Abstract
Extracellular vesicles (EVs) are nano-sized vesicle structures secreted from a variety of cells, which carry numerous biological macromolecules, participate in cell signal transduction and avoid immune system clearance. EVs have a plethora of specific signal recognition factors, and many studies have shown that they can play an important role in the precise treatment of tumors. This review aims to compile the applications of EVs as nanocarriers for antitumor drugs, gene drugs and other nanomaterials with anticancer capability. Additionally, we systematically summarize the preparation methodology and expound upon how to improve the drug loading and cancer-targeting capacity of EVs. We highlight that EV-based drug delivery has the potential to become the future of precise cancer treatment.
Background
As we all know, cancer has always been a public health problem of global concern [Citation1]. In the modern medicine era, researchers have studied and developed a plethora of methods for malignant tumor treatment, such as drugs, surgery, radiotherapy and immunotherapy [Citation2–4]. Of these, neoadjuvant chemotherapy strategies have received increasing attention and may become the most promising alternative to surgical procedures [Citation5]. However, pharmacotherapy has many drawbacks, including poor targeting, low stability, poor biocompatibility and facile degradation, which may lead to an unsatisfying treatment outcome and severe side effects. How to overcome these problems has become the focal point of current chemotherapeutic research.
The current commercial anticancer nanodrugs and nanovectors include three kinds: liposome, albumin nanoparticles and polymeric micelles. Besides albumin, ferritin, with its stable cage-like structure, is also intensively applied to encapsulate many drug molecules. Ferritin has an 8-nm diameter inner cavity and its outer 12-nm diameter is suitable for the enhanced permeation and retention effect in cancer treatment [Citation6]. Although providing some encouraging effects, the application of these nanodrugs and nanovectors faces the problem of physiological barriers. Under the circumstances, researchers focus on the topic of natural vectors. Red cells, immunological cells and stem cells have been processed into cell medicines for cancer treatment [Citation7]. These endogenous cells are applicable for the delivery of nanomedicines because they can help the latter to escape from the mononuclear phagocytic system (MPS) and other physiological barriers in vivo. Within the limits of current technology, the in vivo replication of these cells brings challenges and potential risks in clinical cancer treatment [Citation8]. The application of platelets and extracellular vesicles (EVs) may provide a breakthrough in this field. Similar to cells, platelets are membrane-based tiny bits of protoplasm and have also been used for drug delivery. Platelet membranes have CD44 and P-selectin receptors on their surface so that they can bind to circulating cancer cells, which makes platelets good candidates for anticancer drug delivery [Citation9]. Compared with platelets, EVs have much smaller size, which is beneficial for the delivery of anticancer cargo to cancer cells. In fact, EVs were verified to possess higher anticancer efficiency than ferritin when delivering the membrane protein (SIRPα) [Citation10].
EVs are naturally occurring nanoparticles and include exosomes, microvesicles and apoptotic bodies. Exosomes were first discovered in 1981, but for a long period they were treated as waste products of cells [Citation11]. With the deepening of research, exosomes have begun to receive widespread attention. Exosomes are so small that studying them is challenging and there are errors in the measurement at current levels of development. Nanoparticle tracking analysis is fast but is not suitable for detecting vesicles smaller than 50 nm, while flow cytometry can only detect vesicles larger than 300 nm. Dynamic light scattering can expand the detection range to 1–6000 nm; however, it will overestimate the contribution of larger vesicles and is sensitive to the density of the particle suspension, surface structures and the surrounding fluid. Identifying exosomes using electron microscopy and atomic force microscopy is time-consuming and expensive and requires a high level of device operation and sample preparing technology. The support film may be torn or damaged if the sample concentration is too high, leading to a failure in analyzing the exosome size and morphology using electron microscopy [Citation12]. The measurement methods cannot be unified, so it is impossible to clearly indicate a very accurate range of exosome sizes, thus the size of exosomes reported in the literature is inconsistent. Generally, exosomes are defined as bilayer membrane-like structures with a diameter of about 40–160 nm which are secreted by cells and function as transfer vehicles, carrying a variety of substances like proteins, nucleic acids and lipids [Citation13]. Microvesicles, which bud directly from the plasma membrane, can be distinguished from exosomes by their larger size (50–1000 nm). Apoptotic bodies, EVs of 500–5000 nm in diameter, are released by cells undergoing programmed cell death. In recent years several studies have demonstrated that EVs can load foreign materials, including all manner of drugs, not just natural substances [Citation14]. Compared with other drug-loading methods, EVs have several advantageous [Citation15]. First, EVs come from a wide range of sources and have small molecular weight; compared with liposomes, EVs have reduced toxicity, superior tissue tolerance and increased biocompatibility. Second, the lipid bilayer membrane structure of EVs can protect their contents from degradation, enhancing biological stability. Third, the specific receptors on the membrane surface of the EV can be exclusively recognized by the target cells, which reduces drug leakage and systemic toxicity compared with traditional administration methods. Fourth, EVs are small enough to pass through the blood–brain barrier, bringing a new therapeutic strategy to treat brain tumors. Lastly, EVs can circulate in the body and prolong the drug’s duration of action.
Sources of EVs
EVs can be sourced from a variety of organisms, including animals, plants and bacteria. In , we summarize some basic categories of the natural sources of EVs as drug vectors.
Table 1. The natural sources of extracellular vesicles as drug vectors.
Mammalian cell-derived EVs
In mammals, EVs can be extracted from almost any cells, such as tumor cells, B cells, T cells and mesenchymal stem cells [Citation17–19,Citation23,Citation30–33]. In 2016, researchers at Oxford University announced they had develpoed an innovative exosome-based product and founded Evox on the data. This exosome product can precisely deliver certain drugs to specific areas of the body and treat incurable diseases including brain diseases, autoimmune diseases and cancer [Citation34]. Similar progress in EV-based treatment is being made all over the world. The physicochemical properties and pharmacokinetics of EVs derived from different cell lines are similar, but the yields are quite different [Citation21]. Determining which cell line promises the best yield is the most important aspect in designing EV-based drug vectors. Takahashi et al. compared exosomes derived from B16BL6 murine melanoma cells, C2C12 murine myoblasts, NIH3T3 murine fibroblasts, MAEC murine aortic endothelial cells and RAW264.7 murine macrophage-like cells; the result showed that C2C12 and RAW264.7 cells produced more exosomes than the other types of cells [Citation21]. In fact, RAW264.7 is the most frequently used murine cell line source for EV nanovectors [Citation22]. Exosomes derived from proinflammatory M1-type RAW264.7 cells can provide a proinflammatory environment, which enhances the antitumor activity via the caspase-3-mediated pathway. The obtained M1 exosomes were used as paclitaxel (PTX) carriers and showed obviously higher anticancer efficiency than free PTX in breast cancer in vitro and in vivo [Citation22]. Similarly, human immune cells themselves have the potential to inhibit cancer progression; thus EVs derived from these immune cells also have a particular effect on tumor treatment, making the immune cell-derived EVs natural gene drugs and excellent nanovectors. For instance, human natural killer (NK) cell-derived EVs containing tumor suppressor miR-186 have been shown to inhibit neuroblastoma growth and immune escape mechanisms, indicating the potential application of NK cell-derived EVs in cancer immunotherapy [Citation16].
With the in-depth study of exosomal drug loading, exosomal biomimetic particles have shown promise and become a pre-eminent research direction. Exosomal biomimetic particles are a class of artificial lipid bilayer particles rich in specific protein molecules and of a size similar to exosomes [Citation35]. The surface structure of endogenous exosomes is complicated. When targeted drug delivery is performed, this feature may lead to off-target effects and unsatisfactory results [Citation36]. Exosomal bionic nanoparticles can simplify the surface structure of exosomes to achieve specific functions. Tian et al. developed murine breast cancer 4T1 cell-derived exosome-camouflaged porous silicon nanoparticles (E-MSNs) as a drug delivery system. ID@E-MSNs were obtained by loading the E-MSNs with indocyanine green and doxorubicin (Dox). With the help of exosome membrane, ID@E-MSNs showed synergistic effects of chemotherapy and photothermal therapy against breast cancer in 4T1 tumor-bearing BALB/c mice [Citation24]. In fact, researchers have verified that EVs derived from cancer cells have potential tropism to their parent cells, providing strategies for personalized chemotherapy and immunotherapy in all kinds of cancers [Citation20].
EVs from animal milk
Recently, EVs isolated from animal sources such as bovine, porcine and murine milk have been found to be valuable for human cancer treatment [Citation37]. These animal-derived EVs have multiple specific advantages, including physical and biological stability, tolerance and scalability of manufacturing process [Citation25]. Several berries have been found to inhibit tumor growth, with their extracts possessing an anticancer effect [Citation38]. Mechanistically, this effect has been linked to the diverse mixture of anthocyanidins present in these berries and their extracts. Based on this principle, researchers have developed a nanoformulation that contains anthos (aglycone anthocyanidins) and EVs harvested from raw bovine milk. Compared with the free anthos, the exosomal anthos nanoformulation showed significantly enhanced suppression activity against a lung cancer tumor xenograft in nude mice experiments [Citation39]. Loading curcumin into milk-derived EVs has also produced improved bioavailability and tumor suppression compared with the free curcumin [Citation40,Citation41]. To date, reports about the application of human milk-derived EVs in cancer treatment are lacking. Considering the large production of animal-derived EVs, they may be a rich resource for future drug development, but their safety needs to be verified.
EVs from plants
EVs secreted from plants may also be helpful in the treatment of malignancy. Plant-derived EVs currently used in cancer treatment are extracted from nontoxic edible plant cells such as ginger, grapes and lemons [Citation26,Citation27,Citation42,Citation43]. Ginger-derived nanolipids, modified with the targeting ligand folic acid and loaded with the anticancer drug Dox, have shown excellent histocompatibility and high inhibition efficiency in colon cancer [Citation27]. In addition to their drug-carrying ability, the plant-derived EVs themselves have been found to possess anticancer effects. One study showed that miR-159 richness in Arabidopsis thaliana and Glycine max-derived EVs was inversely related to the onset and progression of breast cancer [Citation44]. Lemon-derived EVs specifically stimulate TNF-related apoptosis, inducing ligand-mediated apoptotic cells to exert anticancer effects [Citation43]. More and more studies show that plant-derived EVs, as both carrier and therapeutic, display a gratifying effect in cancer treatment.
