Abstract
Purpose: To investigate the impact of intravenously injected gold nanoparticles on interstitially delivered laser induced thermal therapy (LITT) in the liver.
Methods: 3D finite element modelling, ex vivo canine liver tissue containing gold nanoparticles absorbing at 800 nm, and agar gel phantoms were used to simulate the presence of nanoparticles in the liver during LITT. Real-time magnetic resonance temperature imaging (MRTI) based on the temperature sensitivity of the proton resonance frequency shift (PRFS) was used to map the spatiotemporal distribution of heating in the experiments and validate the predictions of 3D finite element simulations of heating.
Results: Experimental results show good agreement with both the simulation and the ex vivo experiments. Average discrepancy between simulation and experiment was shown to be 1.6°C or less with the maximum difference being 3.8°C due to a small offset in laser positioning.
Conclusion: A high nanoshell concentration in the surrounding liver parenchyma, such as that which would be expected from an intravenous injection of gold nanoshells (∼120 nm) acts as both a beam stop for the laser and secondary heat source for the treatment, helping to better heat the lesions and confine the treatment to the lesion. This indicates a potential to use nanoparticles to enhance both the safety and efficacy of LITT procedures in the liver.
Introduction
The majority of cancer lesions in the liver occur as a result of metastases from organs elsewhere in the body Citation[1]. Current treatment options include surgical resection, chemotherapy and radiofrequency Citation[2]. Surgical resection is possible in only a small number of patients Citation[2], chemotherapy is not specific to the lesion but will affect other organs. Radiotherapy ablation can lead to complications such as hepatic failure, intraperitoneal bleeding, hepatic abscess, and bile duct injury Citation[3].
In recent years laser thermal therapy has also been employed to treat localised liver disease and showed less than 5% recurrence in 6 months regardless of tumour size Citation[4]. Minimally invasive MR-guided laser-induced thermal therapy (MRgLITT) of liver metastases has already transitioned into the clinical setting Citation[4–7]. MRgLITT can be coupled with the use of gold coated nanoshells (AuNS). These exhibit a plasmon resonance when excited by laser radiation, the optical frequency of this resonance is determined by the thickness of the gold shell that surrounds the exterior of the silica core Citation[8], Citation[9]. The property of nanoshells to absorb strongly at a specific wavelength and cause intense local heating will amplify the ultimate effect of laser thermal therapy in liver tumours. Biodistribution studies in mice Citation[10] have shown 13.6% of the nanoshells will be taken up by the liver due to the reticuloendothelial system 24 h after an intravenous infusion of nanoshells. The majority of the nanoshells (83%) were taken up by the spleen with trace amounts in other organs. A large concentration of nanoshells within the liver will alter the geometry as well as the magnitude of the laser heating Citation[11] and influence the outcome of the treatment.
In this work we simulate the uptake of intravenously injected nanoshells in liver with a tumour to investigate the impact on interstitial laser-induced thermal therapy, based on results of SPIO uptake Citation[12] it is assumed that in clinical cases very few nanoshells would transfer from the liver to the tumour. The hypothesis is that for an applicator placed in the tumour, photons spreading out from the fibre source inside the tumour will strike the nanoshells at the interface between the tumour and the healthy liver tissue, providing a secondary source of heating at the periphery, helping to distribute the energy of the beam through the tumour and increase the rate and size of the ablation zone. Phantom and ex vivo laser experiments were conducted using a canine liver with a tumour-like inclusion; with real-time temperature monitoring, using magnetic resonance temperature imaging (MRTI) Citation[13], Citation[14]. Finite element modelling simulated the therapy for comparison with the phantom experiments. Experimental results show good agreement with both the simulation and the ex vivo experiments validating the hypothesis that a high nanoshell concentration in the surrounding liver acts as a beam stop for the laser and secondary heat source for the treatment.
