Abstract
Purpose: We report on the design, performance, and specifications of a dedicated set‐up for the treatment of rats on a clinical magnetic resonance high intensity focused ultrasound (MR‐HIFU) system.
Materials and methods: The small animal HIFU‐compatible 4‐channel MR receiver volume coil and animal support were designed as add‐on to a clinical 3T Philips Sonalleve MR‐HIFU system. Prolonged hyperthermia (T ≈ 42°C, 15 min) and thermal ablation (T = 65°C) was performed in vivo on subcutaneous rat tumours using 1.44 MHz acoustic frequency. The direct treatment effect was assessed with T2‐weighted imaging and dynamic contrast enhanced (DCE‐) MRI as well as histology.
Results: The developed HIFU‐compatible coil provided an image quality that was comparable to conventional small animal volume coils (i.e. without acoustic window), and a SNR increase by a factor of 10 as compared to the coil set‐up used for clinical MR‐HIFU therapy. The use of an animal support minimised far field heating and allowed precise regulation of the animal body core temperature, which varied <1°C during treatment.
Conclusions: The results demonstrated that, by using a designated set‐up, both controlled hyperthermia and thermal ablation treatment of malignant tumours in rodents can be performed on a clinical MR‐HIFU system. This approach provides all the advantages of clinical MR‐HIFU, such as volumetric heating, temperature feedback control and a clinical software interface for use in rodent treatment. The use of a clinical system moreover facilitates a rapid translation of the developed protocols into the clinic.
Introduction
Magnetic resonance guided high intensity focused ultrasound (MR‐HIFU) is a promising technique for controlled non‐invasive tumour treatment by selective tissue heating Citation[1], Citation[2]. By focusing the mechanical ultrasound waves, ultrasonic energy can be deposited deep within the body without harming surrounding tissue, which is not possible with other thermal therapy methods (e.g. laser, radiofrequency, microwave or cryothermal). Clinical application of HIFU has been accelerated with the introduction of image guidance, especially MR imaging Citation[3]. The latter offers excellent soft tissue contrast and spatial resolution that aids in planning the HIFU treatment and also evaluating the treatment outcome Citation[4]. More importantly, MR has the ability to map the local temperature distribution within the targeted location by dynamic acquisition of MR phase images Citation[5], Citation[6], which can serve as feedback to the ultrasound control unit. Using MR‐controlled ultrasonic heating, the local temperature can be slightly increased and maintained at a level of hyperthermia (T = 41°–47°C), or the temperature can be raised further, leading to cell death (thermal ablation) Citation[7–9]. The thermal dose at which cell death occurs varies for different types of tissue Citation[10–12].
Tumour thermal ablation with MR‐HIFU is currently being introduced to the clinic to treat both benign (e.g. uterine fibroids Citation[13], Citation[14]) and malignant tumours (e.g. breast Citation[15], Citation[16]). In contrast to benign tumours, the clinical treatment of malignant tumours demands complete coverage. There, treatment with high spatial and thermal precision is more of a critical issue as malignant tumour border zones need precise treatment to prevent local recurrence and to minimise damage to surrounding healthy tissues Citation[17]. To achieve complete coverage of large tumours, volumetric ultrasonic heating combined with multiplane MR‐based temperature mapping allows for depositing a well‐defined thermal dose within a predefined treatment volume Citation[18]. Hyperthermia‐induced drug delivery is another promising application that can benefit from the temperature feedback made possible by the MR‐HIFU technology. For this application, a tumour needs to be heated to a constant temperature of around T = 42°C for a prolonged time period Citation[19], Citation[20].
The clinical translation of malignant tumour ablation and hyperthermia‐induced drug delivery using MR‐HIFU requires thorough pre‐clinical testing in large cohorts together with therapy monitoring methods. For this, studies performed with small animal models such as mice and rats are preferred over larger animals (e.g. rabbits, pigs) for both ethical and practical reasons, as well as the extensive knowledge and availability of antibodies and tumour models in rodents, for example xenografts in immune compromised strains. Rabbit tumour therapy studies are limited by the restricted choice in well characterised transplantable tumour models (currently VX2 only Citation[21], Citation[22]). Furthermore, the natural body temperature of rabbits is slightly higher than the human body temperature (39°C as compared to 37°C), which, although used Citation[23], Citation[24], does not make them ideal candidates for use in mild hyperthermia studies. Finally, therapeutic outcome can be evaluated more easily in small animals using standard small animal imaging equipment for positron emission tomography (PET) or single photon emission computed tomography (SPECT) studies as compared to similar evaluations in larger animals.
Most focused ultrasound studies in rodents are performed using manual positioning systems, which limits the type and amount of exposures Citation[25], Citation[26], and/or are performed under ultrasound guidance Citation[27], Citation[28], which lacks an accurate temperature feedback. Chopra et al. Citation[29] were the first to present a MR‐compatible prototype system for ultrasound treatments in rodents under MR guidance with a piezoelectric 3‐axis positioning system. Meanwhile, more systems have been developed including phased array systems in high field small bore animal scanners Citation[30].
In contrast to studies in rodents, MR‐HIFU studies in pigs and rabbits have the advantage that a clinical system can be used for therapy. Most clinical MR‐HIFU systems provide automated transducer positioning with a phased‐array transducer and MR‐temperature feedback combined with a software control interface enabling accurate planning and heating of the entire target area to a predefined temperature without re‐positioning the animal. Furthermore, the thermal dose delivered to the target tissue is calculated online and can, in addition to the measured temperature change, be used as feedback to guide therapy. The use of a phased array transducer allows complete electronic control over the ultrasound field, which could for example be exploited for fast volumetric heating of the target tissue Citation[22], or for compensation of motion or beam distortion. Finally, the use of a clinical system during pre‐clinical studies facilitates a rapid translation of the developed protocols to clinic.
MR‐HIFU treatment and treatment monitoring of large animals or humans differs from treatment and treatment monitoring in rodents due to the target size. First of all, a high spatial resolution (approximately 1 mm) is required for accurate temperature feedback in rat‐sized tumours (typically around 10 × 10 × 10 mm3). Increasing the spatial resolution of the temperature feedback on the clinical MR‐HIFU system would immediately affect the signal‐to‐noise ratio, which in turn would increase the measured temperature uncertainty Citation[31]. Furthermore, high resolution anatomical images are required for MR therapy assessment post small animal treatment. The use of conventional (phased array) small animal MR volume coils would offer the required image quality; however, most of these coils do not have an acoustic window and are therefore not HIFU compatible.
Another difficulty in treating small animals is to control the exit of the ultrasound wave. The acoustic beam still contains a large part of its initial energy after the target due to the small pathway travelled through tissue. Reflection of this energy would result in uncontrolled far field heating. Finally, the focal point dimensions in combination with the heat diffusion and limited heat capacity of small animals makes it a challenge to treat a small part of the animal while doing minimal harm to healthy tissues and keeping the animal body temperature constant.