Bacteria-derived EVs
Studies on bacteria-derived EVs primarily focus on the field of immune defense, similar to the immunotherapy improvement in various tumor treatments [Citation45]. As most of the bacterial toxins are located in the outer wall of the cell, less toxic bacterial protoplasts are applied to extract EVs for targeted delivery systems [Citation28,Citation29].
Methods of cargo loading
EVs are membrane–scaffold-based structures similar to cell membranes and liposomes, thus the drug loading methods of cells and liposomes are usually applicable for EVs. Current research indicates there are two main methods for drug loading in EVs: direct drug loading and indirect drug loading (). The characteristics of these methods are compared in .
(A–E) Direct drug-carrying methods. (A) Coincubation. (B) Transfection. (C) Electroporation. (D) Saponins. (E) Ultrasound. (F & G) Indirect drug-carrying methods. (F) The lentivirus containing the target gene transfers the target gene into the cell and is released after being encapsulated by the EVs. (G) The chemotherapeutic drugs enter the cell through osmosis and are encapsulated by EVs and then released.
EV: Extracellular vesicle.
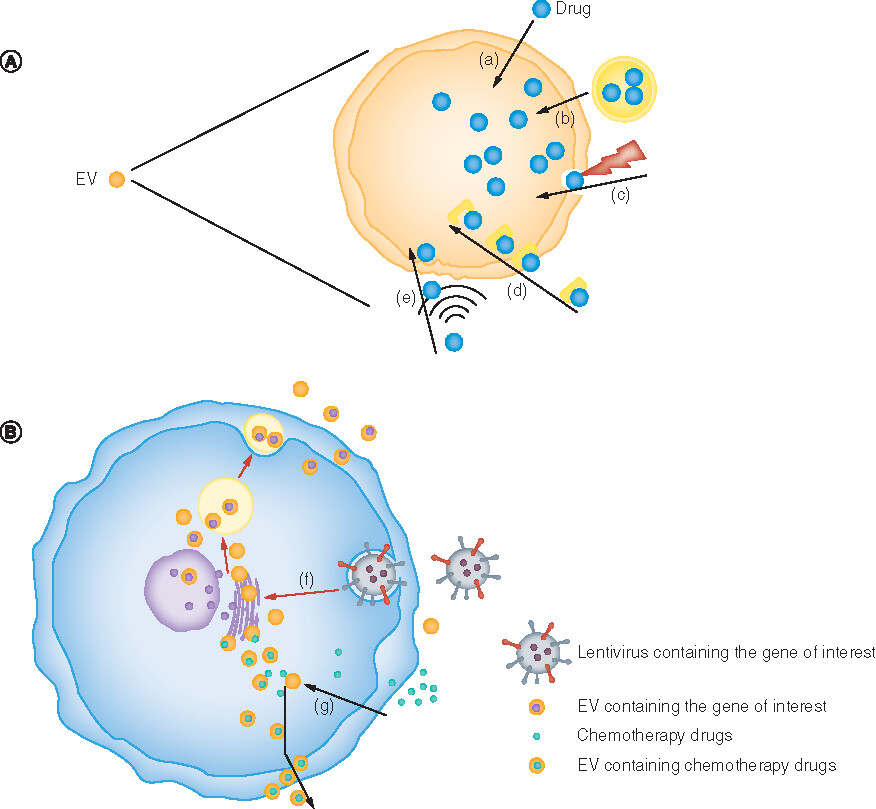
Table 2. Comparison of different loading methods.
Direct drug carrying
The direct drug loading methods involve loading a drug directly into an EV without passing through EV-derived cells. This method can ensure the specificity of drug loading and maximally reduce other interfering substances. The main current direct loading methods include coincubation, transfection, electroporation and ultrasonication [Citation46,Citation52,Citation53]. Coincubation involves cocultivating the drugs with EVs in an appropriate environment. The efficiency of coincubation may be related to the nature of the encapsulated drug; for instance, curcumin incubation takes only 5 min, while Dox requires overnight incubation [Citation54,Citation55]. This effect may be related to the good compatibility of curcumin and the exosomal membrane. Although this method relies on molecular motion, similar to the principle of compatibility, the transfer rate is relatively low [Citation50]. The transfection method, which uses chemical transfection reagents to load target drugs into EVs, can increase the efficiency and level of drug transfer [Citation48]. Electroporation is a physical method utilizing the formation of small pores in the bilayer membrane of EVs under transient high pressure, increasing the permeability of EVs and allowing drugs to enter the EVs through these pores [Citation52]. Different voltages are applied to encapsulate drugs depending on the source of the donor cells. Electroporation does not induce changes in exosomal size and morphology, but it causes damage to exosomal membranes [Citation56]. In addition, when EVs are loaded with siRNAs via electroporation, this may cause siRNA aggregation and subsequently reduce the loading efficiency [Citation49]. Therefore the efficiency of drug loading through electroporation may not be as high as it theoretically should be. Electroporation has generally been used in combination with other methods to raise drug encapsulation efficiency. The ultrasound method involves multiple ultrasonic treatments of a drug mixture with exosomes [Citation46]. Simply stated, the drugs are encapsulated into the EVs under the influence of sound waves on the exosomal membrane. Compared with electroporation and coincubation, the ultrasound method provides superior drug loading without affecting the content of EV lipids, which is beneficial for the diffusion of drugs [Citation46,Citation57]. Saponin is a surfactant molecule that can form a complex with cholesterol in the cell membrane structure and generate transient pores, thereby increasing the permeability of the membrane [Citation58,Citation59]. Saponin has been used to increase the affinity of macromolecular substances like proteins for the exosomal membrane. Haney et al. showed that when exosomes were permeabilized with saponin, the cargo loading efficiency significantly increased [Citation47]. Fuhrmann et al. verified that hydrophobic drugs will not change the structure and/or function of exosomes when loaded through active drug loading technologies, such as electroporation and coincubation [Citation56]. Other methods, like extrusion and freeze–thaw cycling, require high-standard operating instruments and can induce defects in the exosomal membrane, leading to unsatisfactory drug loading efficiency [Citation47]. Researchers have generally preferred to use these direct drug encapsulation methods in combination to avoid the shortcomings of a single method.
Indirect drug loading
The indirect drug loading methodology must encapsulate drugs to the EV-derived cells via intracellular synthesis processing, then collect the EVs containing the drugs of interest from the culture medium of the donor cells [Citation60,Citation61]. After that, the EVs must be further filtered to select those containing the drug of interest. For example, researchers incubated 1 μM Dox with pancreatic cancer cells, pancreatic stellate cells and macrophages for 48 h, then used differential ultracentrifugation to obtain EVs to explore the efficiency and effectiveness of the three kinds of cells loaded with Dox. The results indicated that pancreatic cancer cells showed the greatest efficiency in exosomal drug loading. However, Dox-loaded EVs (Exo-Dox) derived from macrophages has the greatest tumor cytotoxicity against the proliferation of pancreatic cancer cells, which may be related to the anticancer effect of EVs derived from macrophages [Citation62]. Although having shortcomings such as a long cycle and poor controllability, the indirect drug loading method is most commonly employed because of its high yield and technical simplicity. By transfecting sorted γδ T cells with LV-hsa-mir-138, Li et al. prepared miR-138-rich γδ T cell-derived EVs (γδ TDEs) and used them to treat oral squamous cell carcinoma [Citation51]. This transfection method can serve as a strategy to load gene drugs into EVs.
Given that the quantity of EVs secreted by natural cells falls far short of production requirements, researchers have tried to explore various ways to boost secretion amount without reducing quality. Fukuta et al. found that stimulating cells with a low-level electric treatment (with a voltage of 0.34 mA/cm2) for about 60 min can accelerate cell signal transduction and cell throughput, and promote the release of EVs [Citation63]. The quality of EVs exhibited no significant changes compared with the EVs secreted without electrical stimulation. In conclusion, electrical stimulation does not change the properties and/or function of EVs themselves, but accelerates the process of intracellular production and processing [Citation63]. As shown in , other methods that can promote EV production will be summarized in the latter part of this review.
Cargo type
Chemotherapeutic compounds
Current conventional chemotherapy drugs have poor targeting and severe side effects. Due to the existence of natural exosomes, there is potential for these EVs to be suitable candidates for improving the targeting of chemotherapeutic drugs. At present, the most widely studied drug for exosomal chemotherapy drugs is PTX. In clinical applications, PTX has poor water solubility and is often dissolved in Cremophor EL and ethanol to improve solubility. However, Cremophor EL itself can induce hypersensitivity reactions, hyperlipidemia and red blood cell aggregation, and may cause long-term, irreversible sensory neuropathy [Citation64]. Agrawal et al. encapsulated PTX into milk-derived exosomes and were able to achieve continuous PTX release for up to 48 h with ideal drug stability [Citation65]. After encapsulating PTX into exosomes, it could be administered orally and its systemic and immunogenic responses were significantly degraded compared with direct intravenous administration. In another animal study by Agrawal et al., it was shown that exosome preparations could repress growth of ovarian cancer cells even if the exosome administration level was below the optimal amount for free anthos. In comparison, free anthos did not show significant inhibition of ovarian cancer cells at the same dose [Citation66]. This study showcases that the antitumor effect of exosomes can be more efficient than that of the free drug itself. Concurrently, the study also found that the combination of anthos and PTX can significantly reduce p-glycoproteins (by ∼30%) in cancer cells, enhancing the sensitivity of PTX-resistant ovarian cancer.