Materials and methods
All phantom and ex vivo experiments were carried out in a 1.5 T General Electric MRI scanner (Signa Excite, HD, Milwaukee, WI) using a 2D fast, radio frequency-spoiled, gradient-echo sequence (TR/TE = 38/10 ms, flip angle = 30°, FOV = 140 mm, matrix = 256 × 128, slice thickness = 4 mm, receiver bandwidth = 14.2 kHz, acquisition time = 5 s/image). The laser source was a clinical Diomed 15 plus EVLT (Sigmacon Medical Products, Ontario, Canada) continuous wave (CW) diode laser capable of 15 W output in the 790–830 nm range. The nanoshells themselves were obtained from a commercial vendor (Nanospectra Biosciences, Houston, TX); they consisted of a 110 nm spherical silica core with an approximately 10 nm thick gold coating giving the nanoshell maximum absorption at ∼810 nm.
Phantom experiments
The phantom consisted of a simulated tumour inclusion surrounded by simulated liver parenchyma with and without nanoshells. The tumour phantom was formed in a 15 mL conical tube of 2.95 mL of evaporated milk +21 µL of ICG and filled to 15 mL with 0.5% agarose. Phantom properties were based on the measured optical properties of liver and tumour and are similar to those used by other authors Citation[15]. The liver phantom was formed in a 52 × 52 × 56 mm plastic cube of 29 mL of evaporated milk +2.64 mL of ICG, 6.46 mL of stock nanoshells, and filled to 400 mL with 0.5% agarose. Before the addition of nanoshells, the phantom has an optical penetration depth of 0.6 cm at 808 nm. With the addition of the nanoshells to the liver phantom the scattering coefficient was 57.4 cm−1 and the absorption coefficient 2.89 cm−1, the optical penetration depth at 808 nm decreased (see ). These phantoms were prepared by Nanospectra Biosciences, the values of scattering and absorption were also supplied by Nanospectra Biosciences. The 2-cm diameter ‘tumour phantom’ was positioned inside the liver phantom and the 2-mm diameter catheter containing the laser fibre was then inserted down the centre of the tumour phantom as shown in . Initially measurements were conducted at a laser power of 15 W for 3 min to ensure that the gel did not melt. This was shown not to occur and later measurements were performed at 15 W for 5 min.
Figure 1. Schematic showing the laser position inside the phantom. The tumour is seen as the central region surrounding the laser. The grey area at the end of the laser shows the diffusive tip, the active, light-emitting region of the laser.
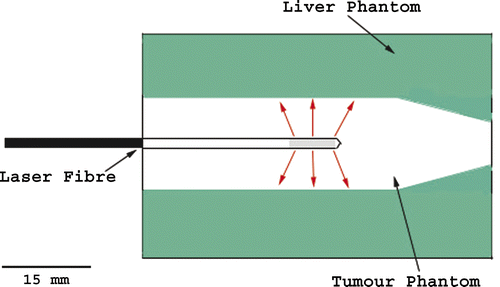
Table I. Optical properties of phantoms.
Simulation using ex vivo canine liver
For ex vivo experiments, the optically equivalent tumour phantom from the previous experiment was placed inside a freshly excised canine liver of an animal that had been injected with nanoshells 24 h previously Citation[16] for treatment of an implanted tumour in a separate location. The liver was prepared by cutting a recess large enough to contain the cylindrical tumour occlusion; the tumour phantom then had the interstitial laser fibre inserted into it. A biodistribution study to determine uptake of nanoshells in several organs was performed Citation[16]. The nanoshell uptake per gram of tissue in the liver was found to be 400 ± 128 µg/g. It was determined that the amount of gold per nanoshell was 1.66 × 10−14 g/NS which gives the nanoshell concentration per gram of tissue in the liver as 2.4 × 1010± 7.7 × 109 NS/g.