We recently showed the use of a dedicated set‐up for hyperthermia‐induced drug delivery treatment of rats on a clinical MR‐HIFU system Citation[32]. Here we report on the design, specification, and performance of this set‐up and show its use for both hyperthermia and ablation treatment of rat tumours. We show that the different features of the clinical system can be used for treatment of rats and potentially mice. The developed set‐up consisted of a small animal HIFU‐compatible MR receiver coil and animal support. The use of a dedicated coil resulted in a higher signal‐to‐noise ratio and/or increased spatial resolution for accurate temperature mapping and therapy monitoring in small animals, while the animal support set‐up minimised far field heating and allowed precise regulation of the animal body temperature. The performance of the combined system was tested in a proof‐of‐concept study, showing that volumetric tumour ablation as well as maintained tumour hyperthermia using a binary feedback temperature control algorithm was possible in rats. The short‐term treatment effect was monitored with standard dynamic contrast enhanced (DCE) MRI in combination with T2‐weighted anatomical imaging and histology.
Methods
MR‐HIFU
Pre‐clinical HIFU treatments were performed using a clinical MR‐HIFU platform (Philips Sonalleve®, Vantaa, Finland) integrated into a 3T human MR scanner (Philips Achieva®, Best, the Netherlands). The HIFU platform included a HIFU transducer embedded in a MR table top, a RF‐generator cabinet and a workstation for therapy control. The 256‐element spherical shell (12 cm radius of curvature, 13 cm aperture) phased‐array HIFU transducer (Imasonic SA, Besançon, France) could be mechanically translated with five degrees of freedom (three translational and two rotational). The focal point (1 × 1 × 9 mm3 at −6 dB, measured using a needle hydrophone in water at 1.44 MHz) could be steered electronically allowing volumetric heating of the target area. The clinical MR‐HIFU platform was equipped with a single loop RF coil element integrated inside the table top surrounding the acoustic window, combined with a multi‐element coil that could be placed on top of the pelvic area of the patient.
Small animal set‐up
In order to comply with the demands of small animal treatment monitoring (high spatial and temporal resolution), a dedicated small animal HIFU compatible MR receiver coil (Philips Healthcare) was designed and developed. The coil was a 4‐channel volume coil designed for full body, high quality imaging of rats or mice (maximum weight 400 g). The multichannel volumetric design allowed for parallel imaging (sensitivity encoding, SENSE Citation[33]) which could be utilised to decrease the image acquisition time. Mutual coupling of the individual coil elements was prevented by capacitive decoupling of the bottom two elements, geometrical overlap between laterally adjacent upper and lower elements, and high impedance preamplifiers for the upper two elements. High‐pass filtering at the MR receive coil prevented possible interference of the low frequency electrical signals from the ultrasound driver chain with the MR radio frequency signal.
To create an acoustic pathway for the ultrasound beam from the transducer to the inner part of the small animal coil, the coil had an open structure () and was partially immersed in demineralised degassed water. To this end, the coil was made fully waterproof and was carefully tuned to compensate the effects of the dielectric properties of the water. A pool that fitted onto the acoustic window in the MR‐HIFU table top was designed to support and fixate the coil (). The bottom of the pool was made of thin transparent polyester film (Mylar®), which was chosen based on thickness and acoustical properties to minimise reflections and refractions of the ultrasound when entering the pool. The space between the MR‐HIFU table top and the pool was filled with a thin layer of degassed water to ensure acoustic coupling between the pool and the acoustic window of the table top. A water circulation system was installed against the sides of the pool to heat the pool water. This set‐up resulted in an accessible sonication volume of approximately 50 cm3. A thin copper strip, placed in the middle of the acoustic window at the bottom of the coil, was physically shared by the bottom two elements of the coil in order to further improve SNR. The strip was designed to minimise its impact on the ultrasound beam and did not noticeably influence the focal spot shape Citation[34].
Figure 1. (A) The acoustic window in the bottom of the small animal HIFU‐compatible MR coil (dimensions 10 × 10 cm2) with the copper strip in the middle. (B) The coil fixed inside the water pool and installed on the patient bed of the MR scanner. (C) Side view of the animal bed design. The space between the foil layers (indicated by the arrow) was filled with degassed water and ultrasound gel. (D) Top view of the animal bed showing the details of the design.
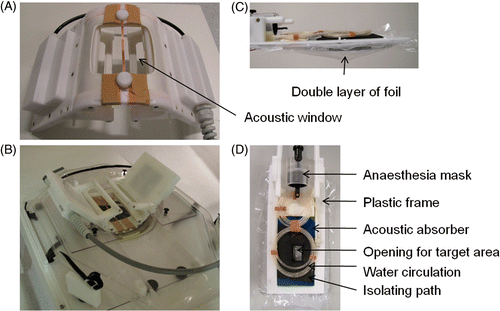
To achieve good coupling of the ultrasound beam into the animal and to minimise far field heating, the target area was submerged in a mixture of degassed water and ultrasound gel. Therefore a cradle was designed that consisted of two layers of thin plastic foil (Saran wrap, Dow, Terneuzen, the Netherlands) stretched in a rectangular plastic frame (). The volume between the two layers of foil was filled with a mixture of degassed water and ultrasound gel (Aquasonic 100, Parker, Fairfield, NJ). The ultrasound gel was added to minimise water convection caused by local heating. An acoustic absorber with a pyramidal structured surface (Aptflex F28P, Precision Acoustics, Dorchester, Dorset) was placed on the top foil layer to eliminate far field heating. The pyramidal structured surface of the absorber ensured that the reflected ultrasound did not converge to a focus. A small part of the absorber was covered with a thin isolating layer of rubber material to protect the animal skin from damage due to direct absorber heating. At the top end of the frame, a rat anaesthesia inhalation mask (Minerve, Esternay, France) was placed. A water circulation system was installed on top of the absorber for temperature regulation of the animal (). Finally, the rat was positioned on top of the circulation tubing with the target area submerged below the rest of the body through an opening in the absorber and top plastic layer ().
Animal protocol
Hyperthermia or ablation treatment was performed on tumour‐bearing female Fisher344 rats (n = 10, age 5–7 weeks). Tumours were inoculated by subcutaneous injection of 5·106 GS 9L cells (rat glioma, HPA Cultures, Salisbury, Wiltshire) on the hind leg. The tumours had a minimum volume of 400 mm3 at the time of treatment. General anaesthesia was induced and maintained by inhalation of isoflurane (1–3%) in medical air (flow 0.4–0.6 L/min). All animals received RIMADYL® (carprofen, 3 mg/kg bw) as precautionary pain suppressor prior to HIFU treatment. The lower part of the animal was shaved using depilatory cream (Veet, Hoofddorp, the Netherlands). The skin on the target area was covered with degassed ultrasound (US) gel (Aquasonic 100) after which the animal was positioned inside the small animal MR‐HIFU set‐up. Vital parameters were monitored throughout the entire treatment, including respiration frequency using a small balloon sensor (Graseby, Smiths Medical, St Paul, MN) and body temperature using a rectal temperature probe (Neoptix, Québec City, Canada). At the end of the experiment, animals were euthanised by tissue perfusion fixation and the treated area was dissected and conserved for histology. All animal experiments were approved by the animal welfare committee of Maastricht University (the Netherlands) and were in compliance with the guidelines set by the institutional animal care committee, accredited by the National Department of Health.