Dox is another common antitumor drug used in many cancers, but it usually leads to serious cytotoxicity or cardiotoxicity. In addition, its nonspecific tissue targeting and poor half-life result in poor drug efficacy. Gong et al. found that, compared with the free Dox-treated group, Exo-Dox could effectively inhibit breast cancer cell proliferation while significantly reducing cardiotoxicity [Citation55]. Regarding cardiotoxicity, several studies have found that the cardiomyocyte H9C2 cell line’s uptake of Exo-Dox was much lower than for free Dox at levels where Exo-Dox efficiently killed the osteosarcoma MG63 cell line. This effect may be due to the interaction between exosomes derived from marrow mesenchymal stem cells and membrane proteins on the surface of the MG63 cells [Citation18]. Nie and Wei’s groups prepared iRGD-Exo-Dox by using exosomes derived from mouse immature dendritic cells (DCs) which were engineered to express exosomal membrane protein (Lamp2b) fused to αv integrin-specific iRGD peptide (CRGDKGPDC) [Citation23]. The iRGD exosomes were loaded with Dox via electroporation and showed highly efficient targeting and Dox delivery to breast cancer cells. iRGD-Exo-Dox or equal doses of free Dox were intravenously injected into the tumor-bearing mice every day for a total of six times, then the activities of serum creatinase MB isoenzyme and aspartate aminotransferase were measured to evaluate the level of heart damage. The results showed that levels of the two enzymes were significantly lower in the iRGD-Exo-Dox group (p < 0.01), indicating that being encapsulated by exosomes reduced the cardiotoxicity of Dox [Citation23].
To track the distribution of EVs in tumor-bearing mice, the researchers prepared DiR dye-labeled exosomes and injected them into the mice through the tail vein, taking DiR-loaded micelles DiR@PEG-PE as a comparison [Citation20]. Then they used a real-time imaging system to determine the in vivo biodistribution of these exosomes at 2, 4 and 8 h. The results showed that DiR-labeled exosomes accumulated in the tumor site in a time-dependent manner. DiR-labeled exosomes reached the tumor site at 4 h and accumulated more at 8 h, while DiR@PEG-PE failed to reach the tumor site. Also corresponding to the biodistribution study of gemcitabine (GEM), the researchers found that Exo-GEM can effectively promote the accumulation of GEM at the tumor site [Citation20]. In order to evaluate the safety of exosomal drug delivery vehicles, Liang et al. conducted the following experiments. Through detecting cell proliferation using the Cell Counting Kit-8 (CCK-8) assay, they found that the IC50 value of norcantharidin (NCTD) was 26.14 μg/ml, while the IC50 value of NCTD encapsulated in exosomes (Exo-NCTD) was 10.89 μg/ml. The drug release curve shows that the cumulative release of free NCTD will be complete after 10 h with a release rate of 93.02%, while Exo-NCTD showed sustained release after 12 h, with a release rate of 79.84% at 36 h. In vivo experiments on nude mice with hepatocellular carcinoma indicated the liver and kidney tissues of the NCTD-treated mice were damaged to a certain extent, while the Exos-NCTD-treated mice had no obvious tissue damage [Citation19]. Another group used nomadic dye IR780 and Exo-IR780 to evaluate the efficiency of exosome homing and penetration in pancreatic cancer. The MiaPaca-2 cell line, stably transfected with luciferase, was implanted into the pancreas of nude mice to prepare an orthotopic pancreatic ductal adenocarcinoma model. The biodistribution of injected nomadic IR780 and Exo-IR780 were observed at predesignated time points (1, 4, 8, 12, 24 and 48 h). At 12 h, it was found that Exo-IR780 began to accumulate in the tumor, while most of the nomadic IR780 had been metabolized by liver and kidney. Within 24–48 h, Exo-IR780 showed obvious and permanent aggregation in the tumor area, while the nomadic IR780 had been completely excreted. These results show that EVs have excellent tumor homing ability [Citation67].
RNA molecules
RNA interference (RNAi) has unique advantages in treating cancer. RNAi mainly conducts gene regulation via shRNA, siRNA and miRNA. Despite advances in technology, the delivery of shRNA, siRNA and miRNA remains challenging for the following reasons: first, the problem of how to avoid nonspecific delivery, especially to the liver; and second, how to minimize the immunogenicity of delivery vehicles. EVs were proposed to be good candidates for RNA delivery, having been applied to synergize with chemotherapeutic drugs to increase their efficiency in cancer cells. Alvarez-Erviti et al. firstly loaded siRNA into DC-derived exosomes and delivered them to the brain in mice, via engineering the DCs to express Lamp2b [Citation53]. c-Met is the tyrosine kinase receptor of hepatocyte growth factor, which participates in the development of malignant tumors and plays a role in the processes of proliferation, invasion and metastasis [Citation68]. Zhang et al. found that the transfection of exosomal si-c-Met into gastric cancer cells could increase their sensitivity to cisplatin, which inhibited their proliferation and accelerated apoptosis [Citation69].
miRNA is a type of ncRNA; that is, a type of RNA that does not have protein coding functions. ncRNA is involved in complex network regulation and plays a role in the development of cancer [Citation70]. In the past, many studies have shown that exosomal ncRNAs play an important role in tumor progression and diagnosis [Citation71,Citation72]. However, with the deepening of current research, their role in cancer treatment is gradually being explored.
Exosomes loaded with ncRNA can inhibit cancer progression by inhibiting tumor angiogenesis, such as in one study where mesenchyme-derived miR-100 retarded breast cancer angiogenesis [Citation73]. Guohua Louet et al. found that when miR-122 was transfected into adipose-derived mesenchymal stem cells, they could efficiently produce exosomes (122-Exo) that changed the miR-122 target gene in hepatocellular carcinoma and increased the sensitivity of cancer cells to chemotherapy drugs [Citation74]. Zhang et al. found that the EVs of normal cells would load lncRNA PTENP1 and deliver it to bladder cancer cells, thereby promoting cell apoptosis and inhibiting the invasion and metastasis of bladder cancer [Citation75].
As shown in , EVs can transfer RNA molecules to control the progress of cancer by improving the cells’ sensitivity to chemotherapy drugs, inhibiting growth, invasion and metastasis [Citation76–79]. Although there are still many difficulties to overcome, such as how to accurately deliver EVs to cancer cells and how to clear them in vivo, EVs loaded with RNA molecules are still highly anticipated to be practicable in cancer therapy.
Table 3. Anticancer ability of extracellular vesicles loaded with RNA molecules.
Tumor antigens for cancer immunotherapy
EVs extracted and purified from certain cells express tumor antigens on their surface, making these exosomes immune cell activators in cancer immunotherapy. DCs are antigen-presenting cells with the capacity to induce immune responses in vivo. DC-derived exosomes (DEX) have been shown to be excellent candidates for cancer immunotherapy. Using an IFN-γ enzyme-linked immunosorbent spot assay, Admyre et al. found that EVs derived from antigen-presenting cells such as DCs can directly stimulate peripheral human T cells [Citation80]. They incubated monocyte-derived DCs (MDDCs) with a viral peptide mix containing 23 viral MHC class I-specific peptides from three viruses: Epstein–Barr virus, cytomegalovirus and influenza virus. The viral peptide mix was used as an antigen, and EVs obtained from the MDDCs were verified to be loaded with the antigen. MDDC-derived exosomes were then added to antigen-specific CD8+ T cells and were found to be able to stimulate these immune cells [Citation80].
In 1998 Amigorena et al. coincubated DCs with acid-eluted tumor peptides for 18–20 h, collected the supernatant and obtained exosomes via ultracentrifugation. P815 (a type of mast cell tumor) and TS/A (a type of undifferentiated breast cancer) were subcutaneously inoculated into the right upper limb of DBA/2 and BALB/c mice. After tumor formation, 3–5 ng of exosomes were injected intracutaneously into the mice for immunotherapy. Within 1 week of therapy initiation, the tumors in the mice injected with P815 stopped growing. On the 60th day, 40–60% of the mice had no tumors. TS/A tumors established in BALB/c mice showed similar results. These results indicate that exosomes derived from DCs have powerful antitumor effects. In order to verify that the antitumor effect of exosomes derived from DCs was greater than that of the DCs alone, the experimenters treated P815 tumor-forming mice with tumor peptide-loaded DCs and exosomes derived from the same number of tumor peptide-loaded DCs. Approximately 20% of the mice injected with DCs were tumor-free on day 60, indicating that the exosomes of DCs have a superior antitumor effect over DCs alone [Citation81].
Lyerly et al. conducted a Phase I clinical trial to study the safety, feasibility and effectiveness of DEX loaded with MAGE tumor antigens in the treatment of non-small-cell lung cancer (NSCLC) [Citation82]. The study included patients with HLA A2+, stage IIIb (n = 4) and stage IV (n = 9) NSCLC pre-treatment, whose tumors expressed MAGE-A3 or A4. The patients received four doses of DEX generated by leukocyte-separated DCs and loaded with MAGE-A3, -A4, -A10 and -3DPO4 peptides each week. The results showed that in patients with advanced NSCLC, DEX could be utilized as a vaccine, and its administration was tolerated.
In order to explore the clinical significance of DEX-based immunotherapy, Chaput et al. designed a Phase II clinical trial on the maintenance of immunotherapy after induction chemotherapy [Citation83]. The study enrolled patients with advanced NSCLC who were not eligible for locoregional treatment: HLA-A2+, responding or stable after four cycles of platinum chemotherapy, and with neutrophils ≥1.5 × 109/l. Patients received six DEX (IFN-γ) vaccinations every 2 weeks, followed by 2 weeks of rest, then continued vaccination every 3 weeks until they progressed or were unable to obtain DEX. The results showed that the progression-free survival at 4 months was 32% (95% CI: 16–53), and the median progression-free survival for all 22 patients was 2.2 months. The overall survival rates at 6 months, 1 year and 2 years were 86% (95% CI: 67–95), 55% (95% IC: 35–73) and 25% (95% CI: 11–47), respectively. The median overall survival was 15 months. Altogether, IFN-γ-DEX was verified to be relatively safe for use in clinical immunotherapy. Moreover, when IFN-γ-DEX was used as maintenance immunotherapy for NSCLC patients who were stable/responsive to chemotherapy, it could enhance NKp30-dependent NK cell function and has potential for use in clinical immunity to provide new governance solutions.
