Abstract
The vascular supply of tumours and the tumour microenvironment both play an important role when tumours are treated with hyperthermia. Blood flow is one of the major vehicles by which heat is dissipated thus the vascular supply will influence the ability to heat the tumour. It also influences the type of microenvironment that exists within tumours, and it is now well-established that cells existing in areas of oxygen deficiency, nutrient deprivation and acidic conditions are more sensitive to the effect of hyperthermia. The vascular supply and microenvironment are also affected by hyperthermia. In general, mild heat temperatures transiently improve blood flow and oxygenation, while higher hyperthermia temperatures cause vascular collapse and so increase the adverse microenvironmental conditions. Being able to image these vascular and microenvironmental parameters both before and after heating will help in our ability to predict and assess response. Here we review the various techniques that can be applied to supply this information, especially using non-invasive imaging approaches.
Introduction
The establishment of a functional vascular supply is essential for tumour growth, and hence new vessels are constantly being formed in proliferating tumours Citation[1], Citation[2]. However, tumour angiogenesis is highly unregulated and lags behind the rapid tumour cell proliferation. The resulting vasculature also possesses several marked differences from the vasculature encountered in the corresponding healthy tissue from which it was derived Citation[3]. It is chaotic, displaying extensive branching and shunts Citation[4]. Vessels are leaky and lack many of the control mechanisms encountered in normal vasculature Citation[3], Citation[5]. As a consequence, tumour blood flow is highly heterogeneous often resulting in significant tumour areas with decreased oxygen and nutrition supply and low extracellular pH Citation[4], Citation[6], Citation[7]. The tumour vascular supply and the resulting tumour microenvironment play a significant role in the response of tumours to hyperthermia treatment ().
Figure 1. Schematic representation of the tumour vasculature and microenvironment and their role in the response to hyperthermia. The tumour vascular supply controls heat deposition. It also delivers oxygen and nutrients to the tumour, thus controlling the microenvironment, and cells that exist in areas where these are deficient are more sensitive to heat damage. Both the vasculature and microenvironment are also influenced by heat, but this is dependent on the temperature of the hyperthermia treatment.
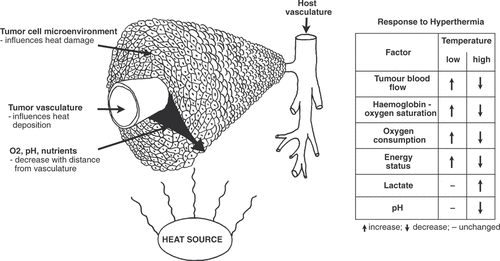
Arguably, the predominant factor governing tumour response to hyperthermia is blood flow. By virtue of convection blood flow constitutes the most potent cooling mechanism. Hence, animal studies have demonstrated a clear connection between tumour blood flow and the ability to heat the tumour Citation[8], Citation[9]. In the latter case it was clearly shown that decreased blood flow resulted in a marked increase in the attainable tumour temperature. However, response is also highly dependent on the local tumour microenvironment, especially with regard to oxygenation and nutritional status, and extracellular pH. In vitro studies exploring the importance of these factors showed that cells incubated under these adverse conditions were more susceptible to heat damage Citation[10–12]. These results have been corroborated by in vivo studies in which the tumour vascular supply was compromised. This has been achieved with physiological modification, such as clamping Citation[13], Citation[14] or physiological agents Citation[15–17]. More recent approaches have utilised so-called vascular disrupting agents (VDAs), which selectively disrupt the established tumour vasculature Citation[18–20]. Such studies showed a clear connection between optimal timing of administration of the vascular modifier for the best enhancement of the hyperthermia response and the time at which maximal shutdown of blood flow was achieved. Additional studies have demonstrated that this improvement in heat response is due partially to a better heating but mostly results from an increase in the heat sensitive adverse microenvironmental conditions within the tumour Citation[15], Citation[28–30].
Although tumour vasculature and the resulting microenvironment influence heat response, these parameters themselves are also affected by heat in return (). When performing mild temperature hyperthermia the initial result is often an improvement in tumour oxygenation status resulting from a heat-induced increase in blood flow in general and in oxy-haemoglobin saturation in particular Citation[19], Citation[24]. At higher temperatures the reverse effect comes into play. Although these higher temperatures may initially lead to an increase in blood flow, vascular damage rapidly ensues with a decrease in tumour blood flow as an immediate result Citation[19], Citation[24]. The decreased blood flow will lead to the adverse conditions described above, but if prolonged may ultimately lead to cell death.
During recent years substantial effort has been put into extending diagnostic imaging to visualisation and quantification of molecular processes Citation[25]. These advances include non-invasive, image-based quantification of vascular parameters, oxygen tension, pH as well as nutritional and energetic status. Knowledge of the spatial distribution of these parameters prior to heat treatment would facilitate prediction of the effect of heating. Post-treatment imaging would additionally enable assessment of the actual efficacy. However, it is important to remember that when applying methods which rely on reference tissue this tissue must be unaffected by the heat treatment, if treatment response is to be measured.
The aim of this review is to present those methods that are currently available for imaging and measuring the pathophysiological parameters involved in hyperthermia treatment in oncology. lists the various methods that can, and often have, been used to monitor the various pathophysiological parameters affected by hyperthermia. For each respective parameter the imaging methods are grouped under those based on magnetic resonance (MR), positron emission tomography (PET), or after techniques. The rationale for such separation is because, as shown in the table, MR- and PET-based methods can actually be used to identify all the relevant heat-related parameters. They are also two of the most commonly used non-invasive techniques in clinical imaging. Below and in the table we have chosen, as a rule, to apply a strict definition of imaging methods, such that only modalities that produce the spatial distribution of the parameter in question have been included. For example, methods such as Xe-clearance and Rb-uptake, which measure global tumour blood flow and perfusion respectively, would not be considered imaging methods.