MR acquisition
The HIFU therapy was planned on a T2‐weighted 3D image set acquired with a turbo spin echo scan (TSE‐factor: 12, TR/TE: 1500/40 ms, FOV: 150 × 99 × 100 mm3, voxel size: 0.6 × 0.6 × 1.0 mm3, SENSE‐factor: 1.5 (in both RL and AP direction), number of averages: 2, acquisition time: 9:22 min). Subsequently, a susceptibility sensitive scan (T1W 3D steady‐state gradient‐echo, TR/TE: 15/12 ms, FA: 10°, FOV: 280 × 280 × 40 mm3, voxel size: 1.1 × 1.1 × 2.0 mm3, slices: 20, acquisition time: 3:56 min) was acquired to check for presence of air bubbles in the ultrasound beam path.
Direct treatment effects were monitored by comparing T2‐weighted and dynamic contrast enhanced T1‐weighted MR images acquired before and after HIFU treatment. The T2‐weighted images were acquired with a TSE scan at the tumour location (TSE‐factor: 7, TR/TE: 7644/50 ms, FOV: 40 × 60 × 50 mm3, voxel size: 0.5 × 0.5 × 0.6 mm3, slices: 20, rest slabs: 2, acquisition time: 1.39 min (respiratory triggered)) before treatment, immediately after treatment (within 15 min) and 1 h post treatment. The T2‐weighted images acquired before treatment were used to determine tumour size in a slice by slice approach. DCE‐MRI was performed 1 h before and 30 min after treatment using the low molecular weight contrast agent gadopentetate dimeglumine (Gd‐DTPA, Magnevist®, Bayer Schering, Berlin, Germany). A pre‐contrast reference scan was performed (single shot 3D gradient‐echo, TR/TE: 4.1/1.74 ms, FA: 3°, FOV: 80 × 80 × 42 mm3, voxel size: 0.7 × 0.7 × 0.7 mm3, number of averages: 4, acquisition time: 28.4 s) to calculate a pre‐contrast T1 map required for quantitative analysis of the uptake kinetics Citation[35]. Gd‐DTPA was injected via a tail vein catheter using an infusion pump (0.2 mmol/kg bw, 0.2 mL/min) after the tenth dynamic scan during a dynamic scan series (single shot 3D gradient‐echo, TR/TE: 4.1/1.74 ms, FA: 13°, FOV: 80 × 80 × 42 mm3, voxel size: 0.7 × 0.7 × 0.7 mm3, number of averages: 1, dynamic scan time: 10.7 s, number of dynamics: 90, acquisition time: 16:05 min).
During ablation therapy, the temperature change in the target region was monitored by continuous acquisition of three adjacent slices perpendicular to the beam axis centred on the target area (RF‐spoiled gradient echo with EPI readout, EPI‐factor: 7, TR/TE: 52/20 ms, FOV: 250 × 250 mm2, voxel: 1.4 × 1.4 × 4.1 mm3, fat suppression: spectral pre‐saturation with inversion recovery (SPIR), SENSE‐factor: 1.8, SENSE‐direction: RL, number of averages: 2, dynamic scan time: 5.0 s). During hyperthermia therapy, the temperature change in the target region was continuously monitored in one slice placed perpendicular to the beam axis and centred to the target area, which was acquired with the same acquisition parameters as for the ablation therapy but with the number of averages increased to eight, resulting in a dynamic scan time of 5.9 s.
The temperature maps were calculated online using proton resonance frequency (PRF) shift thermometry Citation[6] and displayed on the therapy control workstation. The temperature images were corrected for baseline drift by subtracting the average apparent temperature change calculated from all voxels in the temperature maps that were located at a sufficient distance from the heating trajectory (3 cm from the trajectory centre) to be considered non‐heated. The SNR of the temperature mapping sequence was calculated by dividing the mean signal intensity of an ROI by the standard deviation of the background noise in the same ROI. The background noise was measured by acquisition of a noise dynamic scan. Thermal dose maps of the target area were calculated online based on the obtained temperature images, according to the Sapareto‐Dewey equation Citation[36], with a unit of equivalent minutes at T = 43°C (EM). During heating, the evolving 30 EM and 240 EM thermal dose contours were shown as overlay on anatomical images of the leg and target area. Thermometry and total thermal dose calculation was continued for 3 min after the end of sonication or until the temperature in the target area had returned to normal body temperature.
Volumetric MR‐HIFU therapy
Volumetric heating of the target tissue was achieved by electronic steering of a single focus point along a circular trajectory (diameter = 4 mm, referred to as the treatment cell) perpendicular to the direction of the HIFU propagation, as described by Köhler et al. Citation[18]. The trajectory was divided into eight points, of which the order of sonication was chosen to maximise the distance between successive points, ensuring even temperature rise over the entire circle. Each location was sonicated for 50 ms before switching (<10 µs) to the next position. Ultrasound was applied as continuous wave ultrasound with a frequency of 1.44 MHz. Low power test sonications (acoustic power: 5–10 W, duration: 20 s) were performed prior to the treatment and used to correct for misregistration between the heated area and planned treatment location. For prolonged hyperthermia treatment, the maximum temperature reached in the target area was used as input for a binary feedback control algorithm which was a modified version of the algorithm described by Enholm et al. Citation[37]. Here, the feedback temperature was compared with pre‐defined threshold temperatures (Tmin = 41°C, Tmax = 43°C) and used to switch the transducer power on or off accordingly. In all the hyperthermia and ablation treatments, tumours were treated once using a single ellipsoidal treatment cell of approximately 4 mm in diameter and 10 mm in length. The treatment cell was positioned in the tumour and heated to an average temperature of T = 42°C for 15 min using 8 W acoustic power (ISATA ≈ 117 W/cm2) or shortly to T = 65°C using 30 W acoustic power (ISATA ≈ 441 W/cm2) for hyperthermia and ablation experiments, respectively.
DCE‐MRI analysis
The uptake kinetics of Gd‐DTPA before and after HIFU treatment were analysed on a voxel‐by‐voxel basis using the 2‐parameter Tofts and Kermode model Citation[38]. The model was fitted to the voxel concentration–time curves measured in the treated area and used for the calculation of quantitative parametric maps of the transfer constant ktrans (min−1), the rate constant kep (min−1) and the volume of extra‐vascular extracellular space (EES) per unit volume of tissue ve (−) using an IDL‐based software tool (IDL 6.3, ITT Visual Information Solutions, Boulder, CO). The transfer constant is a measure for the volume transfer between the blood plasma and the EES. Here, it was assumed that the transfer constant was equal to the blood plasma flow per unit volume of tissue due to high vascular permeability for the low molecular weight contrast agent used. The rate constant was defined as the flux rate constant between EES and blood plasma, or kep = ktrans/ve. The arterial input function was described by a bi‐exponential model function as proposed by McGrath et al. Citation[39]. The corresponding model descriptors were determined in independent experiments in rats. A region of interest (ROI) was manually drawn on each slice containing tumour tissue. The non‐perfused tumour volume was calculated before and after HIFU treatment from the number of voxels for which ktrans < 0.04 min−1. This threshold value was based on empirical findings.