Li et al. conducted a Phase I clinical trial on ascites-derived exosomes (AEX) and granulocyte-macrophage colony stimulating factor (GM-CSF) to evaluate their immunotherapy effects in colorectal cancer [Citation84]. The study included 45 adult patients with advanced colorectal cancer who met these conditions: HLA-A*0201+, CEA+ in serum, and malignant peritoneal effusions with no bleeding and a protein concentration >30 g/l. These patients were randomly divided into eight groups. Patients in groups A–D received 100, 200, 300 and 500 μg of autologous AEX (in 100 μl of phosphate-buffered saline) subcutaneously in the forearm, while patients in groups E–G received 100, 200, 300 and 500 μg autologous AEX in the forearm plus 50 μg of GM-CSF (in 100 μl of phosphate-buffered saline) subcutaneously. The patients received a total of four immunizations at weekly intervals. The results showed that AEX combined with GM-CSF for colorectal cancer immunotherapy is feasible and safe and may be an alternative to immunotherapy for advanced colorectal cancer in the future.
Gabrielsson et al. loaded DC exosomes derived from bone marrow cells with α-galactosylceramide (αGC) and ovalbumin (OVA) to obtain EXO(αGC-OVA) [Citation85]. In the murine model of B16 melanoma overexpressing OVA, the authors found that the median survival rate of mice injected with EXO(αGC-OVA) was significantly higher than that of other groups. At the same time, compared with the group injected with EXO(αGC-OVA) once, two injections could significantly prolong the survival period and reduce tumor growth. This indicated that EXO(αGC-OVA) enhances innate and antigen-specific Th1 adaptive immunity and antitumor immunity. Improving the immunogenicity of EVs derived from DCs can provide a reference for future treatment of malignant diseases. Yin et al. discovered that tumor-derived EVs could trigger DCs to produce effective immune responses in liver cancer in mice and in human liver cancer cells [Citation86]. Hepa1–6 cells were injected subcutaneously into the left axilla of C57BL6 mice to establish a mouse subcutaneous liver cancer model, then EVs derived from liver cancer cells were used to activate DCs. They were found to significantly inhibit the proliferation of liver cancer cells, significantly increase T lymphocytes and IFN-γ levels and inhibit the expression of IL-10 and TGF-β. These results indicate that cell-derived exosomes–pulsed DCs (DCTEX) stimulate a strong antitumor immune response and may reshape the tumor microenvironment in mice with liver cancer.
EVs can also be modified with specific tumor antigens via engineering methods. Such EVs can activate immune cells and inhibit cancer growth. Takakura et al. used a plasmid vector encoding a protein fused with streptavidin (SAV) and lactadherin (LA) to transfect murine melanoma B16BL6 cells and achieve genetically engineered SAV-LA exosomes (SAV-Exo) [Citation87]. SAV-Exo with biotinylated CpG DNA were then used to prepare CpG DNA-modified exosomes (CpG-SAV-Exo), which were then used to deliver CpG DNA to mice dendritic DC2.4 cells. CpG-SAV-Exo treatment effectively activated DC2.4 cells and enhanced tumor antigen presentation. B16BL6 mice immunized with CpG-SAV-Exo showed stronger antitumor effects in vivo than with the administration of exosomes and CpG DNA separately. This study demonstrates that genetically engineered CpG-SAV-Exo is an effective exosome-based tumor antigen–adjuvant codelivery system with promising applications in cancer immunotherapy.
Nanomaterials
Researchers have functionalized exosomes with all manner of nanomaterials and applied them to the diagnosis and treatment of cancer [Citation88]. A typical representative of nanomaterial-functionalized exosomes is magnetic exosomes. Once functionalized with iron oxide nanoparticles, exosomes gain magnetic properties and can be tracked by MRI [Citation89]. Reticulocyte (RTC)-derived exosomes contain transferrin receptors, which can be used in superparamagnetic nanoparticle (SMNC)–transferrin conjugation. Employing this property, Yuan et al. conceived of a RTC-derived exosome-based superparamagnetic nanoparticle cluster (SMNC-EXO) strategy for anticancer drug delivery [Citation90]. SMNC-EXOs were collected via magnetic separation from predialyzed serum extracted from the blood of healthy mice. Via a hydrophobic effect, Dox was loaded into the SMNC-EXOs to form D-SMNC-EXOs. The magnetic targeting ability of SMNC-EXOs was evaluated by a microfluidic system. The results showed that SMNC-EXOs could be retained and directly accumulate via an externally applied moderate magnetic field. In vitro and in vivo studies further verified the magnetic targeting ability and anticancer effect of SMNC-EXOs, highlighting EVs’ potential as an anticancer tool.
Exosomes can penetrate the blood–brain barrier and thus have a strong prospect in the treatment of brain tumors. In one such study, Tang et al. encapsulated superparamagnetic iron oxide nanoparticles and curcumin into exosomes and then conjugated the obtained exosomes with neuropilin-1-targeted peptide (RGERPPR, RGE) via click chemistry [Citation91]. The obtained engineered exosomes were glioma-targeting, with a glioma theranostic effect. The magnetothermal effect of the nanoparticles could induce hyperthermia which, when combined with curcumin-mediated therapy, led to improved survival.
Besides magnetic nanoparticles, other nanoparticles have also been loaded in exosomes for the diagnosis and treatment of various diseases. Typically, nanomaterials can be encapsulated in exosomes via the indirect labeling mode through parent cells. As mentioned above, exosomes can bypass blood–brain barriers, providing new strategies for brain imaging and brain targeted therapy [Citation92,Citation93]. Popovtzer et al. labeled exosomes with gold nanoparticles (GNPs) via the direct labeling mode, which was found to be more efficient than the indirect labeling method. They verified that the glucose-coated GNPs were taken into mesenchymal stem cell-derived exosomes via an active, energy-dependent method mediated by the glucose transporter GLUT-1 and endocytic proteins. The obtained GNP-labeled exosomes were used in neuroimaging via intranasal administration in mice [Citation93]. Using the mechanical method or extrusion, Namdee et al. synthesized GNPs encapsulated by neuron-specific rabies viral glycoprotein (RVG)-targeted exosomes. They demonstrated the enhanced targeting ability of these exosomes in blood–brain barrier models [Citation92]. The studies in this field of nanomaterials have demonstrated the feasibility of loading nanoparticles and chemical agents synchronously into exosomes, which could improve system performance by overcoming individual limitations and enabling synergistic effects. We have reasons to believe that these engineered exosomes will play powerful roles in the early diagnosis and effective treatment of cancer.
Increasing EV production
Mammalian cells usually release a low quantity of EVs, which is problematic as they are the most used EV source in cancer treatment. Animal milk can also serve as a scalable source of EVs [Citation25]. EVs can be isolated from bovine raw milk by differential centrifugation, with an average yield of 335 ± 48 mg/l of milk. When stored with ≤6 mg EV protein/ml at -80°C, the obtained EVs remain largely free of coagulation for up to 18 months. These bovine milk-derived EVs have been proven to be biocompatible both in vitro and in vivo, highlighting them as suitable candidates for drug delivery. Plants and bacteria are also studied as abundant sources of EVs according to the condition of the treatment subject.
Researchers have reported several physical methods to increase the production of mammalian EVs. Changes in temperature have been shown to impact the efflux quantity of EVs by tumor cells. One study found that high temperatures in tumor hyperthermia significantly enhance the release of tumor cell EVs [Citation94]. However, this hyperthermia method also caused the volume of each individual EV to increase. Another approach, focused ultrasound, has been showcased as a noninvasive method for generating thermal energy and mechanical destruction that was able to increase the secretion of exosomes by approximately 46% without altering their size [Citation95]. In 2013 Gho et al. produced large amounts of nanovesicles via the breakdown of monocytes or macrophages through a serial extrusion method, using filters with diminishing pore sizes ranging from 10 to 1 μm [Citation96]. This method not only produced a 100-fold increase in production yield, but showed that the exosome mimic retained the basic characteristics of EVs. In 2016 the same group applied this extrusion method to the construction of siRNA-loaded exosome-mimetic nanovesicles [Citation14]. In this study, they evaluated whether the obtained exosome mimics could be loaded with siRNA exogenously or endogenously and whether the siRNA-loaded nanovesicles could be taken up by recipient cells to induce knockdown responses. Either an anti-GFP siRNA was first loaded into nanovesicles by electroporation, or c-Myc shRNA was expressed inside the cells. The nanovesicles were successfully loaded by both techniques and then taken up by recipient cells, resulting in attenuated expression of the targeted gene. This study shows that exosome-mimetic nanovesicles can serve as a platform for RNAi delivery to cell cytoplasm even after scaling up 100-fold. Similarly, Lee and Teng’s group obtained exosome mimics by serial extrusion of nontumorigenic epithelial MCF-10A cells and applied them in gene delivery in cancer both in vitro and in vivo [Citation97].
Many chemicals have been applied to increase EV secretion via disrupting lysosome function or improving membrane permeability to certain ions. Monensin is widely used to stimulate EV secretion because it can increase the intracellular Ca2+ by Na+/H+ exchange and is also found to inhibit the formation of apoptotic bodies [Citation98,Citation99]. Exposure to the calcium ionophore ionomycin can also increase exosome secretion via the same mechanism [Citation100]. It has been reported that lysosome dysfunction prevents efficient lysosome and multivesicular body fusion, which will promote EV secretion [Citation101]. Through incubating cells with 20 mM ammonium chloride for up to 7 days, or with 200 nM bafilomycin A1 for up to 72 h, Alvarez-Erviti et al. achieved lysosomal inhibition of human neuroblastoma cells SH-SY5Y, leading to an increase in exosome release [Citation102]. The lysosome inhibitor chloroquine is frequently used as a positive control in stimulating exosome secretion [Citation103].