Numerical simulation of nanoshell enhanced laser heating
In order to characterise the thermal response of nanoshells and to predict whether our hypothesis was true for planning experiments, we implemented a finite element model simulation of the spatiotemporal heating of the nanoshells and verified the model in phantom material via MR temperature imaging. Temperature distribution throughout the phantom was modelled using the non-homogeneous heat equation Citation[17] given bywhere T = temperature in Kelvin, ρ = density of phantom material (kg m−3), c = specific heat of phantom material (J kg−1°C−1), k = thermal conductivity of tissue (W m−1°C−1), qi = Cartesian coordinates (m), t = time (s), P = power deposited per unit volume by the laser (W m−3). The term P was calculated using optical diffusion approximation which has been used by several authors Citation[18], Citation[19] to describe laser penetration into tissue and was used to describe the tumour tissue that was assumed to contain no nanoshells. The model was implemented using COMSOL Multiphysics (Heat Transfer Module, Comsol, Burlington, MA). Equation 1 was solved numerically using the COMSOL software, values of specific heat, thermal conductivity and density as well as the absorption and reduced scattering coefficients (μa and μ’s) for the parts of the phantom containing no nanoshells were taken from published sources Citation[20], Citation[21]. The mesh generated for the finite element calculations was a 2D triangular mesh with an average triangle size of 0.02 cm2. The density of this mesh was increased by a factor of 2 in the regions of greatest temperature increase, this increased computational accuracy without a large increase in computation time.
Results
Phantom experiments
All temperatures quoted in the following results are relative temperatures and reflect the increase in temperature above background.
These experiments were carried out using a laser power of 15 W for 5 min as opposed to the 15 W for 3 min for the ex vivo experiments due to the fragile nature of the 0.5% agar-milk phantoms being used. shows the results of the liver phantom experiment using the interstitial fibre. shows the actual temperature overlay of the thermal distribution onto an MRI image of the phantom. The solid rectangle in the upper part of the image indicates the size of the diffusive section of the laser fibre and the white line indicates the margin of the tumour component of the phantom. The diameter of the tumour section within the phantom was 2 cm. The maximum temperature change reached during the experiments was 24°C above background and is indicated in which shows the temperature profile taken at the time the maximum temperature was reached. The black line in the centre indicates the width of the catheter carrying the cooling water surrounding the laser fibre which showed no heating. The grey region indicates the tumour and the white region represents the normal liver tissue containing the nanoshells. shows the same experimental setup as ; however, this phantom has no nanoshells in the liver portion of the phantom. In this case the maximum temperature rise was only 6°C.
Figure 2. Phantom demonstrating the effect of nanoshells in the surrounding parenchyma as opposed to in the tumour. The MRTI measured thermal distribution generated by the phantom with nanoshells outside the white line (A) at the point where the maximum temperature was reached and the resulting spatial profile where the nanoshells lie outside the grey region and the laser fibre is shown by the black region (B). The maximum change in temperature was 24°C versus performing the experiment in a phantom without nanoshells (C) where the maximum change in temperature was only 6°C.
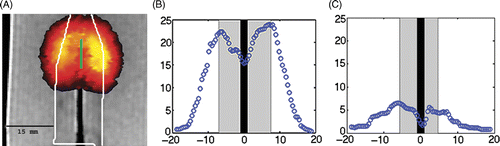
Simulation using ex vivo canine liver
The diameter of the tumour phantom used in the canine livers was 0.8 cm (the diameter was set by the container it was made in). shows results of heating ex vivo canine liver containing tumour phantom for time points 20 s (prior to heating), 75 s (point of maximum temperature when laser was switched off) and 150 s into the heating, indicating that the diffusive component of the heating does not spread appreciably once it is established. The plot of the heating profiles for 75 s and 150 s is shown in . The green dot at the centre indicates the laser position, the cyan coloured outline surrounds the tumour phantom, and the black outline indicates a fluid pocket that formed between the tumour phantom and the liver prior to the experiment. At 75 s into treatment a maximum temperature change of approximately 38°C was obtained in this imaging plane. This maximum temperature occurred at the border between the tumour phantom and the liver, in good agreement with the original hypothesis. shows temperature profiles for the heating at 75 s and 150 s, the grey area indicates the width of the occlusion. The difference in temperature across the occlusion at 75 s is 12°C; such a large temperature difference across a tumour could adversely affect the therapeutic value of the treatment by leaving the tumour partly unablated. This is partly due to the presence of the fluid pocket and partly due to the closer proximity of the fibre to the left side of the tumour as indicated by the dashed lines in . also shows explicitly the width of the heating not spreading to any appreciable extent (average = 1.3 mm) into the surrounding healthy tissue for both cases. This thermal containment takes place in spite of the fact that there are effectively two heating sources, the laser itself and the nanoshells at the edge of the tumour.