Table I. List of various parameters of importance for heating and the different methods for imaging them. MR, magnetic resonance; DCE-MRI, dynamic contrast-enhanced magnetic resonance imaging; DSC-MRI, dynamic susceptibility contrast magnetic resonance imaging; BOLD, blood oxygenation level dependent; VSI, vessel size imaging; NIRS, near infrared spectroscopy; EPR, electron paramagnetic resonance; SPECT, single photon emission computed tomography; CAIX, carbonic anhydrase IX.
Tumour vascularisation parameters
Historically, tumour blood flow/perfusion has been measured by a variety of techniques, including microspheres, radioactive and non-radioactive tracer uptake and clearance, laser Doppler flowmetry, and even heat clearance Citation[26], Citation[27]. Unfortunately these procedures are often invasive in nature and do not deliver information on the spatial distribution of the measured parameter. Information about the spatial distribution of, for example, blood vessels, although this method is highly invasive, can be obtained with histological markers. For a non-invasive and spatially sensitive approach there is dynamic contrast-enhanced (DCE-) MRI. This method involves injecting a contrast agent into the host and obtaining signal time curves that show a signal increase related to the concentration of contrast agent and can be converted to concentration time curves Citation[28–30]. These dynamic data can be analysed in each image voxel or the signal can be averaged for regions of interest (ROIs). Both signal time curves and concentration time curves can be analysed semi-quantitatively; the semi-quantitative parameters are typically initial area under the curve (IAUC); maximal (peak) signal enhancement, which reflects perfusion and cell density Citation[31]; initial slope or signal enhancement rate, which relates to perfusion and permeability; and time to peak. IAUC depends on complex and unknown combinations of several physiological parameters including blood volume, perfusion, vessel permeability surface area product, and extravascular extracellular space (EES). However, IAUC can be interpreted as a qualitative measure for the general level of vascularisation Citation[32]. Furthermore, it is easy to calculate, suffers from fewer assumptions than quantitative model parameters, and has shown good reproducibility Citation[33], Citation[34].
Kinetic model analysis further separates the physiological parameters and yield quantitative measures. This approach requires either a priori estimates of the arterial input function or a direct measurement. Tofts et al. Citation[35] standardised quantities and symbols in the general kinetic model based on the model of Kety Citation[36] to a widely used model. The estimated parameters are: Ktrans, the transfer constant from plasma to the EES; kep, the rate constant from here back to plasma; ve, the volume fraction of EES. The general kinetic model assumes that the blood plasma volume fraction, vp, is 0, but vp can be included and assessed in the model Citation[35].
Ktrans depends on both blood flow and the permeability surface area product because these factors limit the extravasation. In the flow limited condition where permeability is high Ktrans reflects mainly blood flow, and in the permeability limited condition, Ktrans reflects mainly the permeability surface area product Citation[35]. More complicated models separate perfusion and permeability, for example, the model proposed by Lawrence and Lee Citation[37].
Another MR approach utilises the variations in magnetic susceptibility between blood and tissue. The susceptibility imaging methods are based on the transverse relaxation rates, R2 and , which are increased by common intravascular contrast agents. The resulting gradient echo and spin echo signals have been explored analytically, by simulations, and experimentally with the aim of understanding and utilising the susceptibility contrast properties. This has been utilised for measurements of blood flow, blood volume and mean transit time by first pass tracer kinetics Citation[38–40].
DCE-MRI provides information on vascular permeability. Ktrans depends on perfusion and permeability surface area (PSA) and can under certain conditions be assumed to reflect either perfusion or PSA alone Citation[35]. Contrast agent size influences the kinetics because of vessels’ permeability for different particle sizes. Using large contrast particles, Ktrans depends mostly on PSA. Many studies are performed with smaller contrast agents such as Gd-DTPA, which extravasates in normal tissue such as muscle, and often to a higher degree in the often highly permeable tumour vessels. Larger contrast agents, which do not extravasate in normal tissue, may extravasate in tumour tissue, but showing slower kinetics such that a longer dynamic scan may be appropriate.
The finding of specific differences in vessel size dependency of R2 and in susceptibility imaging has led to the MRI method vessel size imaging (VSI) Citation[41–43]. Δ
, the
change caused by the contrast agent, is proportional to the blood volume independently of the vessel sizes when these are at capillary size or above, whereas ΔR2 is most sensitive to smaller vessels. By these quantities, VSI quantitatively estimates blood volume and mean vessel size R. VSI can be performed on cerebral dynamic susceptibility contrast MRI data using standard gadolinium chelates Citation[42] or iron oxide contrast agent Citation[44], or in other organs using a contrast agent that remains intravascular sufficiently long to allow R2 and
measurements at steady state Citation[41], Citation[43], Citation[45]. Besides contrast agent administration, the blood content of the paramagnetic deoxyhaemoglobin can provide vascular information through the relaxation rate
in blood oxygenation level-dependent (BOLD) imaging Citation[46–48]. In VSI,
is measured and can be analysed separately or compared with VSI parameters obtained with contrast agents.
Non-invasive blood flow imaging can also be obtained with dynamic PET scans using 15O-labelled water and has been applied in some clinical studies. PET-derived tumour perfusion estimates have shown good reproducibility Citation[49] and the observation that highly perfused voxels do not retain the PET hypoxia marker 18F-EF5 supports its potential role in blood flow measurements Citation[50]. Qualitative PET-based perfusion assessment requires an accurate input function which can be obtained by continuous blood withdrawal using an image-derived input function Citation[50].