In addition to physical and chemical methods, changes to the culture system might also be able to increase the production of EVs. Liu et al. used a hollow-fiber bioreactor based on 3D culture systems to produce mesenchymal stem cell-derived-exosomes [Citation104]. Compared with the traditional 2D flask culture system, they found that this method increased the production of exosomes 19.4-fold when vessels were inoculated with the same number of cells. In the 3D culture system, the concentration of EVs in the supernatant was higher than that in the traditional culture system (15.5-fold), resulting in a higher EV collection efficiency. Yang et al. reported a cellular nanoporation (CNP) method to increase the exosome yield. They developed a CNP system consisting of a nanochannel array with 500-nm channels [Citation105]. A monolayer of various source cells can be cultured above the CNP biochip surface. When incubated in the plasmid DNA buffer and stimulated with transient electrical pulses, the plasmid DNA enters the cells and the membrane is damaged. In order to repair the damage, the source cells secrete a large amount of exosomes carrying endogenously transcribed mRNA, which can be collected for drug delivery. Compared with conventional bulk electroporation, this CNP system produced up to 50-fold more exosomes. These mRNA-containing exosomes can be used in the inhibition of different tumors, indicating that the CNP method may enable the clinical use of exosomes as nucleic acid carriers for cancer therapy.
Enhancing the tumor-targeting ability of EVs
EVs are limited in their abilities to target specific recipient cell types. For precise cancer treatment, achieving better targeting is therefore an important direction for EV-based tumor therapy research. To this end, several strategies have been developed to enhance the tumor targeting ability of EVs.
Reducing macrophage targeting of EVs
As a nanostructure, EVs can be absorbed quickly by the MPS in vivo; hence reducing the uptake of exosomes by the MPS is of critical importance. In addition to the phagocytic effect, efficient endocytosis also contributes to the uptake of EVs by macrophages. In an attempt to elucidate the mechanisms behind this uptake, Liu et al. compared the expression of endocytosis-associated genes in the liver and found that the expression of clathrin heavy chain (Cltc) was higher than that of Cav1, Pak1 or Rhoa [Citation106]. When compared with heart, the expression of Cltc in the liver was also much higher. From this the authors hypothesized that Cltc plays a vital role in mediating endocytosis of exosomes in the liver and spleen. In vitro experiments showed that the Cltc blocker siClathrin was able to significantly reduce the uptake of exosomes by RAW264.7 cells. In animal model experiments of heart injury, siClathrin was able to block the MPS of the liver and spleen and increase the absorption of cardiac protective exosomes by the heart. It is plausible that the findings of their work could be translated over to exosome-mediated cancer therapy, and indeed this method has been used as a strategy to enhance tumor targeting of exosomes via inhibiting endocytosis by the MPS [Citation106].
To reduce macrophage targeting of EVs, fusogenic exosomes have been constructed through engineering exosomes with liposomes, with modification by other molecules such as polyethylene glycol (PEG). The nature of the exosome’s surface allows it to be easily modified by PEG liposomes. After the PEG-induced fusion between liposomes of a specific composition and cell-derived exosomes, researchers have found that the cargo carried by the exosomes themselves is not lost and maintains its original physiological characteristics in the exosomes [Citation107]. Under optimized conditions, more than 60% of the membrane and soluble content can be transferred from liposomes to exosomes, indicating that the targeted substances on the surface of liposomes might be transferred into the mixed exosomes. The presence of PEG on the surface of liposomes has been shown to extend their circulation time in the blood via reducing their uptake by mononuclear phagocytes [Citation108]. Consequently, PEG-modified mixed exosomes can increase the exosomes’ interaction time with cells, thereby increasing exosome uptake by the target cells [Citation109]. Exosome membranes inserted into EGFR-specific antibodies conjugated with a phospholipid (DMPE)-PEG derivative have been shown to increase exosome circulation time in vivo. As with the previous example, this may increase the accumulation of exosomes in target organs and improve the efficiency of drug action.
Magnetic targeting
As discussed previously, EVs modified by magnetic nanoparticles have enhanced targeting capability [Citation110]. The aforementioned study by Yuan et al. indicated that SMNCs were anchored to each RTC-derived exosome through iron transporter receptor interaction and that these exosomes responded to external magnetic fields, enabling the authors to direct the therapeutic. Both in vivo and in vitro experiments have shown that the effectiveness of DOX-SMNC-EXOs on tumors can be increased under an external magnetic field [Citation90].
Modifying EVs with targeting molecules
Functionalizing EVs with targeting molecules – including antibodies and peptides – is the most commonly employed strategy to enhance tumor targeting. EGFR is a transmembrane tyrosine kinase receptor involved in the progression of a variety of cancers and may be a promising target for cancer treatment [Citation111–116]. Because EGF, the natural ligand for EGFR, has strong mitogenic and neoangiogenic effects, it is necessary to find new specific ligands. A study by Li et al. screened EGFR-binding peptides and found that the GE11 peptide specifically binds to EGFR, with no obvious mitogenic activity [Citation117]. In an HCC70 xenograft breast cancer model, Ohno et al. used exosomes modified with the GE11 peptide to deliver a cancer suppressor let-7a miRNA to breast cancer tissue expressing EGFR after intravenous administration. Their results showed that the exosomal surface containing the GE11 peptide was able to achieve the targeted delivery of drugs to tumor tissues [Citation113]. Another study showed that modification of EVs with specific IRGD peptides (CRGDKGPDC) produced efficient targeting for αv integrin-positive breast cancer cells [Citation23]. Unfortunately, the targeting peptide was too unstable and degraded during transportation in the body. To improve its stability and avoid degradation, the group increased the glycosylation stabilization sequence and achieved satisfactory results [Citation118]. In another study, the researchers modified exosomes with cationized pollutant, which has the ability to target hepatocyte asialoglycoprotein receptors in liver cells [Citation119].
Aptamers are a series of single-stranded oligonucleotides that normally contain between 20 and 80 nucleotides; they are also called ‘chemical antibodies’ because they can bind to target molecules with great affinity and specificity. One study by Tan et al. reported aptamer-based DNA nanoassemblies on exosomes via an in situ assembly method. This method utilizes the molecular recognition between DNA aptamers and exosome surface markers, as well as the DNA hybridization chain reaction initiated by an aptamer-chimeric trigger. Compared with genetic engineering and chemical conjugation methods, this in situ assembly method is easier to operate, and the aptamer-based nanoassemblies can be selectively assembled on target cell-derived exosomes, while avoiding the nontarget exosomes [Citation120]. In 2018 Zheng et al. isolated living mouse DCs and covalently conjugated a nucleolin-targeting aptamer, AS1411, onto their membrane. In brief, AS1411 was first covalently conjugated to cholesterol-PEG to obtain AS1411-PEG2000-chol. Second, AS1411-PEG2000-chol was anchored to the DC membrane via cholesterol efficiently inserting into the cellular lipid bilayer. Subsequently, through a strainer, the aptamer-grafted exosomal analog was obtained by extrusion. All exosomes carried the AS1411 aptamers and were able to target MDA-MB-231 cells. This methodology can obtain a large number of targeted exosomes in a short time and provides a feasible program for the clinical application of exosomes [Citation121].
Increasing the expression of targeted molecules via cell modification
In recent years, EVs expressing specific surface molecules have primarily been obtained through gene transfection of the donor cells. In one example, researchers used a vector to transfect the gene encoding GE11 into human embryonic kidney cell line 293 (HEK293) to obtain exosomes with the GE11 peptide on the surface [Citation113]. The researchers used an injection of let-7a-loaded GE11-positive exosomes to treat breast cancer mouse models (tumors highly expressing EGFR), and found that tumor sizes were significantly reduced compared with the control group. These results showed that GE11-positive exosomes can enhance the targeting of drugs to EGFR-expressing tumors. In another study, Apo-A1 – a known target of the scavenger receptor class B type 1 (SR-B1) – was expressed via a gene fusion between the transmembrane protein CD63 and a sequence from Apo-A1 in human embryonic kidney 293T (HEK293T) cells [Citation122]. Researchers then isolated and purified the exosomes from the Apo-A1-overexpressing HEK293T cells to obtain Apo-A1-engineered exosomes (Apo-Exo). Using the electroporation method, they then loaded the Apo-Exo with miR-26a. These exosomes were able to specifically bind to hepatoma cells expressing SR-B1 receptors, enabling Apo-Exo/miR-26a to be internalized by HepG2 cells via SR-B1 receptor-mediated endocytosis and inhibit cell proliferation. Researchers have also been able to transfect HEK293T cells with the Pinco plasmid containing the recombinant human Lamp2b gene fused to the human IL-3 gene fragment to produce exosomes expressing IL3-Lamp2b (IL3L) [Citation123]. In vitro, confocal experiments showed that both imatinib-sensitive and imatinib-resistant strains internalized IL3L exosomes more rapidly than the non-exosome counterparts. Furthermore, IL3L exosomes loaded with imatinib or BCR-ABL siRNA were able to induce significant tumor shrinkage in chronic myelogenous leukemia. Exosomes expressing IL-3 fragments have been used for patients with chronic myelogenous leukemia in receptor-targeted therapy, particularly to provide new solutions for the treatment of drug-resistant patients.
Conclusion
Recently, there have been major breakthroughs in the treatment of tumors, such as oncogene sequencing, the application of targeted drugs and the implementation of tumor vaccines [Citation124–126]. With the quantity and diversity of studies going into cancer therapy, treatments have gradually diversified. The present review covers the most recent efforts to apply EVs in cancer treatment. We summarized all kinds of sources of EVs, including mammalian cells, animal milk, plants and bacteria. EV-mimicking nanoparticles were also incorporated into the sources of EVs because of their similar structure and function. The engineering of EVs, including EV yield improvement, cargo loading and targeted delivery, was also introduced.