Histology
Tumours and surrounding muscles were collected for histological analysis. The tissue samples were split in half to allow for using both paraffin and cryo‐sections. For the paraffin sections, the tissue was fixated in 4% formaldehyde solution for at least 24 h before embedding in paraffin. The embedded tissue was cut into 5‐µm thick slices and stained with haematoxylin and eosin (H&E) to analyse general morphology. For cryo‐sections, the tissue was snap frozen to T = −40°C using 2‐methylbutane (Acros Organics, Geel, Belgium) and stored at T = −20°C. The frozen tissue was cut into 6‐µm thick slices and air dried briefly at room temperature to allow for nicotinamide adenine dinucleotide (NADH) diaphorase staining to analyse cell viability Citation[40]. The cryo‐sections were incubated at T = 37°C for 1 h in a Gomori‐Tris‐HCl buffer (pH 7.4, 10 mL) containing β‐NAD reduced disodium salt hydrate (N8129, Sigma‐Aldrich, St Louis, MO, 10 mg) and nitro blue tetrazolium (N5514, Sigma‐Aldrich, 10 mg). Specimens were then washed and mounted for analysis.
Statistical analysis
Statistical analysis was performed using the Statgraphics software package (Statgraphics Centurion XVI version 16.0.05, StatPoint Technologies, Warrenton, VA). All data were expressed as mean plus or minus standard deviation. DCE‐MRI results obtained before and after MR‐HIFU treatment were tested for normality and compared using one‐way analysis of variance (ANOVA). In case of equal variances, additional post‐hoc analysis was performed with a paired, two‐tailed t‐test. A p value of less than 0.05 was considered statistically significant.
Results
Small animal set‐up
A dedicated HIFU compatible small animal MR receiver coil (, 1B) was developed and used as an add‐on to a clinical MR‐HIFU system. High resolution anatomical images were obtained and used in therapy planning and assessment (, 3B). For temperature mapping in small animals, a spatial resolution of 1.4 × 1.4 × 4.1 mm3 was obtained while providing a high in vivo SNR of around 258 ± 131 in rat tumour (n = 4) and around 20 ± 14 in rat muscle (n = 2) using the temperature‐sensitive EPI sequence (). These SNRs correspond to theoretical temperature uncertainties ≤0.3°C in the targeted tissue Citation[41], Citation[42]. When the multi‐element coil set‐up provided for human uterine fibroid treatment monitoring was used, SNRs of around 21 ± 11 and 4 ± 2 (n = 2, corresponding to theoretical temperature uncertainties ≤1.7°C) were obtained in tumour and muscle tissue respectively, using the same scan parameters.
Figure 3. (A) Sagittal T2‐weighted full body image acquired to plan the therapy. (B) Detailed transversal T2‐weighted image at tumour location acquired with the sequence used for immediate evaluation of the treatment effects. (C) Magnitude image of the temperature mapping sequence in a scan plane perpendicular to the HIFU beam at the tumour location. T, tumour; L, leg; F, foot.
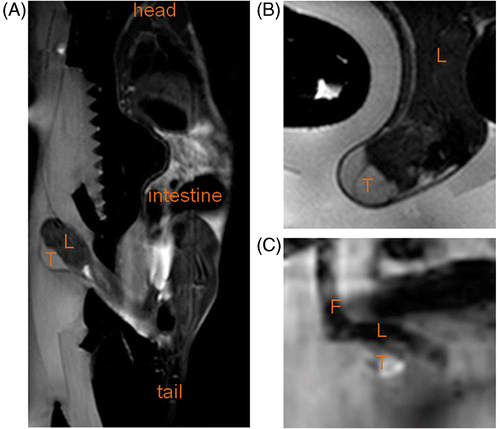
The standard deviation of the temperature change measured with the small animal coil over 15 min in the absence of heating was 0.09°C in a phantom bottle (SNR ≈ 890) and 0.17°C in vivo in tumour tissue (). The small but stable decrease in the in vivo temperature after 400 s () represents a small drift in the animal body temperature, indicating the difficulty of keeping the animal body temperature completely stable. Overall, a very good phase and temperature measurement stability was found over time.
Figure 4. Stability of the temperature change measurements over 15 min, measured in a phantom and in in vivo tumour tissue without heating.
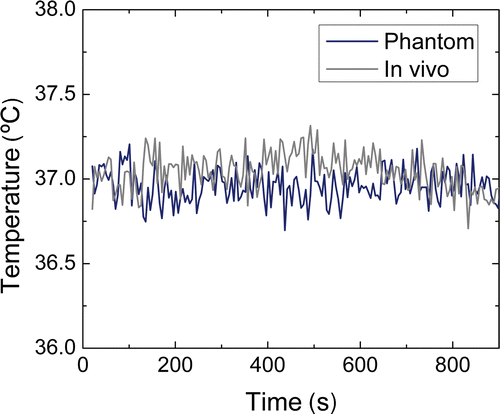
The acoustic absorber placed above the submerged target area limited far‐field heating as well as propagation of the exiting ultrasound beam through the entire animal body. This approach in combination with the water circulation system enabled a steady body temperature of 36.8 ± 0.4°C to be maintained in rats during treatment. No skin or muscle damage was observed at the side of the leg where the ultrasound beam exited the body, indicating controlled exiting of the ultrasound wave. As proof of principle, five rats were treated with hyperthermia and five rats with ablation using the developed set‐up ().
Table I. Overview of the performed animal experiments.
Tumour hyperthermia
In the hyperthermia experiments, the temperature in the treatment volume was successfully elevated. The use of the binary feedback algorithm resulted in a stable cycling temperature development around T = 42°C for 15 min (Tmin ≈ 40.8°C, Tmax ≈ 43.8°C, ). Temperatures slightly above and below the defined thresholds were observed due to the dynamic scan time of the temperature mapping sequence. As the under‐ and overshoot did not exceed 1.8°C, the temporal resolution of 5 s that was obtained in this study was considered feasible.
Figure 5. Temperature distribution during (A) hyperthermia on tumour and (B) ablation on tumour. Plots show respectively an image of the temperature mapping during treatment, in which the colours indicate the temperature distribution and the red contour indicates the tumour area, an anatomical image of the animal leg with tumour with an overlay of the thermal dose contour (acquired at the end of the therapy, orange line indicates 30 EM dose contour, white line indicates 240 EM dose contour; F, feet; M, lower leg muscle; A, planned location of the treatment cell centre). Furthermore, the evolution of the maximum temperature in time and the spatial distribution of the temperature elevation are plotted. The dotted lines indicate the end of the sonication in the temporal temperature profiles and the size of the treatment cell in the spatial temperature profiles. For the tumour ablation, an extra plot is included showing the spatial distribution of the thermal dose are shown. FH, feet–head; LR, left–right.