Figure 3. Axial view of the experiment performed using a nanoshell-laden canine liver ex vivo. On a T2-weighted MRI, the cyan line indicates the boundary between tumour phantom and liver parenchyma (dark on image), a bright water pocket (black line) and the laser fibre (green) (A). The maximum extent of heating measure by MRTI is shown on the MRI (B) the maximum temperature was 40°C above background. Diffusion of heat into surrounding healthy tissue is shown in (C) and (D) shows how the heat dissipates within the occlusion.
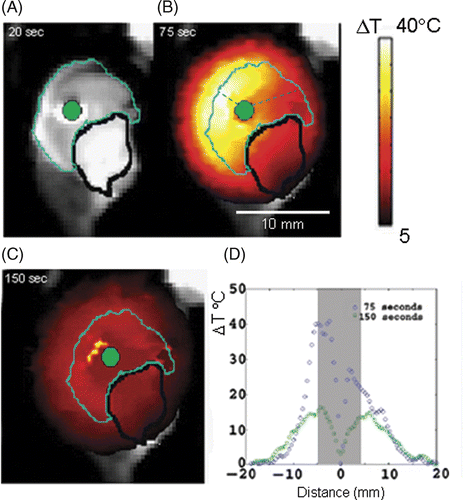
shows the sagittal view of the ex vivo tumour heating experiment. In the tumour phantom is the dark area outlined in white with the green solid line representing the laser probe. shows the thermal distribution at the time of maximum heating. The maximum temperature reached is seen to arc around the laser probe following the white line that is the boundary between the tumour phantom and the liver tissue; this is in accordance with the original hypothesis. The laser fibre in this case penetrated beyond the end of the tumour phantom into the nanoshell-containing healthy liver tissue and a small fluid pocket was formed at the tip. shows that heating takes place in an arc around the periphery of the fluid pocket at the interface of the nanoshell/non-nanoshell region in the same way it does in the tumour, showing the high density of nanoshells in the liver tissue acts as a ‘beam stop’ for the laser photons and a secondary heating source for thermal therapy. show temperature profiles taken vertically and horizontally through the tumour, the grey region represents the width of the tumour where these profiles were taken. was taken vertically through the thermal distribution as shown by the dashed line in and shows similar behaviour to previous examples, the maximum temperature change (43° ± 3°C in this plane) is obtained at the edge of the tumour with drop-off down to non-ablative temperatures (∼10°C above background) taking place within 10 mm of the lesion. shows the heating profile taken along the line of the laser fibre; near the surface and away from the sides of the occlusion the temperature is relatively low, increasing by only about 20°C above the background, moving along the fibre closer to the nanoshell laden liver the temperature rises rapidly to 43° ± 3°C above background.
Figure 4. Sagittal view of the ex vivo tumour. The dark area outlined in white in (A) is the tumour phantom, the green solid line represents the laser probe. (B) The highest temperature increase was 45°C in this plane above background and is seen at the boundary of tumour and healthy tissue. The end of the laser probe passes through the tumour into the healthy tissue beyond. (C) shows temperature distribution through the dashed line in (B). (D) shows temperature distribution along the laser fibre.
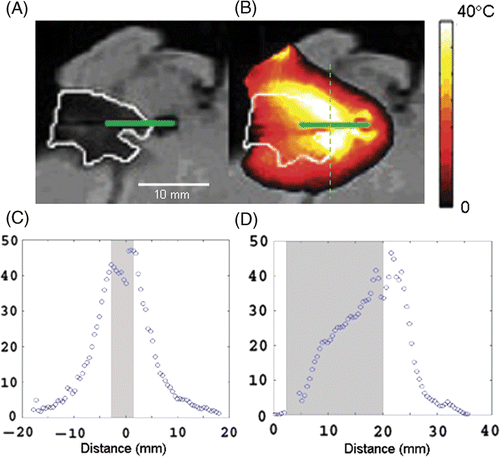
Numerical simulation of nanoshell enhanced laser heating
shows the predicted temperature profile following 3 min heating; the temperature peak was seen to coincide with that of the tumour. The maximum temperature change obtained was 23.5°C at the boundary of the tumour and the lowest temperature rise within the tumour was 15°C. This is consistent with the temperature rises observed in the experimental data of , the root mean square difference between the model and experiment was taken as an estimate of the root mean square (RMS) error and found to be 1.6°C with the maximum temperature difference being 3.8°C. shows the model predicts that maximum heating occurred at the boundary between the healthy liver and the phantom, which again supports the original hypothesis. compares the time history near the edge of the occlusion for the experimental data and the finite element simulation. The heating part of the simulation curve agrees well with experiment, the RMS error between the two is 1°C. Once the point of maximum temperature is reached and cool down begins, the two curves tend to deviate more substantially, possibly because of some damage sustained by the phantom during heating. Another point to note for the heating part of the curve and the chief source of error is that the curvature of the finite element simulation is less than that of the experiment. This appears due mainly to the thermal conductivity of the medium.