Future perspective
Nowadays, precise treatment is a constant pursuit of researchers. With the discovery of and research done on EVs, many scholars believe they are one of the most exciting vehicles for the precise treatment of tumors. However, the research on tumor EVs has just begun, and the research content is scattered. In other words, there are still many problems to be solved. We must first understand whether or not EVs themselves have a specific anticancer effect, as some studies have shown [Citation61]. If they do, the EV components responsible for this effect must be identified, and whether this effect applies across all tumors, or is cancer type specific, warrants further exploration. On the other hand, EVs from the same cells may have different subtypes, which offers a fertile field for study.
In addition to their potential innate advantages, EVs as drug carriers have several avenues for improvement. The sources of EVs are very varied, and the cell source most suited for use as a ‘factory’ for the mass production of EVs remains a contentious subject. Nonetheless, there are many methods for drug loading in EVs, making the mass production of EVs a tantalizing possibility rather than wishful thinking. In application, several questions to optimize this process remain; the isolation, purification, detection and identification of EV specimens are complicated and time-consuming, severely limiting their clinical application. Faster and more streamlined methods need to be developed. How can researchers purify and identify EV-based drugs? How can they avoid clearance by the MPS in vivo? How can EVs be preserved when transporting RNA molecules to avoid degradation of activity? Despite these questions, EVs have shown they are suitable for administration and can play an effective role in cancer therapy. In a preclinical study, we should not only consider the curative effect of an EV-based delivery system, but also the drug metabolic clearance rate. Modification strategies to improve drug clearance rate and avoid long-term accumulation in patients requires further exploration.
Targeted therapy is a promising field in cancer treatment, and it is worth considering how to achieve ideal EV targeting. Although EVs can be directed by specific targeting peptides, further evaluation is needed to explore how receptor-targeting peptides are able to target tumor tissues with specificity while avoiding damage to normal tissues. In addition, how to avoid the degradation of these targeted substances once injected into the body, and how to effectively dispose of them once their role is played, are research directions needed before their clinical administration. We believe that with continuous systematic and in-depth study, EVs will achieve the goal of precise cancer treatment.
Source of extracellular vesicles
Extracellular vesicles (EVs) can be sourced from human cells, animal cells, plants and bacteria.
Besides natural EVs, exosomal biomimetic particles have also been used as drug carriers.
Drug types & loading methods
Chemotherapeutic compounds, RNA molecules and nanomaterials can be delivered by EVs to play anticancer roles.
EVs derived from certain cells express tumor antigens on their surface, making these EVs immune cell activators in cancer immunotherapy.
Drugs can be loaded in EVs via direct or indirect methods.
Changing the physical or chemical conditions of preparation can promote the secretion and release of EVs.
Changing culture conditions of the source cells can also increase EV production.
Cancer-targeting ability of EVs
Several strategies, such as engineering exosomes with liposomes, modifying EVs with targeting ligands, and source cell modification, have been developed to enhance the tumor targeting ability of EVs.
With the discovery of and research done on EVs, many scholars believe they are one of the most exciting vehicles for precise cancer treatment.
Author contributions
X-H Cao, M-X Liang and K Yang read the literature and wrote the original draft. W Zhang and J-H Tang conceived and designed the article framework. Y Wu, W Zhang and J-H Tang reviewed and edited the manuscript.
Financial & competing interests disclosure
This work was funded by the National Natural Science Foundation of China (grant number 81872365, 81801827 and 81901883), the Basic Research Program of Jiangsu Province (grant number BK20181086 and BK20191080). The authors have no other relevant affiliations or financial involvement with any organization or entity with a financial interest in or financial conflict with the subject matter or materials discussed in the manuscript apart from those disclosed.
No writing assistance was utilized in the production of this manuscript.
Additional information
Funding
References
- Siegel RL , MillerKD, FuchsHE, JemalA. Cancer Statistics, 2021. CA Cancer J. Clin.71(1), 7–33 (2021).
- Morrissey KM , YuraszeckTM, LiCC, ZhangY, KasichayanulaS. Immunotherapy and novel combinations in oncology: current landscape, challenges, and opportunities. Clin. Transl. Sci.9(2), 89–104 (2016).
- Fisher B . Biological research in the evolution of cancer surgery: a personal perspective. Cancer Res.68(24), 10007–10020 (2008).
- Royce TJ , QureshiMM, TruongMT. Radiotherapy utilization and fractionation patterns during the first course of cancer treatment in the United States from 2004 to 2014. J. Am. Coll. Radiol.15(11), 1558–1564 (2018).
- Rangarajan K , PucherPH, ArmstrongT, BatemanA, HamadyZ. Systemic neoadjuvant chemotherapy in modern pancreatic cancer treatment: a systematic review and meta-analysis. Ann. R. Coll. Surg. Engl.101(7), 453–462 (2019).
- He J , FanK, YanX. Ferritin drug carrier (FDC) for tumor targeting therapy. J. Control. Release311–312, 288–300 (2019).
- Xue J , ZhaoZ, LeiZ, XueL, ZhangC. Neutrophil-mediated anticancer drug delivery for suppression of postoperative malignant glioma recurrence. Nat. Nanotechnol.12(7), 692–700 (2017).
- Kimbrel EA , LanzaR. Next-generation stem cells – ushering in a new era of cell-based therapies. Nat. Rev. Drug Discov.19(7), 463–479 (2020).
- Guido C , MaioranoG, CorteseB, D’amoneS, PalamàI. Biomimetic nanocarriers for cancer target therapy. Bioengineering7(3), 111 (2020).
- Cho E , NamGH, HongYet al. Comparison of exosomes and ferritin protein nanocages for the delivery of membrane protein therapeutics. J. Control. Release279, 326–335 (2018).
- Trams EG , LauterCJ, SalemN, HeineUJr. Exfoliation of membrane ecto-enzymes in the form of micro-vesicles. Biochim. Biophys. Acta645(1), 63–70 (1981).
- Lyu TS , AhnY, ImYJ, KimSS, ChoJA. The characterization of exosomes from fibrosarcoma cell and the useful usage of Dynamic Light Scattering (DLS) for their evaluation. PLoS ONE16(1), e0231994 (2021).
- Kalluri R , LebleuVS. The biology, function, and biomedical applications of exosomes. Science367(6478), 640 (2020).
- Lunavat TR , JangSC, NilssonLet al. RNAi delivery by exosome-mimetic nanovesicles – implications for targeting c-Myc in cancer. Biomaterials102, 231–238 (2016).
- Kim YK , ChoiY, NamGH, KimIS. Functionalized exosome harboring bioactive molecules for cancer therapy. Cancer Lett.489, 155–162 (2020).
- Neviani P , WisePM, MurtadhaMet al. Natural killer-derived exosomal miR-186 inhibits neuroblastoma growth and immune escape mechanisms. Cancer Res.79(6), 1151–1164 (2019).
- Phan J , KumarP, HaoD, GaoK, FarmerD, WangA. Engineering mesenchymal stem cells to improve their exosome efficacy and yield for cell-free therapy. J. Extracell. Vesicles7(1), 1522236 (2018).
- Wei H , ChenJ, WangSet al. A nanodrug consisting of doxorubicin and exosome derived from mesenchymal stem cells for osteosarcoma treatment in vitro. Int. J. Nanomedicine14, 8603–8610 (2019).
- Liang L , ZhaoL, WangY, WangY. Treatment for hepatocellular carcinoma is enhanced when norcantharidin is encapsulated in exosomes derived from bone marrow mesenchymal stem cells. Mol. Pharm.18(3), 1003–1013 (2021).
- Li Y , WuJ, WangJ, HuX, CaiJ, XiangD. Gemcitabine loaded autologous exosomes for effective and safe chemotherapy of pancreatic cancer. Acta Biomater.101, 519–530 (2020).
- Charoenviriyakul C , TakahashiY, MorishitaM, MatsumotoA, NishikawaM, TakakuraY. Cell type-specific and common characteristics of exosomes derived from mouse cell lines: yield, physicochemical properties, and pharmacokinetics. Eur. J. Pharm. Sci.96, 316–322 (2017).
- Wang P , WangH, HuangQet al. Exosomes from M1-polarized macrophages enhance paclitaxel antitumor activity by activating macrophages-mediated inflammation. Theranostics9(6), 1714–1727 (2019).
- Tian Y , LiS, SongJet al. A doxorubicin delivery platform using engineered natural membrane vesicle exosomes for targeted tumor therapy. Biomaterials35(7), 2383–2390 (2014).
- Tian R , WangZ, NiuR, WangH, ChangJ. Tumor exosome mimicking nanoparticles for tumor combinatorial chemo-photothermal therapy. Front. Bioeng. Biotechnol.8, 1010 (2020).
- Munagala R , AqilF, JeyabalanJ, GuptaRC. Bovine milk-derived exosomes for drug delivery. Cancer Lett.371(1), 48–61 (2016).
- Wang Q , RenY, MuJet al. Grapefruit-derived nanovectors use an activated leukocyte trafficking pathway to deliver therapeutic agents to inflammatory tumor sites. Cancer Res.75(12), 2520–2529 (2015).
- Zhang M , XiaoB, WangHet al. Edible ginger-derived nano-lipids loaded with doxorubicin as a novel drug-delivery approach for colon cancer therapy. Mol. Ther.24(10), 1783–1796 (2016).
- Kim OY , ChoiSJ, JangSCet al. Bacterial protoplast-derived nanovesicles as vaccine delivery system against bacterial infection. Nano Lett.15(1), 266–274 (2015).
- Kim OY , DinhNTH, ParkHT, ChoiSJ, HongK, GhoYS. Bacterial protoplast-derived nanovesicles for tumor targeted delivery of chemotherapeutics. Biomaterials113, 68–79 (2017).
- Hoshino A , Costa-SilvaB, ShenTLet al. Tumour exosome integrins determine organotropic metastasis. Nature527(7578), 329–335 (2015).
- Samuel M , GabrielssonS. Personalized medicine and back-allogeneic exosomes for cancer immunotherapy. J. Intern. Med.289(2), 138–146 (2021).