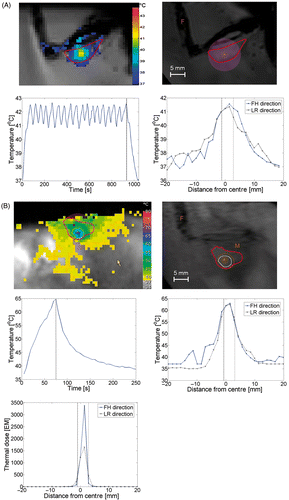
Only minor changes were visible on the T2‐weighted images acquired pre‐ and post‐hyperthermia in treated tumour (average change in signal intensity: −1.4 ± 7.3%, surrounding muscle: 1.0 ± 3.6%) (). A similar result was obtained for T2‐weighted images obtained directly after hyperthermia treatment (average change in signal intensity: 5.3 ± 7.3%, surrounding muscle: 2.3 ± 3.7%) as compared to 1 h post‐HIFU hyperthermia treatment. Kinetic modelling of the tissue contrast agent uptake showed a minor decrease in ktrans (Δktrans = −0.017 ± 0.013 min−1, p value: 0.02), however no change in kep (Δkep = −0.022 ± 0.035 min−1, p value: 0.22) or ve (Δve = −0.089 ± 0.083, p value: 0.09) was found when averaged over the entire tumour including the treated volume in all animals (). Some inter‐animal variability was found in the increase in non‐perfused tumour volume (ktrans ≤ 0.04 min−1) (). On average, the non‐perfused volume increased by 116.4 ± 78.9 mm3 after tumour hyperthermia treatment (average tumour volume: 809 ± 285 mm3), which also affected the tumour averaged transfer constant ktrans.
Figure 6. T2‐weighted images, T1‐weighted DCE‐MRI images and parametric maps of ktrans [min− 1], kep [min−1] and ve [–] of the treated area before and after (A) hyperthermia treatment (data animal 2) and (B) ablation treatment of the tumour (data animal 7). A red ROI is manually drawn around the tumour in the parametric maps. The orange arrow points at local oedema visible after HIFU ablation treatment on the T2‐weighted scan. hyp, hyperthermia; ab, ablation.
![Figure 6. T2‐weighted images, T1‐weighted DCE‐MRI images and parametric maps of ktrans [min− 1], kep [min−1] and ve [–] of the treated area before and after (A) hyperthermia treatment (data animal 2) and (B) ablation treatment of the tumour (data animal 7). A red ROI is manually drawn around the tumour in the parametric maps. The orange arrow points at local oedema visible after HIFU ablation treatment on the T2‐weighted scan. hyp, hyperthermia; ab, ablation.](/cms/asset/a66fdc24-57fc-4b1e-b4de-c2f71f476c15/ihyt_a_648137_f0006_b.gif)
Figure 7. Change in pharmacologic parameters per animal study after HIFU treatment. For treatment parameters see .
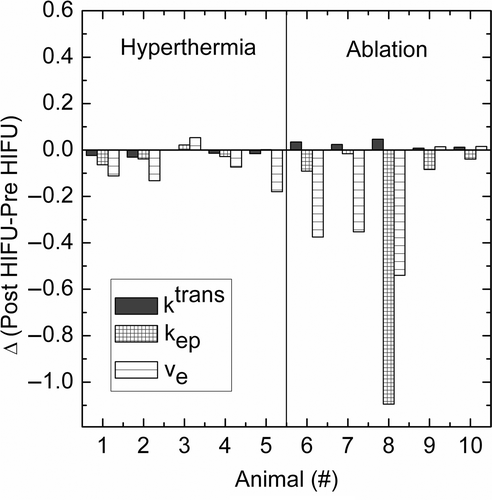
Histological analysis using NADH‐diaphorase staining for cell viability showed viable tumour tissue as well as viability of the muscle tissue surrounding the tumour (). On H&E staining, the hyperthermia‐treated tumour appeared similar to control tumour tissue; showing a tight packing of cell nuclei and a large amount of blood vessels ().
Figure 8. NADH‐diaphorase staining after (A) tumour hyperthermia and (B) tumour ablation. In the hyperthermia experiments, all tissue is viable. In the ablation experiments a clearly defined area of non‐viable tissue is visible. The black box indicates the areas from which the high magnification images were taken. v, viable tissue; n, non‐viable tissue.
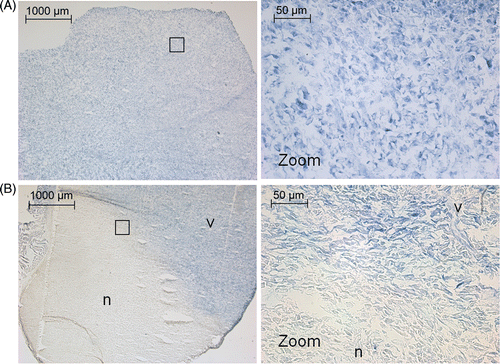
Tumour ablation
In the ablation experiments, the lethal thermal dose region perpendicular to the beam axis direction corresponded well with the dimensions of the treatment cell (diameter: 4 mm) (). Along the beam axis, comparison of the area of induced thermal damage to the treatment cell size could not be performed due to the spatial resolution of the temperature monitoring in that direction (three slices of 4.1 mm thickness, as compared to the treatment cell length of 10 mm). No correlation was found between the heating rate and the tumour‐to‐muscle signal intensity of T2‐weighted images (). Tumour ablation treatment and associated necrosis resulted in local oedema formation in the surrounding muscle, as visible on T2‐weighted images (change in signal intensity surrounding muscle: −33.6 ± 23.5%, ). The area of tissue necrosis in the tumour could not be distinguished on the T2‐weighted images (change in signal intensity tumour: 1.2 ± 3.6%).
Comparison of the DCE‐MR images before and after ablation treatment gave a good impression of the affected area. The effect of the ablation was largest on transfer constant ktrans, which showed a significant decrease when averaged over the entire tumour including the treated volume (Δktrans = −0.025 ± 0.016 min−1, p value: 0.03, ). Outside the treated volume, the ktrans did not show a significant change (Δktrans = 0.0062 ± 0.018 min−1, p value: 0.36). As a result, an increase in non‐perfused volume (NPV) of 371 ± 123 mm3 was found after ablation treatment using a 4‐mm diameter treatment cell ().
Two animals were allowed to recover after ablation to monitor the effect of the treatment on the animal well‐being and tumour growth. These animals were scanned using MR imaging again 4 days post therapy. Both animals showed shrinkage of the tumour volume compared to before treatment as determined from T2‐weighted MR images (animal 6: −493 mm3, animal 10: −73 mm3) and showed normal behaviour, including use of both legs. Partial tumour ablation was also seen in histological analysis with NADH‐diaphorase staining (). A sharp demarcation was found between viable and non‐viable tissue at the edge of the ablated region. NADH‐diaphorase staining of the muscle tissue directly surrounding the treated part of the tumour showed a superficial layer of necrosis in the muscle tissue, which extended up to 2 mm maximum into the muscle tissue. The effect of tumour ablation was poorly defined by H&E staining, showing varying results directly after tumour ablation (). Infiltration of neutrophiles into the tumour lesion was observed 4 days after ablation treatment, and cell damage was more pronounced in the treated part of the tumour ().
