liver tumor biology in vivo. These diverse approaches will allow us to develop therapeutic options better suited to the complex nature of the disease. We are heading in the right direction.
Table 1. A list of animal models useful for liver cancer.
Financial & competing interests disclosure
This work was supported by the intramural research program of the center for cancer research, the National Cancer Institute to XW Wang (Z01-BC010876) and by the National Cancer Institute to W Xue (5R00CA169512). The authors have no other relevant affiliations or financial involvement with any organization or entity with a financial interest in or financial conflict with the subject matter or materials discussed in the manuscript apart from those disclosed.
No writing assistance was utilized in the production of this manuscript.
Additional information
Funding
References
- Totoki Y , TatsunoK, CovingtonKRet al. Trans-ancestry mutational landscape of hepatocellular carcinoma genomes. Nat. Genet.46(12), 1267–1273 (2014).
- Zucman-Rossi J , VillanuevaA, NaultJC, LlovetJM. The genetic landscape and biomarkers of hepatocellular carcinoma. Gastroenterology doi:10.1053/j.gastro.2015.05.061 (2015) ( Epub ahead of print).
- Takai A , DangHT, WangXW. Identification of drivers from cancer genome diversity in hepatocellular carcinoma. Int. J. Mol. Sci.15(6), 11142–11160 (2014).
- Wang XW , ThorgeirssonSS. The biological and clinical challenge of liver cancer heterogeneity. Hepat. Oncol.1(4), 349–353 (2014).
- Grisham JW . Interspecies comparison of liver carcinogenesis: implications for cancer risk assessment. Carcinogenesis18(1), 59–81 (1997).
- Lee JS , ChuIS, MikaelyanAet al. Application of comparative functional genomics to identify best-fit mouse models to study human cancer. Nat. Genet.36(12), 1306–1311 (2004).
- Newell P , VillanuevaA, FriedmanSL, KoikeK, LlovetJM. Experimental models of hepatocellular carcinoma. J. Hepatol.48(5), 858–879 (2008).
- Wang R , FerrellLD, FaouziS, MaherJJ, BishopJM. Activation of the MET receptor by cell attachment induces and sustains hepatocellular carcinomas in transgenic mice. J. Cell. Biol.153(5), 1023–1034 (2001).
- Chen X , CalvisiDF. Hydrodynamic transfection for generation of novel mouse models for liver cancer research. Am. J. Pathol.184(4), 912–923 (2014).
- Xue W , ZenderL, MiethingCet al. Senescence and tumour clearance is triggered by p53 restoration in murine liver carcinomas. Nature445(7128), 656–660 (2007).
- Zender L , SpectorMS, XueWet al. Identification and validation of oncogenes in liver cancer using an integrative oncogenomic approach. Cell125(7), 1253–1267 (2006).
- Huang CH , LujambioA, ZuberJet al. CDK9-mediated transcription elongation is required for MYC addiction in hepatocellular carcinoma. Genes Dev.28(16), 1800–1814 (2014).
- Rudalska R , DauchD, LongerichTet al. In vivo RNAi screening identifies a mechanism of sorafenib resistance in liver cancer. Nat. Med.20(10), 1138–1146 (2014).
- Zender L , XueW, ZuberJet al. An oncogenomics-based in vivo RNAi screen identifies tumor suppressors in liver cancer. Cell135(5), 852–864 (2008).
- Doudna JA , CharpentierE. Genome editing. The new frontier of genome engineering with CRISPR-Cas9. Science346(6213), 1258096 (2014).
- Sander JD , JoungJK. Crispr-Cas systems for editing, regulating and targeting genomes. Nat. Biotechnol.32(4), 347–355 (2014).
- Sanchez-Rivera FJ , JacksT. Applications of the CRISPR-Cas9 system in cancer biology. Nat. Rev. Cancer15, 387–395 (2015).
- Chisari FV , PinkertCA, MilichDRet al. A transgenic mouse model of the chronic hepatitis B surface antigen carrier state. Science230(4730), 1157–1160 (1985).
- Koike K , MoriyaK, IinoSet al. High-level expression of hepatitis B virus HBx gene and hepatocarcinogenesis in transgenic mice. Hepatology19(4), 810–819 (1994).
- Bility MT , ZhangL, WashburnML, CurtisTA, KovalevGI, SuL. Generation of a humanized mouse model with both human immune system and liver cells to model hepatitis C virus infection and liver immunopathogenesis. Nat. Protoc.7(9), 1608–1617 (2012).
- Bertola A , MathewsS, KiSh, WangH, GaoB. Mouse model of chronic and binge ethanol feeding (the NIAAA model). Nat. Protoc.8(3), 627–637 (2013).
- Calle EE , RodriguezC, Walker-ThurmondK, ThunMJ. Overweight, obesity, and mortality from cancer in a prospectively studied cohort of US adults. N. Engl. J. Med.348(17), 1625–1638 (2003).
- Yoshimoto S , LooTM, AtarashiKet al. Obesity-induced gut microbial metabolite promotes liver cancer through senescence secretome. Nature499(7456), 97–101 (2013).
- Llovet JM , VillanuevaA, LachenmayerA, FinnRS. Advances in targeted therapies for hepatocellular carcinoma in the genomic era. Nat. Rev. Clin. Oncol.12(7), 408–424 (2015).
- Roessler S , LongEL, BudhuAet al. Integrative genomic identification of genes on 8p associated with hepatocellular carcinoma progression and patient survival. Gastroenterology142(4), 957–966 (2012).
- Villanueva A , Hernandez-GeaV, LlovetJM. Medical therapies for hepatocellular carcinoma: a critical view of the evidence. Nat. Rev. Gastroenterol. Hepatol.10(1), 34–42 (2013).
- Ito R , TakahashiT, KatanoI, ItoM. Current advances in humanized mouse models. Cell. Mol. Immunol.9(3), 208–214 (2012).
- Wang H , YangH, ShivalilaCSet al. One-step generation of mice carrying mutations in multiple genes by CRISPR/Cas-mediated genome engineering. Cell153(4), 910–918 (2013).
- Xue W , ChenS, YinHet al. CRISPR-mediated direct mutation of cancer genes in the mouse liver. Nature514(7522), 380–385 (2014).
- Li Y , ParkA, MouHet al. A versatile reporter system for CRISPR-mediated chromosomal rearrangements. Genome Biol.16(1), 111 (2015).
- Zhang S , LiL, KendrickSL, GerardRD, ZhuH. TALEN-mediated somatic mutagenesis in murine models of cancer. Cancer Res.74(18), 5311–5321 (2014).