Several of these vascular imaging techniques have already been used in connection with hyperthermia. Both DCE-MRI Citation[51] and PET Citation[52] have been utilised to observe the effects of heating tumours in both animals and humans. In the latter O-PET was used to measure perfusion as well as water diffusion. It was demonstrated that hyperthermia applied for at least 1 h would significantly enhance water diffusion, leading the authors to conclude that this would possibly apply for tumour oxygenation as well. Recently, two studies have attempted to use DCE-MRI measurements in canines Citation[53] and humans Citation[54] to predict response to therapy, albeit with heat in combination with radiation or neoadjuvant chemotherapy, respectively. Computed tomography can be used to monitor many of the same kinetic parameters that are estimated using DCE-MRI, for example Ktrans and the EES which has been done to estimate the response of pancreatic tumours to chemotherapy and radiation therapy Citation[55]. Finally, ultrasound can, especially in combination with contrast agents, be used to provide qualitative estimates of tumour perfusion and its response to radiation therapy Citation[56].
Estimates of tumour hypoxia/oxygenation
A large number of studies have been performed in order to elucidate the relationship between tumour oxygenation and response to heat treatment. Historically this has been performed using invasive micro-electrodes Citation[57–62] to measure actual oxygen tension. Recently a number of non-invasive imaging methods have been developed to measure both oxygen transport and tension parameters, based predominantly on MRI, PET and SPECT. The deoxyhaemoglobin level can also be manipulated by breathing different gasses (e.g., oxygen or carbogen) to indicate vessels, which respond to this Citation[45], Citation[46]. This shows the potential for monitoring blood oxygenation changes without the administration of contrast agents.
A fully quantitative MRI-based method for measuring oxygen tension uses 19F as imaging nucleus. The longitudinal relaxation rate of 19F has been shown to depend on the local oxygen tension Citation[63]. Although this relationship is dependent on the specific fluorine-containing molecule, the dependency is generally linear. A comprehensive list of such molecules and the corresponding coefficients can be found in Yu et al. Citation[64]. Hexafluorobenzene (HFB) has been applied extensively in tumour studies Citation[65–68]. The primary weakness of this approach is the low concentration of the imaged nucleus and consequently low signal. In the case of HFB, this has been remedied by intra-tumoural injection Citation[69], however consecutive systemic intravenous injections on several days before imaging has also been demonstrated to result in a significant accumulation of fluorinated molecules within the tumour. Finally, DCE-MRI has been employed in conjunction with an extended Krogh-model to provide qualitative measurements of oxygen tension Citation[70].
Probably the most direct non-invasive method for monitoring tumour hypoxia involves PET. This primarily involves the use of hypoxia-selective nitroimidazole-based agents (e.g. 18F-fluoromisonidazole, 18F-fluoroazomycin arabinoside), which are reduced enzymatically and trapped when cellular pO2 drops below ∼10 mmHg Citation[71], Citation[72]. In addition to its non-invasive nature, a major advantage of PET is that it can measure global tumour hypoxia status as well as provide three-dimensional maps of regional tumour oxygenation at a resolution down to 100 µL. The major weakness of nitroimidazoles is their slow kinetics, and accurate assessment of hypoxia is therefore only possible several hours after tracer administration, when sufficient tracer has been retained in hypoxic cells and contaminating unbound tracer has cleared from the circulation (for a review on pitfalls in PET hypoxia imaging see Busk et al. Citation[73]). Nitroimidazole-based hypoxia PET scans may be particularly useful for identification of patients with hypoxic (and possible acidic) tumours with reduced sensitivity towards radio and/or chemotherapy, for which a therapeutic benefit from complementary high-temperature hyperthermic treatment is most likely. However, the ability of PET hypoxia imaging to actually identify tumours suitable for hyperthermic treatment has never been tested in patients or animal experimental models, but clearly such studies would be highly valuable.
Due to the aforementioned slow tracer distribution, the usefulness of nitroimidazole-based hypoxia PET to assess the magnitude and durability of the increased oxygenation following mild temperature hyperthermia is more questionable since most studies suggest that enhanced oxygenation in heated tumours is short-lived (minutes to a few hours). In contrast, invasive immunohistochemical quantification of cellular retention of unlabelled nitroimidazole hypoxia markers has been used to assess the magnitude and kinetics of mild-temperature hyperthermia-induced reoxygenation in an animal model. By sequential administration of the two comparable but separable to the nitroimidazole-based hypoxia markers pimonidazole and EF5 before and after treatment, respectively, Sun and colleagues Citation[74] were able to show an improvement in oxygenation in a human colon adenocarcinoma xenograft tumour model 1 h after hyperthermic treatment at 41°C, but the effect had disappeared by 6 h.
Copper-diacetyl-bis (N4-methylthiosemicarbazone) (ATSM) complexes belong to another group of PET hypoxia tracers which has been shown to accumulate in hypoxic cells in vitro and in vivo Citation[75], Citation[76], by a poorly understood mechanism. 64Cu-ATSM, the most widely used compound, distributes much more rapidly than the nitroimidazole-based agents, and it was shown in a recent study that 64Cu-ATSM PET scans are able to detect even short-lived changes in tumour hypoxia elicited by local heating to 41.5°C for 45 min Citation[77]. Complementary electrode measurements confirmed that the PET-observable changes were correlated with real changes in tumour oxygenation Citation[77]. However, the hypoxia specificity of 64Cu-ATSM is apparently tumour-type dependent Citation[78], Citation[79], and furthermore, it was shown by Matsomoto and co-workers Citation[80] that 64Cu-ATSM PET was unable to detect changes in tumour oxygenation induced by exposing tumour-bearing mice to hypo- or hyperoxic gas mixtures. Accordingly, the general value of 64Cu-ATSM as a means to detect transient changes in tumour oxygenation needs to be verified in a broad selection of preclinical tumour models using various interventions to modify tumour hypoxia.