Discussion
Results from phantom and ex vivo experiments demonstrate that the large concentration of nanoshells which accumulate in the liver can be utilised to enhance heating by percutaneous LITT procedures for liver tumours. The nanoshells essentially function as a ‘beam stop’ for photons emitted from the laser placed within the tumour. For the 10 W phantom experiment this is demonstrated by the 18°C difference in the temperature between the nanoshell and non-nanoshell phantoms in with the highest temperature change obtained at the interface between nanoshell bearing and non-nanoshell bearing parts of the phantom. This is in agreement with the initial hypothesis. and show a similar effect in the 15 W ex vivo treatment, where a temperature change of 38°C is observed at the boundary between tumour phantom and liver tissue. This high temperature is seen on one side of the tumour due to the closer proximity of the laser and the liver border as the laser probe did not go through the centre of the tumour phantom. This observation emphasises the need for multiple fibre treatments in larger tumours to maximise treatment efficacy by allowing therapeutic ablative temperatures to be obtained throughout the tumour volume. The presence of a water pocket near the bottom of the occlusion in prevented heating in that region of the occlusion. The therapeutic effect of the laser heating is confined to the volume of the tumour with some heating in the periphery of the healthy liver tissue. Both experiment and simulation show that outside the tumour boundary the temperature falls off by 50% within approximately 5 mm. The heating extends slightly beyond the tumour margin which will potentially tackle micrometastasis seen at the tumour border.
Simulation of the phantom data was shown to agree reasonably well with experiment. Qualitatively, the simulation was able to reproduce the principal features of the experimental thermal distribution including the high temperature maxima at the interface of the nanoshell/non-nanoshell region as well as the local minima in the centre of the profile associated with the laser fibre cooling water. Qualitatively, the agreement between experiment and simulation was found to be reasonable with the root mean square difference between the two being 1.6°C overall. Maximum difference of 3.8°C was noted because the geometry of the simulation was perfectly concentric and did not account for the off-center laser fiber placement, which lead to a higher temperature on one side of the phantom. The results of time versus temperature show reasonable agreement with experiment but also demonstrate the need for future work to improve the laser model. One of the most problematic issues is the accuracy of quoted values for thermal conductivity and specific heat.
In the current model these values are constant and do not change as functions of temperature; this will be the subject of future work. By allowing these values to change during heating we would be better able to predict the final outcome in terms of the damage to the tumour.
Declaration of interest: The authors report no conflicts of interest. The authors alone are responsible for the content and writing of the article.