Discussion
The objective of this study was to develop a dedicated add‐on set‐up for MR‐HIFU treatment of rats in a clinical system and to show that the combined set‐up allows full use of the clinical MR‐HIFU features (e.g. temperature feedback, volumetric heating, binary feedback controlled hyperthermia) for small animals. These features have been extensively studied separately by others in rabbit Citation[22–24], pig Citation[18], Citation[43] and human subjects Citation[44] in clinical systems, and in rodents in dedicated small animal systems Citation[45]. Here, the combination of rodents treated in a clinical MR‐HIFU system is demonstrated.
Due to its robustness, PRF‐shift thermometry is the most widely used method for monitoring temperature changes during clinical MR‐HIFU therapy. However, the available MR receive coil of the clinical system was designed for use in the treatment of human uterine fibroids and did not provide sufficient image quality at the high resolution required for an accurate therapy assessment and temperature monitoring of the HIFU therapy in small animals. A dedicated HIFU‐compatible small animal MR coil was therefore developed, that can be mounted on the MR‐HIFU table top. This way, one order of magnitude image SNR improvement was obtained (increase from 21 to 258 for in vivo tumour tissue), which resulted in high resolution anatomical images and a high temperature accuracy at a spatial resolution sufficient for small animal temperature monitoring (1.4 × 1.4 × 4.1 mm3, three slices). Using the small animal coil, the SNR in muscle tissue remained sufficient for accurate temperature feedback (SNR 20, corresponding to a theoretical temperature uncertainty ΔT ≈ 0.3°C), as compared to the human coil set‐up (SNR 3.7, ΔT ≈ 1.7°C). In other words, to obtain the same SNR using the standard human coil set‐up as compared to the small animal coil, the voxel size would have to be increased to, for example, 3.5 × 3.5 × 8 mm3, which is far too large for use in small animal temperature monitoring. In clinical practice, a voxel size of 2.5 × 2.5 × 7 mm3 is used with the standard human coil set‐up, resulting in a relative temperature uncertainty of ΔT ≈ 0.5°C in a porcine thigh at 1.5 T Citation[18].
The actual measured temperature standard deviation was higher than the theoretical temperature uncertainty that could be expected based solely on the obtained SNR (in a phantom: 0.09°C compared to <0.01°C estimate; in vivo tumour: 0.17°C compared to <0.03°C estimate). This could indicate that at the high SNR obtained, other factors like motion, flow, and field drift become more important in determining the temperature uncertainty.
Fast parallel acquisition, enabled by the 4‐channel design of the coil, resulted in an acceptable temporal resolution of 5.0 s, despite the many phase encodings required for acquisition of high resolution images. Altogether, the thermometry measurements acquired with the small animal coil were sufficient for use in a previously described binary feedback algorithm Citation[37], which was here used for controlling the prolonged hyperthermia in a small treatment volume. The online calculated temperature information from the treatment cell was used to determine whether to continue sonicating and heat the target further or to briefly switch off the transducer power to allow the target tissue to slightly cool down. The use of this algorithm resulted in slow controlled fluctuation around a predefined temperature () without requiring a priori knowledge of tissue‐specific parameters such as perfusion, energy absorption, heat diffusion or attenuation.
To manage the entering and exiting of the ultrasound beam into the tissue, the target area was carefully positioned and submerged which formed a sufficiently large area for ultrasound contact. The animal was protected from far‐field heating using an acoustic absorber, which resulted in excellent animal body temperature management during treatment (36.8 ± 0.4°C). The latter is especially important for the development of protocols for hyperthermia‐induced local drug delivery from thermo‐sensitive carriers.
The current small animal set‐up was specifically designed to treat subcutaneous tumours on rat extremities. The similarity to clinical cases could be further improved by using a non‐subcutaneous tumour model. Although not specifically designed for it, the use of the HIFU‐compatible small animal coil could be extended to treat other body parts, e.g. liver or pancreas, of mice and rats. However, for treatment of such areas, the acoustic absorber cannot be used to eliminate far‐field heating. This will complicate treatment planning as well as tight regulation of the overall animal body temperature, especially in case of ablation experiments. Furthermore, respiratory and cardiac motion will become an issue in treating abdominal areas.
Coagulated tumour tissue could not be distinguished from viable tumour tissue based on T2‐weighted scans acquired up to 1 h post treatment, and T2‐weighted imaging is therefore not considered to be well‐suited for direct assessment of the success of tumour therapy in this rat model. However, T2‐weighted imaging was considered useful for general tumour demarcation, imaging of oedema, and corresponding inflammatory response after ablation treatment.
Cheng et al. Citation[46] described the use of permeability parameters ktrans and ve to predict subtle thermal histopathological change. Therefore, DCE‐MRI was here used as the method for direct therapy assessment. A 2‐parameter Tofts and Kermode model was used to estimate the kinetic parameters on a voxel‐by‐voxel basis. The total blood plasma volume (vp) was not taken into account in the DCE modelling, which may have affected the estimates of ktrans because the intravascular signal could have contributed to a large proportion to the observed tissue signal. However, in this work the kinetic parameters calculated from measurements in the same animal directly before and after treatment were compared. This was considered feasible since errors caused by the contribution of the arterial input function model would affect both estimates to the same extent. The contribution of the intravascular signal however, could have been affected by the HIFU ablation treatment. This effect was not taken into account.
DCE‐MRI was used to calculate the NPV of tumour tissue before and after treatment by setting a threshold for the transfer constant. Thirty minutes post hyperthermia treatment, the average values of the kinetic parameters across the tumour tissue showed a minor decrease in ktrans when the data were averaged over all treated animals (). As a result, an increase in NPV was found after hyperthermia treatment in some individual animals (). These results suggest that the vasculature was damaged or destroyed in some parts of the tumour, resulting in local signal decrease, and compensation in other parts of the tumour by an increased perfusion. The effect of hyperthermia treatment on tumour perfusion has been studied extensively. Most studies show an initial perfusion increase Citation[47], but there are also studies that report a decrease in perfusion after hyperthermia treatment which they explain by destroyed tumour vasculature due to local heating above the threshold for vascular damage Citation[48], Citation[49]. For example, heating to 43.5°C was shown to induce a perfusion increase during the first 30 min followed by a pronounced reduction in perfusion up to 24 h after treatment Citation[50].
All animal tumours that were treated with ablation showed a substantial increase in NPV (). The post‐treatment NPV depended on whether the heat diffusion region surrounding the treatment cell was located entirely within the tumour or partly outside the animal, as well as treatment duration (). Overall, the NPV varied between 43% and 88% of the pre‐treatment tumour volume. In this study, no long‐term follow‐up was performed after treatment. Instead, standard imaging methods like DCE‐MRI and T2‐weighted imaging together with histology were used to assess the treatment effect. Although long‐term follow‐up is a prerequisite to determine the therapy efficiency, the study presented here primarily aimed to prove functionality of the small animal set‐up and was not designed to look at long‐term effects of ablation and hyperthermia treatment.