Radioactively labelled tracers have been used to measure oxygen tension for a number for years using in general iodoazomycin containing molecules such as iodoazomycin arabinoside (IAZA). IAZA labelled with 123I and 125I has successfully been utilised to measure tumour hypoxia Citation[81–83]. Minimally invasive quantitative measurements of oxygenation can also be performed in blood with near-infrared spectroscopy Citation[84] and tissue electron paramagnetic resonance Citation[85]. However, none of these techniques have been combined with hyperthermia in oncology.
Measuring pH
The importance of tumour pH in determining response to heating Citation[10–12] has resulted in numerous attempts to monitor it. Most of these have involved using invasive electrode-based measurements, which only estimate extracellular pH Citation[4]. Intracellular pH values can be obtained using MR-based techniques Citation[4] utilising either endogenous agents or following the administration of exogenous agents Citation[86–88]. One of the more popular approaches for estimating pH is based on 31P magnetic resonance spectroscopy (MRS) of inorganic phosphate, and indeed this method has been used to monitor pH distributions before and after heat treatment Citation[89], Citation[90]. Although, strictly speaking MRS is not an imaging method, there are sequences facilitating spatially selective measurements. Tumour pH can also be imaged with magnetisation transfer (MT), which exploits the differences in dephasing rates between free water and water bound by hydrogen bonds to macromolecules such as lipids and proteins, and the subsequent effects on the MR-signal when magnetisation is transferred from the bound pool to the liquid/free pool Citation[91]. Alternatively, one can inject probes with either pH-sensitive 19F or 1H resonances. None of these other approaches have yet been combined with hyperthermia. There is also the possibility to utilise PET to non-invasively image pH. Carbonic anhydrase IX (CAIX) is a pH-responsive protein that is often up-regulated in the adverse microenvironment. Antibodies to CAIX have now been developed which are detectable by PET Citation[92] making pH assessment feasible.
Estimating bioenergetic status
Another important parameter involved in the response to hyperthermia is the metabolic or energy status of tumours. Glucose or lactate levels, for example, will influence pH. Heat itself has also been shown to dramatically change metabolic and energy status within tumours Citation[93]. Many of these parameters can be measured with MRS. 1H spectroscopy allows identification of creatine, phosphocreatine, lactate and glucose, while with 31P spectroscopy, information about phosphocreatine, ATP, ADP, and inorganic phosphorous can be assessed. Investigation of tumour glycolysis through glucose and lactate is possible by the use of 13C-labelled glucose Citation[94].
A surrogate marker of glucose metabolism is fluorodeoxyglucose (FDG) and this is the most commonly used PET tracer in oncology with a huge role in diagnosis, prognosis and treatment-efficacy monitoring (for a review see Hoh Citation[95]). FDG takes advantage of tumour cells’ excessive dependency on glycolysis as a means to produce energy (referred to as aerobic glycolysis or the Warburg effect). Since most glucose is converted to lactic acid in tumour cells, FDG-PET may also provide indirect information on the acid-base status of tumours, especially when combined with blood flow measurements.
Functional imaging with FDG-PET may be useful for rapid assessment of tumour response to treatment, long before morphological changes are evident. In particular, many studies have shown that reduced FDG retention shortly after initiation of a treatment course with radio- and/or chemotherapy correlates well with treatment sensitivity, which allows treatment modification in non-responders (reviewed by Citation[96], Citation[97]). A few studies have examined the usefulness of FDG-PET to predict tumour response in patients treated with hyperthermia and radiotherapy. Ishii and co-workers Citation[98] showed that early evaluation by FDG-microPET, especially 24 h after treatment, is useful to predict the effects of combined radiotherapy (10 Gy) and hyperthermia (43°C for 1 h) in tumour-bearing rabbits. Furthermore, in a clinical study it was concluded that FDG-PET performed before and two weeks after initiation of combined chemoradiation and hyperthermia in patients with oesophageal cancer were able to separate responders and non-responders with reasonable accuracy Citation[99].
A more invasive method for assessing bioenergetic status involves using the bioluminescence technique Citation[100]. This involves exposing frozen tissue selections to a cooled mixture of enzymes and co-enzymes which link the substrate of interest to a luciferase reaction that occurs and can be imaged on warming. Although invasive it does give microenvironmental metabolite concentrations and has been used to monitor the effect of hyperthermia Citation[93].
Conclusions
Hyperthermia is an effective cancer therapy when used in combination with other more conventional treatments, especially radiotherapy Citation[101], but it is has not become an established form of therapy. What is now needed are techniques that are reliable, accurate, easy to apply to patients on a routine basis, and preferably non-invasive for monitoring the more important factors that can both influence response to heat and are indicative of the efficacy of the heating. Since the tumour vascular supply and microenvironment play such an important role in determining the response to heat and are themselves influenced by heating, utilising methods that can actually measure these parameters would clearly be the way to proceed. There are a number of ways that this can be done non-invasively and of these, MRI and PET would certainly seem to be the methods of choice, especially since they are already well-established clinical procedures used routinely in hospitals. Both techniques have been used to some limited extent in connection with hyperthermia, but their full potential in this context has not been achieved. Clearly, additional studies with these procedures are required so that we take full advantage of the beneficial use of hyperthermia in a therapeutic setting.
Acknowledgements
This work has been supported by grants from the Danish Cancer Society and the Danish Research Agency.
Declaration of interest: The authors report no conflicts of interest. The authors alone are responsible for the content and writing of the paper.