References
- Kanematsu M, Kondo H, Goshima S, Kato H, Tsuge U, Hirose Y, Kim MJ, Moriyama N. Imaging liver metastases: Review and update. Eur J Radiology 2006; 58: 217–228
- McKay A, Dixon E, Taylor M. Current role of radiofrequency ablation for the treatment of colorectal liver metastases. Br J Surgery 2006; 93: 1192–1201
- Kong WT, Zhang WW, Qiu YD, Zhou T, Qiu JL, Zhang W, Ding YT. Major complications after radiofrequency ablation for liver tumors: Analysis of 255 patients. World J Gastroenterol 2009; 15(21)2651–2656
- Vogl TJ, Mack M, Eichler K, Lehnert T, Nabil M. Effect of laser-induced thermotherapy on liver metastases. Expert Rev Anticancer Therapy 2006; 6(5)769–774
- Germer CT, Albrecht D, Roggan A, Isbert C, Buhr HJ. Experimental study of laparoscopic laser-induced thermotherapy for liver tumours. Br J Surgery 1997; 84: 317–320
- Stroszczynski C, Gretschel S, Gaffke G, Puls R, Kretzschmar A, Hosten N, Schlag PM, Felix R. Laser-induced thermotherapy (LITT) for malignant liver tumours: The role of sonography in catheter placement and observation of the therapeutic procedure. Ultraschall Med 2002; 23(3)163–167
- Mack MG, Eichler K, Zangos S, Lehnert T, Vogl TJ. Long-term results of MR-guided laser ablation (LITT) of liver metastases of breast cancer. J Clin Oncol 2008; 26(15S)1037
- Hirsch LR, Stafford RJ, Bankson JA, Sershen SR, Rivera B, Price RE, Hazle JD, Halas NJ, West JL. Nanoshell-mediated near-infrared thermal therapy of tumors under magnetic resonance guidance. PNAS 2003; 100(23)13549–13554
- Bohren CF, Huffman DR. Absorption and scattering of light by small particles. Wiley-VCH, New York, NY 2004
- James WD, Hirsch LR, West JL, O'Neal PD, Payne JD. Application of INAA to the build-up and clearance of gold nanoshells in clinical studies in mice. J Radioanalytical Nuclear Chemistry 2007; 271(2)455–459
- Maksimova IL, Akchurin GG, Khlebtsov BN, Terentyuk GS, Akchurin GG, Ermolaev IA, Skaptsov AA, Soboleva EP, Khlebtsov NG, Tuchin VV. Near-infrared laser photothermal therapy of cancer by using gold nanoparticles: Computer simulations and experiment. Med Laser Applic 2007; 22: 199–206
- Ma HL, Xu YF, Qi XR, Maitani Y, Nagai T. Superparamagnetic iron oxide nanoparticles stabilized by alginate: Pharmacokinetics, tissue distribution, and applications in detecting liver cancers. Int J Pharmaceutics 2008; 354: 217–226
- de Senneville BD, Quesson B, Moonen CTW. Magnetic resonance temperature imaging. Int J Hyperthermia 2005; 21: 515–531
- Elliott AM, Stafford RJ, Schwartz J, Wang J, Shetty AM, Bourgoyne C, O'Neal P, Hazle JD. Laser-induced thermal response and characterization of nanoparticles for cancer treatment using magnetic resonance thermal imaging. Med Physics 2007; 34(7)3102–3108
- Germer CT, Roggan A, Ritz JP, Isbert C, Albrecht D, Müller G, Buhr HJ. Optical properties of native and coagulated human liver tissue and liver metastases in the near infrared range. Lasers Surg Med 1998; 23(4)194–203
- Schwartz JA, Shetty AM, Price RE, Stafford RJ, Wang JC, Uthamanthil RK, Pham K, McNichols RJ, Coleman CL, Payne JD. Feasibility study of particle-assisted laser ablation of brain tumors in orthotopic canine model. Cancer Res 2009; 69(4)1659–1667
- Olsrud J, Wirestam R, Persson BRR, Tranberg KG. Simplified treatment planning for interstitial laser thermotherapy by disregarding light transport: A numerical study. Lasers Surg Med 1999; 25: 304–314
- Cai W, Xu M, Alfano RR. Three-dimensional radiative transfer tomography for turbid media. IEEE J Selected Topics Quantum Electronics 2003; 9: 189–198
- Welch AJ. The thermal response of laser irradiated tissue. IEEE J Quantum Electronics 1984; 20: 1471–1481
- Duck FA. Physical Properties of Tissue. A Comprehensive Reference Book. Academic Press, San Diego CA 1990; 50
- Fantini S, Walker SA, Franceschini MA, Kaschke M, Schlag PM, Moesta KT. Assessment of the size, position, and optical properties of breast tumors in vivo by noninvasive optical methods. Applied Optics 1998; 37(10)1982–1989