Histology of the treated tissue was performed to analyse general morphology (H&E) and cell viability (NADH‐diaphorase). No tissue alterations were observed on H&E‐stained hyperthermia‐treated tissue. For ablated tumour tissue, H&E gave varying results and remained inconclusive for defining the coagulated area directly after therapy, probably due to local heat fixation of the treated tissue. The latter causes the treated tissue to appear normal directly after therapy, as previously observed in several clinical HIFU tumour therapy studies Citation[51], Citation[52]. Four days post therapy, the heat‐fixed region started to degrade and lose structure, and infiltration of neutrophiles into the lesion became visible on H&E staining (). NADH‐diaphorase staining showed cell viability for the muscles and tumours treated with hyperthermia, and the skin surrounding these areas, while identifying a clearly defined zone of coagulative necrosis after ablation. Due to the sharp demarcation between viable cells and coagulated tissue NADH‐diaphorase staining can be considered very useful in HIFU therapy assessment.
Conclusion
In this paper a method was presented for focused ultrasound treatment of rat tumours using a clinical MR‐HIFU system. To that aim, a dedicated HIFU‐compatible small animal MR coil and animal support were developed that could be used as add‐on to clinical MR‐HIFU systems. This approach provided all the advantages of clinical MR‐HIFU, such as volumetric heating, temperature feedback control and a clinical software interface for use in rodent treatment. Protocols for stable hyperthermia and ablation treatments of subcutaneous tumours were presented that can be used in future studies in large cohorts of animals for the development and optimisation of temperature‐induced drug delivery strategies as well as for finding optimal imaging methods for post therapy assessment of malignant tumours. The translation of the HIFU protocols and biomarkers from pre‐clinical research to human applications can be accelerated with the use of the clinical system during the pre‐clinical development.
Acknowledgements
The authors would like to thank Caren van Kammen, Maastricht University, for her help with the animal studies and her animal handling expertise. Furthermore the authors acknowledge Chatu Jayadewa (Therapeutic Ultrasound Team headed by Gail ter Haar, Institute for Cancer Research, Sutton, Surrey) for useful discussions on histology of US‐treated tissue. This research was performed within the framework of the Center for Translational Molecular Medicine (www.ctmm.nl), project VOLTA (grant 05T‐201) and within the framework of the European Commission project Sonodrugs (NMP4‐LA‐2008‐213706).
Declaration of interest: The authors Edwin Heijman, Max O. Köhler, Mika Ylihautala, Gösta J. Ehnholm, Arjan W. Simonetti, and Holger Grüll are employed by Philips.
References
- Cline HE, Schenck JF, Watkins RD, Hynynen K, Jolesz FA. Magnetic resonance‐guided thermal surgery. Magn Reson Med 1993; 30: 98–106
- Hynynen K, Freund WR, Cline HE, Chung AH, Watkins RD, Vetro JP, et al. A clinical, noninvasive, MR imaging‐monitored ultrasound surgery method. Radiographics 1996; 16: 185–195
- Hynynen K, Pomeroy O, Smith DN, Huber PE, McDannold NJ, Kettenbach J, et al. MR imaging‐guided focused ultrasound surgery of fibroadenomas in the breast: A feasibility study. Radiology 2001; 219: 176–185
- Hynynen K, Darkazanli A, Unger E, Schenck JF. MRI‐guided noninvasive ultrasound surgery. Med Phys 1993; 20: 107–115
- Rieke V, Butts Pauly K. MR thermometry. J Magn Reson Imaging 2008; 27: 376–390
- Ishihara Y, Calderon A, Watanabe H, Okamoto K, Suzuki Y, Kuroda K, et al. A precise and fast temperature mapping using water proton chemical shift. Magn Reson Med 1995; 34: 814–823
- ter Haar G. Therapeutic applications of ultrasound. Prog Biophys Mol Biol 2007; 93: 111–129
- Hill CR, Rivens I, Vaughan MG, ter Haar GR. Lesion development in focused ultrasound surgery: A general model. Ultrasound Med Biol 1994; 20: 259–269
- ter Haar G. Ultrasound focal beam surgery. Ultrasound Med Biol 1995; 21: 1089–1100
- Sapin‐de Brosses E, Gennisson JL, Pernot M, Fink M, Tanter M. Temperature dependence of the shear modulus of soft tissues assessed by ultrasound. Phys Med Biol 2010; 55: 1701–1718
- McDannold N. Quantitative MRI‐based temperature mapping based on the proton resonant frequency shift: Review of validation studies. Int J Hyperthermia 2005; 21: 533–546
- Yarmolenko PS, Moon EJ, Landon C, Manzoor A, Hochman DW, Viglianti BL, et al. Thresholds for thermal damage to normal tissues: An update. Int J Hyperthermia 2011; 27: 320–343
- Hindley J, Gedroyc WM, Regan L, Stewart E, Tempany C, Hynyen K, et al. MRI guidance of focused ultrasound therapy of uterine fibroids: Early results. Am J Roentgenol 2004; 183: 1713–1719
- Stewart EA, Rabinovici J, Tempany CM, Inbar Y, Regan L, Gostout B, et al. Clinical outcomes of focused ultrasound surgery for the treatment of uterine fibroids. Fertil Steril 2006; 85: 22–29
- Huber PE, Jenne JW, Rastert R, Simiantonakis I, Sinn HP, Strittmatter HJ, et al. A new noninvasive approach in breast cancer therapy using magnetic resonance imaging‐guided focused ultrasound surgery. Cancer Res 2001; 61: 8441–8447
- Schmitz AC, Gianfelice D, Daniel BL, Mali WP, van den Bosch MA. Image‐guided focused ultrasound ablation of breast cancer: Current status, challenges, and future directions. Eur Radiol 2008; 18: 1431–1441
- Salomir R, Delemazure AS, Palussiere J, Rouviere O, Cotton F, Chapelon JY. Image‐based control of the magnetic resonance imaging‐guided focused ultrasound thermotherapy. Top Magn Reson Imaging 2006; 17: 139–151
- Köhler MO, Mougenot C, Quesson B, Enholm J, Le Bail B, Laurent C, et al. Volumetric HIFU ablation under 3D guidance of rapid MRI thermometry. Med Phys 2009; 36: 3521–3535
- Yatvin MB, Weinstein JN, Dennis WH, Blumenthal R. Design of liposomes for enhanced local release of drugs by hyperthermia. Science 1978; 202: 1290–1293
- Weinstein JN, Magin RL, Yatvin MB, Zaharko DS. Liposomes and local hyperthermia: Selective delivery of methotrexate to heated tumors. Science 1979; 204: 188–191