References
- Folkman J. Tumor angiogenesis: Therapeutic implications. N Engl J Med 1971; 285: 1182–1186
- Folkman J. Role of angiogenesis in tumor growth and metastasis. Semin Oncol 2002; 29: 15–18
- Vaupel P. Tumor microenvironmental physiology and its implications for radiation oncology. Semin Radiat Oncol 2004; 14: 198–206
- Vaupel P, Kallinowski F, Okunieff P. Blood flow, oxygen and nutrient supply, and metabolic microenvironment of human tumors: A review. Cancer Research 1989; 49: 6449–6465
- Gillies RJ, Schornack PA, Secomb TW, Raghunand N. Causes and effects of heterogeneous perfusion in tumors. Neoplasia 1999; 1: 197–207
- Moulder JE, Rockwell S. Tumor hypoxia: Its impact on cancer therapy. Cancer Metastasis Rev 1987; 5: 313–341
- Vaupel P, Harrison L. Tumor hypoxia: Causative factors, compensatory mechanisms, and cellular response. Oncologist 2004; 9: S4–S9
- Jain RK, Grantham FH, Gullino PM. Blood flow and heat transfer in Walker 256 mammary carcinoma. J Natl Cancer Inst 1979; 62: 927–933
- Patterson J, Strang R. The role of blood flow in hyperthermia. Int J Radiat Oncol Biol Phys 1979; 5: 235–241
- Gerweck LE, Nygaard TG, Burlett M. Response of cells to hyperthermia under acute and chronic hypoxic conditions. Cancer Res 1979; 39: 966–972
- Overgaard J, Bichel P. The influence of hypoxia and acidity on the hyperthermic response of malignant cells in vitro. Radiology 1977; 123: 511–514
- Overgaard J, Nielsen OS. The role of tissue environmental factors on the kinetics and morphology of tumor cells exposed to hyperthermia. Ann NY Acad Sci 1980; 335: 254–280
- Thrall DE, Gillette EL, Dewey WC. Effect of heat and ionizing radiation on normal and neoplastic tissue of the C3H mouse. Radiat Res 1975; 63: 363–377
- Hill SA, Denekamp J. The effect of vascular occlusion on the thermal sensitization of a mouse tumour. Br J Radiol 1978; 51: 997–1002
- Horsman MR, Christensen KL, Overgaard J. Hydralazine-induced enhancement of hyperthermic damage in a C3H mammary carcinoma in vivo. Int J Hyperthermia 1989; 5: 123–136
- Prescott DM, Samulski TV, Dewhirst MW, Page RL, Thrall DE, Dodge RK, Oleson JR. Use of nitroprusside to increase tissue temperature during local hyperthermia in normal and tumor-bearing dogs. Int J Radiat Oncol BiolPhys 1992; 23: 377–385
- Urano M, Montoya V, Booth A. Effect of hyperglycemia on the thermal response of murine normal and tumor tissues. Cancer Res 1983; 43: 453–455
- Horsman MR, Murata R. Combination of vascular targeting agents with thermal or radiation therapy. Int J Radiat Oncol Biol Phys 2002; 54: 1518–1523
- Horsman MR. Tissue physiology and the response to heat. Int J Hyperthermia 2006; 22: 197–203
- Horsman MR. Angiogenesis and vascular targeting: Relevance for hyperthermia. Int J Hyperthermia 2008; 24: 57–65
- Horsman MR, Murata R, Breidahl T, Nielsen FU, Maxwell RJ, Stodkiled-Jorgensen H, Overgaard J. Combretastatins novel vascular targeting drugs for improving anti-cancer therapy. Combretastatins and conventional therapy. Adv Exp Med Biol 2000; 476: 311–323
- Murata R, Overgaard J, Horsman MR. Combretastatin A-4 disodium phosphate: A vascular targeting agent that improves that improves the anti-tumor effects of hyperthermia, radiation, and mild thermoradiotherapy. Int J Radiat Oncol Biol Phys 2001; 51: 1018–1024
- Hokland SL, Horsman MR. The new vascular disrupting agent combretastatin-A1-disodium-phosphate (OXi4503) enhances tumour response to mild hyperthermia and thermoradiosensitization. Int J Hyperthermia 2007; 23: 599–606
- Vaupel P, Kallinowski F. Physiological effects of hyperthermia. Recent results in cancer research, C Streffer. Springer, Heidelberg, Berlin 1987; 71–109
- Weissleder R, Mahmood U. Molecular imaging. Radiology 2001; 219: 316–333
- Appelgren LK. Methods of recording tumor blood flow. Tumor blood circulation: Angiogenesis, vascular morphology and blood flow of experimental and human tumors, H-I Peterson. CRC Press, Boca Raton 1979; 87–101
- Vaupel P, Okunieff P, Kluge M. Response of tumour red blood cell flux to hyperthermia and/or hyperglycaemia. Int J Hyperthermia 1989; 5: 199–210
- Brix G, Semmler W, Port RE, Schad LR, Layer G, Lorenz WJ. Pharmacokinetic parameters in CNS Gd-DTPA enhanced MR imaging. J Comput Assist Tomog 1991; 15: 621–628
- Larsson HB, Stubgaard M, Frederiksen JL, Jensen M, Henriksen O, Paulson OB. Quantitation of blood–brain barrier defect by magnetic resonance imaging and gadolinium-DTPA in patients with multiple sclerosis and brain tumors. Magn Reson Med 1990; 16: 117–131
- Tofts PS, Kermode AG. Measurement of the blood–brain barrier permeability and leakage space using dynamic MR imaging. Magn Reson Med 1991; 17: 357–367
- Lyng H, Vorren AO, Sundfor K, Taksdal I, Lien HH, Kaalhus O, Rofstad EK. Assessment of tumor oxygenation in human cervical carcinoma by use of dynamic Gd-DTPA-enhanced MR imaging. J Magn Reson Imag 2001; 14: 750–756