- Azoulay-Alfaguter I , MorA. Proteomic analysis of human T cell-derived exosomes reveals differential RAS/MAPK signaling. Eur. J. Immunol.48(11), 1915–1917 (2018).
- Ferguson SW , WangJ, LeeCJet al. The microRNA regulatory landscape of MSC-derived exosomes: a systems view. Sci. Rep.8(1), 1419 (2018).
- Cully M . Exosome-based candidates move into the clinic. Nat. Rev. Drug Discov. (2020). https://www.nature.com/articles/d41573-020-00220-y
- Parodi A , MolinaroR, SushnithaMet al. Bio-inspired engineering of cell- and virus-like nanoparticles for drug delivery. Biomaterials147, 155–168 (2017).
- Kooijmans SA , VaderP, Van DommelenSM, Van SolingeWW, SchiffelersRM. Exosome mimetics: a novel class of drug delivery systems. Int. J. Nanomedicine7, 1525–1541 (2012).
- Manca S , UpadhyayaB, MutaiEet al. Milk exosomes are bioavailable and distinct microRNA cargos have unique tissue distribution patterns. Sci. Rep.8(1), 11321 (2018).
- Kausar H , JeyabalanJ, AqilFet al. Berry anthocyanidins synergistically suppress growth and invasive potential of human non-small-cell lung cancer cells. Cancer Lett.325(1), 54–62 (2012).
- Munagala R , AqilF, JeyabalanJet al. Exosomal formulation of anthocyanidins against multiple cancer types. Cancer Lett.393, 94–102 (2017).
- Carobolante G , MantajJ, FerrariE, VllasaliuD. Cow milk and intestinal epithelial cell-derived extracellular vesicles as systems for enhancing oral drug delivery. Pharmaceutics12(3), 226 (2020).
- Aqil F , MunagalaR, JeyabalanJ, AgrawalAK, GuptaR. Exosomes for the enhanced tissue bioavailability and efficacy of curcumin. AAPS J.19(6), 1691–1702 (2017).
- Rahimi Ghiasi M , RahimiE, AmirkhaniZ, SalehiR. Leucine-rich repeat-containing G-protein coupled receptor 5 gene overexpression of the rat small intestinal progenitor cells in response to orally administered grape exosome-like nanovesicles. Adv. Biomed. Res.7, 125 (2018).
- Raimondo S , NaselliF, FontanaSet al. Citrus limon-derived nanovesicles inhibit cancer cell proliferation and suppress CML xenograft growth by inducing TRAIL-mediated cell death. Oncotarget6(23), 19514–19527 (2015).
- Chin AR , FongMY, SomloGet al. Cross-kingdom inhibition of breast cancer growth by plant miR159. Cell Res.26(2), 217–228 (2016).
- Silverman JM , ClosJ, De’oliveiraCCet al. An exosome-based secretion pathway is responsible for protein export from Leishmania and communication with macrophages. J. Cell Sci.123(Pt 6), 842–852 (2010).
- Kim MS , HaneyMJ, ZhaoYet al. Development of exosome-encapsulated paclitaxel to overcome MDR in cancer cells. Nanomedicine12(3), 655–664 (2016).
- Haney MJ , KlyachkoNL, ZhaoYet al. Exosomes as drug delivery vehicles for Parkinson’s disease therapy. J. Control. Release207, 18–30 (2015).
- Shtam TA , KovalevRA, VarfolomeevaEY, MakarovEM, KilYV, FilatovMV. Exosomes are natural carriers of exogenous siRNA to human cells in vitro. Cell Commun. Signal11, 88 (2013).
- Kooijmans SaA , StremerschS, BraeckmansKet al. Electroporation-induced siRNA precipitation obscures the efficiency of siRNA loading into extracellular vesicles. J. Control. Release172(1), 229–238 (2013).
- Zhang Y , BiJ, HuangJ, TangY, DuS, LiP. Exosome: a review of its classification, isolation techniques, storage, diagnostic and targeted therapy applications. Int. J. Nanomedicine15, 6917–6934 (2020).
- Li L , LuS, LiangXet al. γδTDEs: An efficient delivery system for miR-138 with anti-tumoral and immunostimulatory roles on oral squamous cell carcinoma. Mol. Ther. Nucleic Acids14, 101–113 (2019).
- Hood JL , ScottMJ, WicklineSA. Maximizing exosome colloidal stability following electroporation. Anal. Biochem.448, 41–49 (2014).
- Alvarez-Erviti L , SeowY, YinH, BettsC, LakhalS, WoodMJ. Delivery of siRNA to the mouse brain by systemic injection of targeted exosomes. Nat. Biotechnol.29(4), 341–345 (2011).
- Sun D , ZhuangX, XiangXet al. A novel nanoparticle drug delivery system: the anti-inflammatory activity of curcumin is enhanced when encapsulated in exosomes. Mol. Ther.18(9), 1606–1614 (2010).
- Gong C , TianJ, WangZet al. Functional exosome-mediated co-delivery of doxorubicin and hydrophobically modified microRNA 159 for triple-negative breast cancer therapy. J. Nanobiotechnology17(1), 93 (2019).
- Fuhrmann G , SerioA, MazoM, NairR, StevensMM. Active loading into extracellular vesicles significantly improves the cellular uptake and photodynamic effect of porphyrins. J. Control. Release205, 35–44 (2015).
- Kim MS , HaneyMJ, ZhaoYet al. Engineering macrophage-derived exosomes for targeted paclitaxel delivery to pulmonary metastases: in vitro and in vivo evaluations. Nanomedicine14(1), 195–204 (2018).
- Podolak I , GalantyA, SobolewskaD. Saponins as cytotoxic agents: a review. Phytochem. Rev.9(3), 425–474 (2010).
- Luan X , SansanaphongprichaK, MyersI, ChenH, YuanH, SunD. Engineering exosomes as refined biological nanoplatforms for drug delivery. Acta Pharmacol. Sin.38(6), 754–763 (2017).
- Manfredi F , DiBonito P, RidolfiBet al. The CD8+ T cell-mediated immunity induced by HPV-E6 uploaded in engineered exosomes is improved by ISCOMATRIX™ adjuvant. Vaccines (Basel)4(4), 42 (2016).
- Pascucci L , CocceV, BonomiAet al. Paclitaxel is incorporated by mesenchymal stromal cells and released in exosomes that inhibit in vitro tumor growth: a new approach for drug delivery. J. Control. Release192, 262–270 (2014).
- Kanchanapally R , DeshmukhSK, ChavvaSRet al. Drug-loaded exosomal preparations from different cell types exhibit distinctive loading capability, yield, and antitumor efficacies: a comparative analysis. Int. J. Nanomedicine14, 531–541 (2019).
- Fukuta T , NishikawaA, KogureK. Low level electricity increases the secretion of extracellular vesicles from cultured cells. Biochem. Biophys. Rep.21, 100713 (2020).
- Gelderblom H , VerweijJ, NooterK, SparreboomA. Cremophor EL: the drawbacks and advantages of vehicle selection for drug formulation. Eur. J. Cancer37(13), 1590–1598 (2001).
- Agrawal AK , AqilF, JeyabalanJet al. Milk-derived exosomes for oral delivery of paclitaxel. Nanomedicine13(5), 1627–1636 (2017).
- Aqil F , JeyabalanJ, AgrawalAet al. Exosomal delivery of berry anthocyanidins for the management of ovarian cancer. Food Funct.8(11), 4100–4107 (2017).
- Yu ZA , WzB, XcBet al. Bone marrow mesenchymal stem cells-derived exosomes for penetrating and targeted chemotherapy of pancreatic cancer. Acta Pharm. Sin. B10(8), 1563–1575 (2020).
- Bradley CA , DunnePD, BinghamVet al. Transcriptional upregulation of c-MET is associated with invasion and tumor budding in colorectal cancer. Oncotarget7(48), 78932–78945 (2016).
- Zhang Q , ZhangH, NingTet al. Exosome-delivered c-Met siRNA could reverse chemoresistance to cisplatin in gastric cancer. Int. J. Nanomedicine15, 2323–2335 (2020).
- Anastasiadou E , JacobLS, SlackFJ. Non-coding RNA networks in cancer. Nat. Rev. Cancer18(1), 5–18 (2018).
- Wang Y , LiuJ, MaJet al. Exosomal circRNAs: biogenesis, effect and application in human diseases. Mol. Cancer18(1), 116 (2019).
- Li LM , LiuZX, ChengQY. Exosome plays an important role in the development of hepatocellular carcinoma. Pathol. Res. Pract.215(8), 152468 (2019).
- Pakravan K , BabashahS, SadeghizadehMet al. MicroRNA-100 shuttled by mesenchymal stem cell-derived exosomes suppresses in vitro angiogenesis through modulating the mTOR/HIF-1alpha/VEGF signaling axis in breast cancer cells. Cell Oncol. (Dordr.)40(5), 457–470 (2017).
- Lou G , SongX, YangFet al. Exosomes derived from miR-122-modified adipose tissue-derived MSCs increase chemosensitivity of hepatocellular carcinoma. J. Hematol. Oncol.8, 122 (2015).
- Zheng R , DuM, WangXet al. Exosome-transmitted long non-coding RNA PTENP1 suppresses bladder cancer progression. Mol. Cancer17(1), 143 (2018).
- Liang Y , ZhangD, LiLet al. Exosomal microRNA-144 from bone marrow-derived mesenchymal stem cells inhibits the progression of non-small cell lung cancer by targeting CCNE1 and CCNE2. Stem Cell Res. Ther.11(1), 87 (2020).
- Chen W , QuanY, FanSet al. Exosome-transmitted circular RNA hsa_circ_0051443 suppresses hepatocellular carcinoma progression. Cancer Lett.475, 119–128 (2020).
- Wang X , LiH, LuXet al. Melittin-induced long non-coding RNA NONHSAT105177 inhibits proliferation and migration of pancreatic ductal adenocarcinoma. Cell Death Dis.9(10), 940 (2018).