- Rous P, Beard JW. The progression to carcinoma of virus‐induced rabbit papillomas (Shope). J Exp Med 1935; 62: 523–548
- Palussiere J, Salomir R, Le Bail B, Fawaz R, Quesson B, Grenier N, et al. Feasibility of MR‐guided focused ultrasound with real‐time temperature mapping and continuous sonication for ablation of VX2 carcinoma in rabbit thigh. Magn Reson Med 2003; 49: 89–98
- Staruch R, Chopra R, Hynynen K. Localised drug release using MRI‐controlled focused ultrasound hyperthermia. Int J Hyperthermia 2011; 27: 156–171
- Negussie AH, Yarmolenko PS, Partanen A, Ranjan A, Jacobs G, Woods D, et al. Formulation and characterisation of magnetic resonance imageable thermally sensitive liposomes for use with magnetic resonance‐guided high intensity focused ultrasound. Int J Hyperthermia 2011; 27: 140–155
- Choi JJ, Pernot M, Brown TR, Small SA, Konofagou EE. Spatio‐temporal analysis of molecular delivery through the blood–brain barrier using focused ultrasound. Phys Med Biol 2007; 52: 5509–5530
- Larrat B, Pernot M, Aubry JF, Dervishi E, Sinkus R, Seilhean D, et al. MR‐guided transcranial brain HIFU in small animal models. Phys Med Biol 2010; 55: 365–388
- Hu Z, Yang XY, Liu Y, Sankin GN, Pua EC, Morse MA, et al. Investigation of HIFU‐induced anti‐tumor immunity in a murine tumor model. J Transl Med 2007; 5: 34
- Chen X, Novak P, Benson DG, Jr, Webber JS, Hennings L, Shafirstein G, et al. An alternating focused ultrasound system for thermal therapy studies in small animals. Med Phys 2011; 38: 1877–1887
- Chopra R, Curiel L, Staruch R, Morrison L, Hynynen K. An MRI‐compatible system for focused ultrasound experiments in small animal models. Med Phys 2009; 36: 1867–1874
- Payne A, Todd N, Bever Jd, Volland N, Vyas U, Shea J, et al. Initial experiece with a small animal system for MRgFUS taregeted drug delivery. Second International Symposium MR-guided Focused Ultrasound 2010. Washington, DC 2010
- Conturo TE, Smith GD. Signal‐to‐noise in phase angle reconstruction: Dynamic range extension using phase reference offsets. Magn Reson Med 1990; 15: 420–437
- de Smet M, Heijman E, Langereis S, Hijnen NM, Grull H. Magnetic resonance imaging of high intensity focused ultrasound mediated drug delivery from temperature‐sensitive liposomes: An in vivo proof‐of‐concept study. J Control Release 2011; 150: 102–110
- Pruessmann KP, Weiger M, Scheidegger MB, Boesiger P. SENSE: Sensitivity encoding for fast MRI. Magn Reson Med 1999; 42: 952–962
- Köhler MO, Tillander M, Syriä A, Nakari R, Yilhautala M. Ultrasound-transparent RF coil design for improved MR thermometry of HIFU therapy. International Society for Magnetic Resonance in Medicine (ISMRM) poster 1728. Canada, Montréal 2011
- Wang HZ, Riederer SJ, Lee JN. Optimizing the precision in T1 relaxation estimation using limited flip angles. Magn Reson Med 1987; 5: 399–416
- Sapareto SA, Dewey WC. Thermal dose determination in cancer therapy. Int J Radiat Oncol Biol Phys 1984; 10: 787–800
- Enholm JK, Köhler MO, Quesson B, Mougenot C, Moonen CT, Sokka SD. Improved volumetric MR‐HIFU ablation by robust binary feedback control. IEEE Trans Biomed Eng 2010; 57: 103–113
- Tofts PS, Kermode AG. Measurement of the blood–brain barrier permeability and leakage space using dynamic MR imaging. 1. Fundamental concepts. Magn Reson Med 1991; 17: 357–367
- McGrath DM, Bradley DP, Tessier JL, Lacey T, Taylor CJ, Parker GJ. Comparison of model‐based arterial input functions for dynamic contrast‐enhanced MRI in tumor bearing rats. Magn Reson Med 2009; 61: 1173–1184
- Anderson JK, Baker M, Jaffers O, Pearle MS, Lindberg GL, Cadeddu JA. Time course of nicotinamide adenine dinucleotide diaphorase staining after renal radiofrequency ablation influences viability assessment. J Endourol 2007; 21: 223–227
- Denis de Senneville B, Quesson B, Moonen CT. Magnetic resonance temperature imaging. Int J Hyperthermia 2005; 21: 515–531
- Salomir R, Palussiere J, Vimeux FC, de Zwart JA, Quesson B, Gauchet M, et al. Local hyperthermia with MR‐guided focused ultrasound: Spiral trajectory of the focal point optimized for temperature uniformity in the target region. J Magn Reson Imaging 2000; 12: 571–583
- Quesson B, Laurent C, Maclair G, de Senneville BD, Mougenot C, Ries M, et al. Real‐time volumetric MRI thermometry of focused ultrasound ablation in vivo: A feasibility study in pig liver and kidney. NMR Biomed 2011; 24: 145–153
- Kim YS, Lim HK, Kim JH, Rhim H, Park BK, Keserci B, et al. Dynamic contrast-enhanced magnetic resonance imaging predicts immediate therapeutic response of magnetic resonance-guided high-intensity focused ultrasound ablation of symptomatic uterine fibroids. Invest Radiol 2011
- Guilhon E, Voisin P, de Zwart JA, Quesson B, Salomir R, Maurange C, et al. Spatial and temporal control of transgene expression in vivo using a heat‐sensitive promoter and MRI‐guided focused ultrasound. J Gene Med 2003; 5: 333–342
- Cheng HL, Purcell CM, Bilbao JM, Plewes DB. Prediction of subtle thermal histopathological change using a novel analysis of Gd‐DTPA kinetics. J Magn Reson Imaging 2003; 18: 585–598
- Kong G, Braun RD, Dewhirst MW. Characterization of the effect of hyperthermia on nanoparticle extravasation from tumor vasculature. Cancer Res 2001; 61: 3027–3032
- Ludemann L, Wust P, Gellermann J. Perfusion measurement using DCE‐MRI: Implications for hyperthermia. Int J Hyperthermia 2008; 24: 91–96
- Dings RP, Loren ML, Zhang Y, Mikkelson S, Mayo KH, Corry P, et al. Tumour thermotolerance, a physiological phenomenon involving vessel normalisation. Int J Hyperthermia 2011; 27: 42–52
- Song CW, Lin JC, Chelstrom LM, Levitt SH. The kinetics of vascular thermotolerance in SCK tumors of A/J mice. Int J Radiat Oncol Biol Phys 1989; 17: 799–802
- Wu F, Wang ZB, Cao YD, Xu ZL, Zhou Q, Zhu H, et al. Heat fixation of cancer cells ablated with high‐intensity‐focused ultrasound in patients with breast cancer. Am J Surg 2006; 192: 179–184
- Leslie TA, Kennedy JE, Illing RO, Ter Haar GR, Wu F, Phillips RR, et al. High‐intensity focused ultrasound ablation of liver tumours: Can radiological assessment predict the histological response?. Br J Radiol 2008; 81: 564–571