- Leach MO, Brindle KM, Evelhoch JL, Griffiths JR, Horsman MR, Jackson A, Jayson G, Judson IR, Knopp MV, Maxwell RJ. Assessment of antiangiogenic and antivascular therapeutics using MRI: recommendations for appropriate methodology for clinical trials. Brit J Radiol 2003; 76: S87–S91
- Evelhoch JL, LoRusso PM, He Z, DelProposto Z, Polin L, Corbett TH, Langmuir P, Wheeler C, Stone A, Leadbetter J. Magnetic resonance imaging measurements of the response of murine and human tumors to the vascular-targeting agent ZD6126. Clin Cancer Res 2004; 10: 3650–3657
- Galbraith SM, Lodge MA, Taylor NJ, Rustin GJ, Bentzen S, Stirling JJ, Padhani AR. Reproducibility of dynamic contrast-enhanced MRI in human muscle and tumours: Comparison of quantitative and semi-quantitative analysis. NMR Biomed 2002; 15: 132–142
- Tofts PS, Brix G, Buckley DL, Evelhoch JL, Henderson E, Knopp MV, Larsson HB, Lee TY, Mayr NA, Parker GJ, et al. Estimating kinetic parameters from dynamic contrast-enhanced T(1)-weighted MRI of a diffusable tracer: Standardized quantities and symbols. J Magn Reson Imag 1999; 10: 223–232
- Kety SS. Blood-exchange methods. Theory of blood-tissue exchange and its application to measurement of blood flow. Methods Med Res 1960; 8: 223–227
- Lawrence KSS, Lee TY. An adiabatic approximation to the tissue homogeneity model for water exchange in the brain: I. Theoretical derivation. J Cereb Blood Flow Metab 1998; 18: 1365–1377
- Boxerman JL, Hamberg LM, Rosen BR, Weisskoff RM. MR contrast due to intravascular magnetic susceptibility perturbations. Magn Reson Med 1995; 34: 555–566
- Kjolby BF, Ostergaard L, Kiselev VG. Theoretical model of intravascular paramagnetic tracers effect on tissue relaxation. Magn Reson Med 2006; 56: 187–197
- Ostergaard L, Sorensen AG, Kwong KK, Weisskoff RM, Gyldensted C, Rosen BR. High resolution measurement of cerebral blood flow using intravascular tracer bolus passages. Part II: Experimental comparison and preliminary results. Magn Reson Med 1996; 36: 726–736
- Dennie J, Mandeville JB, Boxerman JL, Packard SD, Rosen BR, Weisskoff RM. NMR imaging of changes in vascular morphology due to tumor angiogenesis. Magn Reson Med 1998; 40: 793–799
- Kiselev VG, Strecker R, Ziyeh S, Speck O, Hennig J. Vessel size imaging in humans. Magn Reson Med 2005; 53: 553–563
- Tropres I, Lamalle L, Peoc'h M, Farion R, Usson Y, Decorps M, Remy C. In vivo assessment of tumoral angiogenesis. Magn Reson Med 2004; 51: 533–541
- Quarles CC, Schmainda KM. Assessment of the morphological and functional effects of the anti-angiogenic agent SU11657 on 9L gliosarcoma vasculature using dynamic susceptibility contrast MRI. Magn Reson Med 2007; 57: 680–687
- Robinson SP, Rijken PF, Howe FA, McSheehy PM, van der Sanden BP, Heerschap A, Stubbs M, van der Kogel AJ, Griffiths JR. Tumor vascular architecture and function evaluated by non-invasive susceptibility MRI methods and immunohistochemistry. J Magn Reson Imag 2003; 17: 445–454
- Abramovitch R, Dafni H, Smouha E, Benjamin LE, Neeman M. In vivo prediction of vascular susceptibility to vascular susceptibility endothelial growth factor withdrawal: Magnetic resonance imaging of C6 rat glioma in nude mice. Cancer Res 1999; 59: 5012–5016
- Kostourou V, Robinson SP, Cartwright JE, Whitley GS. Dimethylarginine dimethylaminohydrolase I enhances tumour growth and angiogenesis. Br J Cancer 2002; 87: 673–680
- Robinson SP, McIntyre DJ, Checkley D, Tessier JJ, Howe FA, Griffiths JR, Ashton SE, Ryan AJ, Blakey DC, Waterton JC. Tumour dose response to the antivascular agent ZD6126 assessed by magnetic resonance imaging. Br J Cancer 2003; 88: 1592–1597
- Lodge MA, Jacene HA, Pili R, Wahl RL. Reproducibility of tumor blood flow quantification with 15O-water PET. J Nucl Med 2008; 49: 1620–1627
- Komar G, Seppanen M, Eskola O, Lindholm P, Gronroos TJ, Forsback S, Sipila H, Evans SM, Solin O, Minn H. 18F-EF5: A new PET tracer for imaging hypoxia in head and neck cancer. J Nucl Med 2008; 49: 1944–1951
- Ludemann L, Wust P, Gellermann J. Perfusion measurement using DCE-MRI: Implications for hyperthermia. Int J Hyperthermia 2008; 24: 91–96
- Ludemann L, Sreenivasa G, Amthauer H, Michel R, Gellermann J, Wust P. Use of H(2) (15)O-PET for investigating perfusion changes in pelvic tumors due to regional hyperthermia. Int J Hyperthermia 2009; 25: 299–308
- Viglianti BL, Lora-Michiels M, Poulson JM, Lan L, Yu D, Sanders L, Craciunescu O, Vujaskovic Z, Thrall DE, Macfall J, et al. Dynamic contrast-enhanced magnetic resonance imaging as a predictor of clinical outcome in canine spontaneous soft tissue sarcomas treated with thermoradiotherapy. Clin Cancer Res 2009; 15: 4993–5001