- Li H , YangC, ShiY, ZhaoL. Exosomes derived from siRNA against GRP78 modified bone-marrow-derived mesenchymal stem cells suppress sorafenib resistance in hepatocellular carcinoma. J. Nanobiotechnology16(1), 103 (2018).
- Admyre C , JohanssonSM, PaulieS, GabrielssonS. Direct exosome stimulation of peripheral human T cells detected by ELISPOT. Eur. J. Immunol.36(7), 1772–1781 (2006).
- Zitvogel L , RegnaultA, LozierAet al. Eradication of established murine tumors using a novel cell-free vaccine: dendritic cell-derived exosomes. Nat. Med.4(5), 594–600 (1998).
- Morse MA , GarstJ, OsadaTet al. A Phase I study of dexosome immunotherapy in patients with advanced non-small cell lung cancer. J. Transl. Med.3(1), 9 (2005).
- Besse B , CharrierM, LapierreVet al. Dendritic cell-derived exosomes as maintenance immunotherapy after first line chemotherapy in NSCLC. Oncoimmunology5(4), e1071008 (2016).
- Dai S , WeiD, WuZet al. Phase I clinical trial of autologous ascites-derived exosomes combined with GM-CSF for colorectal cancer. Mol. Ther.16(4), 782–790 (2008).
- Gehrmann U , HiltbrunnerS, GeorgoudakiAM, KarlssonMC, NaslundTI, GabrielssonS. Synergistic induction of adaptive antitumor immunity by codelivery of antigen with α-galactosylceramide on exosomes. Cancer Res.73(13), 3865–3876 (2013).
- Rao Q , ZuoB, LuZet al. Tumor-derived exosomes elicit tumor suppression in murine hepatocellular carcinoma models and humans in vitro. Hepatology64(2), 456–472 (2016).
- Morishita M , TakahashiY, MatsumotoA, NishikawaM, TakakuraY. Exosome-based tumor antigens-adjuvant co-delivery utilizing genetically engineered tumor cell-derived exosomes with immunostimulatory CpG DNA. Biomaterials111, 55–65 (2016).
- Man K , BrunetMY, JonesMC, CoxSC. Engineered extracellular vesicles: tailored-made nanomaterials for medical applications. Nanomaterials (Basel)10(9), 1838 (2020).
- Hu L , WicklineSA, HoodJL. Magnetic resonance imaging of melanoma exosomes in lymph nodes. Magn. Reson. Med.74(1), 266–271 (2015).
- Qi H , LiuC, LongLet al. Blood exosomes endowed with magnetic and targeting properties for cancer therapy. ACS Nano10(3), 3323–3333 (2016).
- Gang J , YongH, AnYet al. NRP-1 targeted and cargo-loaded exosomes facilitate simultaneous imaging and therapy of glioma in vitro and in vivo. Biomaterials178, 302–316 (2018).
- Khongkow M , YataT, BoonrungsimanS, RuktanonchaiUR, GrahamD, NamdeeK. Surface modification of gold nanoparticles with neuron-targeted exosome for enhanced blood–brain barrier penetration. Sci. Rep.9(1), 8278 (2019).
- Betzer O , PeretsN, AngelAet al. In vivo neuroimaging of exosomes using gold nanoparticles. ACS Nano11(11), 10883–10893 (2017).
- Sen K , SheppeAEF, SinghI, HuiWW, EdelmannMJ, RinaldiC. Exosomes released by breast cancer cells under mild hyperthermic stress possess immunogenic potential and modulate polarization in vitro in macrophages. Int. J. Hyperthermia37(1), 696–710 (2020).
- Sheybani ND , BattsAJ, MathewAS, ThimEA, PriceRJ. Focused ultrasound hyperthermia augments release of glioma-derived extracellular vesicles with differential immunomodulatory capacity. Theranostics10(16), 7436–7447 (2020).
- Jang SC , KimOY, YoonCMet al. Bioinspired exosome-mimetic nanovesicles for targeted delivery of chemotherapeutics to malignant tumors. ACS Nano7(9), 7698–7710 (2013).
- Yang Z , XieJ, ZhuJet al. Functional exosome-mimic for delivery of siRNA to cancer: in vitro and in vivo evaluation. J. Control. Release243, 160–171 (2016).
- Savina A , FurlánM, VidalM, ColomboMI. Exosome release is regulated by a calcium-dependent mechanism in K562 cells. J. Biol. Chem.278(22), 20083–20090 (2003).
- Atkin-Smith GK , TixeiraR, PaoneSet al. A novel mechanism of generating extracellular vesicles during apoptosis via a beads-on-a-string membrane structure. Nat. Commun.6, 7439 (2015).
- Hoang TQ , RamponC, FreyssinetJM, VrizS, Kerbiriou-NabiasD. A method to assess the migration properties of cell-derived microparticles within a living tissue. Biochim. Biophys. Acta1810(9), 863–866 (2011).
- Adams SD , CsereJ, D’angeloGet al. Centrosome amplification mediates small extracellular vesicle secretion via lysosome disruption. Curr. Biol.31(7), 1403–1416 (2021).
- Alvarez-Erviti L , SeowY, SchapiraAHet al. Lysosomal dysfunction increases exosome-mediated α-synuclein release and transmission. Neurobiol. Dis.42(3), 360–367 (2011).
- Wang X , YinX, YangY. Rasal2 suppresses breast cancer cell proliferation modulated by secretory autophagy. Mol. Cell. Biochem.462(1–2), 115–122 (2019).
- Cao J , WangB, TangTet al. Three-dimensional culture of MSCs produces exosomes with improved yield and enhanced therapeutic efficacy for cisplatin-induced acute kidney injury. Stem Cell Res. Ther.11(1), 206 (2020).
- Yang Z , ShiJ, XieJet al. Large-scale generation of functional mRNA-encapsulating exosomes via cellular nanoporation. Nat. Biomed. Eng.4(1), 69–83 (2020).
- Wan Z , ZhaoL, LuFet al. Mononuclear phagocyte system blockade improves therapeutic exosome delivery to the myocardium. Theranostics10(1), 218–230 (2020).
- Piffoux M , SilvaAKA, WilhelmC, GazeauF, TaresteD. Modification of extracellular vesicles by fusion with liposomes for the design of personalized biogenic drug delivery systems. ACS Nano12(7), 6830–6842 (2018).
- Sawant RR , TorchilinVP. Challenges in development of targeted liposomal therapeutics. AAPS J.14(2), 303–315 (2012).
- Sato YT , UmezakiK, SawadaSet al. Engineering hybrid exosomes by membrane fusion with liposomes. Sci. Rep.6, 21933 (2016).
- Silva AK , Kolosnjaj-TabiJ, BonneauSet al. Magnetic and photoresponsive theranosomes: translating cell-released vesicles into smart nanovectors for cancer therapy. ACS Nano7(6), 4954–4966 (2013).
- Tedesco KL , LockhartAC, BerlinJD. The epidermal growth factor receptor as a target for gastrointestinal cancer therapy. Curr. Treat. Options Oncol.5(5), 393–403 (2004).
- Pakkala S , RamalingamSS. Combined inhibition of vascular endothelial growth factor and epidermal growth factor signaling in non-small-cell lung cancer therapy. Clin. Lung Cancer10(Suppl. 1), S17–S23 (2009).
- Ohno S , TakanashiM, SudoKet al. Systemically injected exosomes targeted to EGFR deliver antitumor microRNA to breast cancer cells. Mol. Ther.21(1), 185–191 (2013).
- Wang T , PhilippovichS, MaoJ, VeeduRN. Efficient epidermal growth factor receptor targeting oligonucleotide as a potential molecule for targeted cancer therapy. Int. J. Mol. Sci.20(19), 4700 (2019).
- Martinelli E , DePalma R, OrdituraM, DeVita F, CiardielloF. Anti-epidermal growth factor receptor monoclonal antibodies in cancer therapy. Clin. Exp. Immunol.158(1), 1–9 (2009).
- Woodburn JR . The epidermal growth factor receptor and its inhibition in cancer therapy. Pharmacol. Ther.82(2–3), 241–250 (1999).
- Li Z , ZhaoR, WuXet al. Identification and characterization of a novel peptide ligand of epidermal growth factor receptor for targeted delivery of therapeutics. FASEB J.19(14), 1978–1985 (2005).
- Hung ME , LeonardJN. Stabilization of exosome-targeting peptides via engineered glycosylation. J. Biol. Chem.290(13), 8166–8172 (2015).
- Tamura R , UemotoS, TabataY. Augmented liver targeting of exosomes by surface modification with cationized pullulan. Acta Biomater.57, 274–284 (2017).
- Ying W , RiopelM, BandyopadhyayGet al. Adipose tissue macrophage-derived exosomal miRNAs can modulate in vivo and in vitro insulin sensitivity. Cell171(2), 372 (2017).
- Wan Y , WangL, ZhuCet al. Aptamer-conjugated extracellular nanovesicles for targeted drug delivery. Cancer Res.78(3), 798–808 (2018).
- Liang G , KanS, ZhuY, FengS, FengW, GaoS. Engineered exosome-mediated delivery of functionally active miR-26a and its enhanced suppression effect in HepG2 cells. Int. J. Nanomedicine13, 585–599 (2018).
- Bellavia D , RaimondoS, CalabreseGet al. Interleukin 3-receptor targeted exosomes inhibit in vitro and in vivo chronic myelogenous leukemia cell growth. Theranostics7(5), 1333–1345 (2017).
- Sekar D , ThirugnanasambanthamK, HairulIslam VI, SaravananS. Sequencing approaches in cancer treatment. Cell Prolif.47(5), 391–395 (2014).
- Ciavarella S , MilanoA, DammaccoF, SilvestrisF. Targeted therapies in cancer. BioDrugs24(2), 77–88 (2010).
- Castle PE , MazaM. Prophylactic HPV vaccination: past, present, and future. Epidemiol. Infect.144(3), 449–468 (2016).