- Craciunescu OI, Blackwell KL, Jones EL, Macfall JR, Yu D, Vujaskovic Z, Wong TZ, Liotcheva V, E LR, Prosnitz LR, et al. DCE-MRI parameters have potential to predict response of locally advanced breast cancer patients to neoadjuvant chemotherapy and hyperthermia: A pilot study. Int J Hyperthermia 2009; 1–11
- Park MS, Klotz E, Kim MJ, Song SY, Park SW, Cha SW, Lim JS, Seong J, Chung JB, Kim KW. Perfusion CT: Noninvasive surrogate marker for stratification of pancreatic cancer response to concurrent chemo- and radiation therapy. Radiology 2009; 250: 110–117
- Delorme S, Krix M. Contrast-enhanced ultrasound for examining tumor biology. Cancer Imaging 2006; 6: 148–152
- Shakil A, Osborn JL, Song CW. Changes in oxygenation status and blood flow in a rat tumor model by mild temperature hyperthermia. Int J Radiat Oncol Biol Phys 1999; 43: 859–865
- Horsman MR, Overgaard J. Can mild hyperthermia improve tumour oxygenation?. Int J Hyperthermia 1997; 13: 141–147
- Iwata K, Shakil A, Hur WJ, Makepeace CM, Griffin RJ, Song CW. Tumour pO2 can be increased markedly by mild hyperthermia. Br J Cancer Suppl 1996; 27: S217–221
- Kallinowski F, Zander R, Hoeckel M, Vaupel P. Tumor tissue oxygenation as evaluated by computerized-pO2-histography. Int J Radiat Oncol Biol Phys 1990; 19: 953–961
- Song CW, Shakil A, Osborn JL, Iwata K. Tumour oxygenation is increased by hyperthermia at mild temperatures. Int J Hyperthermia 1996; 12: 367–373
- Okajima K, Griffin RJ, Iwata K, Shakil A, Song CW. Tumor oxygenation after mild-temperature hyperthermia in combination with carbogen breathing: Dependence on heat dose and tumor type. Radiat Res 1998; 149: 294–299
- Thomas SR. The biomedical applications of Fluorine-19 NMR, CL Partain, RR Price, JA Patton, MV Kulkarni, AEJ James. W.B. Saunders, London 1988
- Yu JX, Kodibagkar VD, Cui W, Mason RP. 19F: A versatile reporter for non-invasive physiology and pharmacology using magnetic resonance. Curr Med Chem 2005; 12: 819–848
- Mason RP, Rodbumrung W, Antich PP. Hexafluorobenzene: A sensitive 19F NMR indicator of tumor oxygenation. NMR Biomed 1996; 9: 125–134
- Hunjan S, Mason RP, Constantinescu A, Peschke P, Hahn EW, Antich PP. Regional tumor oximetry: 19F NMR spectroscopy of hexafluorobenzene. Int J Radiat Oncol Biol Phys 1998; 41: 161–171
- Le D, Mason RP, Hunjan S, Constantinescu A, Barker BR, Antich PP. Regional tumor oxygen dynamics: 19F PBSR EPI of hexafluorobenzene. Magn Reson Imag 1997; 15: 971–981
- Mason RP, Constantinescu A, Hunjan S, Le D, Hahn EW, Antich PP, Blum C, Peschke P. Regional tumor oxygenation and measurement of dynamic changes. Radiat Res 1999; 152: 239–249
- Xia M, Kodibagkar V, Liu H, Mason RP. Tumour oxygen dynamics measured simultaneously by near-infrared spectroscopy and 19F magnetic resonance imaging in rats. Phys Med Biol 2006; 51: 45–60
- Wang Z, Su MY, Nalcioglu O. Applications of dynamic contrast enhanced MRI in oncology: Measurement of tumor oxygen tension. Technol Cancer Res Treat 2002; 1: 29–38
- Rasey JS, Nelson NJ, Chin L, Evans ML, Grunbaum Z. Characteristics of the binding of labeled fluoromisonidazole in cells in vitro. Radiat Res 1990; 122: 301–308
- Busk M, Horsman MR, Jakobsen S, Bussink J, van der Kogel A, Overgaard J. Cellular uptake of PET tracers of glucose metabolism and hypoxia and their linkage. Eur J Nucl Med Mol Imag 2008; 35: 2294–2303
- Busk M, Horsman MR, Overgaard J. Resolution in PET hypoxia imaging: Voxel size matters. Acta Oncol 2008; 47: 1201–1210
- Sun X, Li XF, Russell J, Xing L, Urano M, Li GC, Humm JL, Ling CC. Changes in tumor hypoxia induced by mild temperature hyperthermia as assessed by dual-tracer immunohistochemistry. Radiother Oncol 2008; 88: 269–276
- Lewis JS, McCarthy DW, McCarthy TJ, Fujibayashi Y, Welch MJ. Evaluation of 64Cu-ATSM in vitro and in vivo in a hypoxic tumor model. J Nucl Med 1999; 40: 177–183
- Dence CS, Ponde DE, Welch MJ, Lewis JS. Autoradiographic and small-animal PET comparisons between (18)F-FMISO, (18)F-FDG, (18)F-FLT and the hypoxic selective (64)Cu-ATSM in a rodent model of cancer. Nucl Med Biol 2008; 35: 713–720
- Myerson RJ, Singh AK, Bigott HM, Cha B, Engelbach JA, Kim J, Lamoreaux WT, Moros E, Novak P, Sharp TL, et al. Monitoring the effect of mild hyperthermia on tumour hypoxia by Cu-ATSM PET scanning. Int J Hyperthermia 2006; 22: 93–115
- Vavere AL, Lewis JS. Examining the relationship between Cu-ATSM hypoxia selectivity and fatty acid synthase expression in human prostate cancer cell lines. Nucl Med Biol 2008; 35: 273–279
- O'Donoghue JA, Zanzonico P, Pugachev A, Wen B, Smith-Jones P, Cai S, Burnazi E, Finn RD, Burgman P, Ruan S, et al. Assessment of regional tumor hypoxia using 18F-fluoromisonidazole and 64Cu(II)-diacetyl-bis(N4-methylthiosemicarbazone) positron emission tomography: Comparative study featuring microPET imaging, Po2 probe measurement, autoradiography, and fluorescent microscopy in the R3327-AT and FaDu rat tumor models. Int J Radiat Oncol Biol Phys 2005; 61: 1493–1502
- Matsumoto K, Szajek L, Krishna MC, Cook JA, Seidel J, Grimes K, Carson J, Sowers AL, English S, Green MV, et al. The influence of tumor oxygenation on hypoxia imaging in murine squamous cell carcinoma using [64Cu]Cu-ATSM or [18F]Fluoromisonidazole positron emission tomography. Int J Oncol 2007; 30: 873–881
- Chapman JD, Coia LR, Stobbe CC, Engelhardt EL, Fenning MC, Schneider RF. Prediction of tumour hypoxia and radioresistance with nuclear medicine markers. Br J Cancer Suppl 1996; 27: S204–S208
- Parliament MB, Chapman JD, Urtasun RC, McEwan AJ, Golberg L, Mercer JR, Mannan RH, Wiebe LI. Non-invasive assessment of human tumour hypoxia with 123I-iodoazomycin arabinoside: Preliminary report of a clinical study. Br J Cancer 1992; 65: 90–95
- Urtasun RC, Parliament MB, McEwan AJ, Mercer JR, Mannan RH, Wiebe LI, Morin C, Chapman JD. Measurement of hypoxia in human tumours by non-invasive spect imaging of iodoazomycin arabinoside. Br J Cancer Suppl 1996; 27: S209–S212
- Liu H, Song Y, Worden KL, Jiang X, Constantinescu A, Mason RP. Noninvasive investigation of blood oxygenation dynamics of tumors by near-infrared spectroscopy. Appl Opt 2000; 39: 5231–5243
- Elas M, Williams BB, Parasca A, Mailer C, Pelizzari CA, Lewis MA, River JN, Karczmar GS, Barth ED, Halpern HJ. Quantitative tumor oxymetric images from 4D electron paramagnetic resonance imaging (EPRI): Methodology and comparison with blood oxygen level-dependent (BOLD) MRI. Magn Reson Med 2003; 49: 682–691
- Gillies RJ, Raghunand N, Garcia-Martin ML, Gatenby RA. pH imaging. A review of pH measurement methods and applications in cancers. IEEE Eng Med Biol Mag 2004; 23: 57–64
- van der Toorn A, Sykova E, Dijkhuizen RM, Vorisek I, Vargova L, Skobisova E, van Lookeren Campagne M, Reese T, Nicolay K. Dynamic changes in water ADC, energy metabolism, extracellular space volume, and tortuosity in neonatal rat brain during global ischemia. Magn Reson Med 1996; 36: 52–60
- Remy C, Albrand JP, Benabid AL, Decorps M, Jacrot M, Riondel J, Foray MF. In vivo 31P nuclear magnetic resonance studies of T1 and T2 relaxation times in rat brain and in rat brain tumors implanted to nude mice. Magn Reson Med 1987; 4: 144–152
- Vaupel P, Okunieff P, Neuringer LJ. In vivo 31P-NMR spectroscopy of murine tumours before and after localized hyperthermia. Int J Hyperthermia 1990; 6: 15–31
- Sijens PE, Bovee WM, Koole P, Schipper J. Phosphorus NMR study of the response of a murine tumour to hyperthermia as a function of treatment time and temperature. Int J Hyperthermia 1989; 5: 351–357
- Ward KM, Balaban RS. Determination of pH using water protons and chemical exchange dependent saturation transfer (CEST). Magn Reson Med 2000; 44: 799–802
- Lawrentschuk N, Lee FT, Jones G, Rigopoulos A, Mountain A, O'Keefe G, Papenfuss AT, Bolton DM, Davis ID, Scott AM. Investigation of hypoxia and carbonic anhydrase IX expression in a renal cell carcinoma xenograft model with oxygen tension measurements and (124)I-cG250 PET/CT. Urol Oncol 2009
- Kelleher DK, Engel T, Vaupel PW. Changes in microregional perfusion, oxygenation, ATP and lactate distribution in subcutaneous rat tumours upon water-filtered IR-A hyperthermia. Int J Hyperthermia 1995; 11: 241–255
- Nielsen FU, Daugaard P, Bentzen L, Stodkilde-Jorgensen H, Overgaard J, Horsman MR, et al. Effect of changing tumor oxygenation on glycolytic metabolism in a murine C3H mammary carcinoma assessed by in vivo nuclear magnetic resonance spectroscopy. Cancer Res 2001; 61: 5318–5325
- Hoh CK. Clinical use of FDG PET. Nucl Med Biol 2007; 34: 737–742
- Weber WA. Assessing tumor response to therapy. J Nucl Med 2009; 50: S1–10
- Herrmann K, Krause BJ, Bundschuh RA, Dechow T, Schwaiger M. Monitoring response to therapeutic interventions in patients with cancer. Semin Nucl Med 2009; 39: 210–232
- Ishii K, Hosono MN, Wada Y, Maeda M, Kondo S, Takada Y, Tada T, Okamura T, Watanabe Y, Inoue Y. Usefulness of FDG-microPET for early evaluation of therapeutic effects on VX2 rabbit carcinoma. Ann Nucl Med 2006; 20: 123–130
- Westerterp M, Omloo JM, Sloof GW, Hulshof MC, Hoekstra OS, Crezee H, Boellaard R, Vervenne WL, ten Kate FJ, van Lanschot JJ. Monitoring of response to pre-operative chemoradiation in combination with hyperthermia in oesophageal cancer by FDG-PET. Int J Hyperthermia 2006; 22: 149–160
- Mueller-Klieser W, Walenta S, Paschen W, Kallinowski F, Vaupel P. Metabolic imaging in microregions of tumors and normal tissues with bioluminescence and photon counting. J Natl Cancer Inst 1988; 80: 842–848
- Horsman MR, Overgaard J. Hyperthermia: A potent enhancer of radiotherapy. Clin Oncol 2007; 19: 418–426