Abstract
There is an increasing clinical need for novel, externally triggered, local drug delivery methods since many drugs are characterised by high systemic toxicity and/or suffer from limited bioavailability. Thermosensitive liposomes (TSLs) demonstrate great potential for local delivery and release of the payload. They are particularly effective in combination with ultrasound (US) under magnetic resonance (MR) guidance that is known to induce hyperthermia locally in a precisely controlled manner. Bioeffects of hyperthermia include increased blood flow, blood volume and enhanced vascular permeability thus potentiating anti-tumoural efficacy of TSLs. However, bioeffects of ultrasound are not limited to hyperthermia only, and under certain parameters may include locally enhanced extravasation and permeabilisation of cell membrane for compounds. This opens up opportunities for delivery of cell-impermeable and weakly permeable drugs that can be employed together with thermosensitive liposomes. In the latter case US-mediated cavitation and/or radiation forces local permeabilisation of the cells and US-induced hyperthermia releases the drug. This review focuses on bioeffects of US such as hyperthermia, enhanced extravasation and cell permeabilisation utilised for local drug delivery.
Introduction
Externally triggered local drug delivery represents a promising approach for increasing drug efficacy and therapeutic window while reducing systemic toxicity. Hyperthermia is being extensively exploited as an external trigger for drug delivery with responsive nanocarriers Citation[1]. It can be achieved with water bath Citation[2], catheter Citation[3], radiofrequency Citation[4] or microwave radiation Citation[5]. However, its non-invasiveness, high degree or spatial precision and possibility of image-guidance make focused ultrasound (FUS) one of the most promising tools to generate hyperthermia in clinical setting Citation[1], Citation[6–8].
Thermosensitive liposomes (TSLs) release encapsulated drug in response to hyperthermia near their phase transition temperature (Tm), where the lipid membrane undergoes a transition from a gel to a liquid crystalline phase Citation[9–11]. They overcome traditional delivery methods by increasing drug targeting to diseased tissue while decreasing exposure to healthy organs Citation[12]. For example, doxorubicin-loaded Citation[2], Citation[13] and paclitaxel (taxol)-loaded TSLs Citation[14], Citation[15] demonstrated improved efficacy in preclinical studies in terms of both drug bioavailability and anti-tumour effect. Cisplatin-containing liposomes demonstrated significantly improved delivery to the brain in rat and canine models Citation[16], Citation[17]. Lyso-thermosensitive doxorubicin-containing formulation (Thermodox) is currently in clinical trials, including a completed phase I study for primary or metastatic liver tumours Citation[18], an ongoing 600-patient hepatocellular carcinoma phase III study Citation[19] and a pivotal phase I/II open-label, dose-escalating trial for recurring chest-wall breast cancer.
Ultrasound has multiple applications in the field of drug delivery including transdermal delivery, delivery to solid tumours, delivery across the blood–brain barrier and enhanced thrombolysis Citation[20]. Depending on the energy, ultrasound interaction with biological tissues is mediated through thermal (heat) and non-thermal (acoustic cavitation and radiation forces) effects. Non-thermal effects of US trigger a variety of biological responses resulting in increased drug or carrier extravasation Citation[21], Citation[22], blood–brain barrier (BBB) disruption Citation[23], Citation[24] and membrane permeabilisation to otherwise cell-impermeable compounds Citation[25–27].
As pointed out above, ultrasound provides two different stimuli depending on the acoustic parameters (i.e. frequency, pressure amplitude, and duty cycle) leading to either a strong pressure pulse in the focus area or a temperature increase, or both Citation[28], Citation[29]. Both stimuli can be exploited for drug delivery Citation[20]. The objective of this review is to summarise the applications of US to the drug delivery field, focusing on hyperthermia-based strategies, non-thermal effects-based strategies, and the possible combination of the two.
Thermal effects of ultrasound
Tissue ablation and chemotherapy
FUS is currently used in the clinic for non-invasive treatment of uterine fibroids and prostate tumours by generating coagulative necrosis resulting in thermal ablation of the diseased tissue Citation[30]. It is under investigation for treatment of other malignancies such as gliomas Citation[31], breast cancer Citation[32], liver cancer Citation[33], Citation[34] and extra-abdominal desmoid tumours Citation[35]. During thermal ablation continuous ultrasound pulses of the order of seconds are applied and temperatures around 60–70°C are reached in the ultrasound focal volume. However, in many cases due to the difficulties in thermal dose spatial positioning, the margins of the tumour are not ablated, which allows some tumour cells to escape, which results in tumour recurrence Citation[36], Citation[37]. Thus ablation as an adjunct to systemic chemotherapy is a non-surgical option of combining systemic and local treatment for metastatic disease and is a safe treatment with a low risk profile, for example for colorectal pulmonary metastasis Citation[38]. Only a small number of preclinical studies to date have been reported where high-intensity focused ultrasound (HIFU) exposures, as a source of hyperthermia, were combined with thermosensitive liposomes Citation[29]. In one study, TSLs were loaded with a paramagnetic contrast agent, where the triggering temperature of the liposomes was set to thermal ablation levels (i.e. approximately 57°C). Using an MR-guided HIFU system in combination with the TSLs and imaging for T1-weighted signal intensity enhancement, the authors demonstrated that they could non-invasively validate that indeed temperature elevations were achieved for ablating the targeted tissue Citation[7].
Mild hyperthermia
Heat generation can be significantly reduced when using pulsed FUS (from 10–50 ms) by allowing tissue to cool down between US exposures Citation[39]. Despite the use of high energy (1000–2000 W/cm2) such exposure results in mild hyperthermia (3–5°C temperature elevation) Citation[40]. Hyperthermia is successfully employed in clinical trials in combination with chemotherapy for relapsing ovarian cancer Citation[41], localised high-risk soft tissue sarcoma Citation[42] and locally advanced breast carcinoma Citation[43] patients. In tumours, mild hyperthermia leads to increased blood flow and enhanced vascular permeability as reviewed in Citation[44]. For example tumour blood volume has been found to increase 1.5–2-fold compared to controls after 30 min of mild hyperthermia treatment Citation[45] and was consistent with increased tumour oxygenation Citation[46] that was maximal at temperatures 40–42°C. Tumour vessels are characterised by chaotic structure and numerous ‘dead ends’ resulting in a reduced blood flow and which consequently hampers nanocarrier distribution in the tumour vascular compartment and poor exposure to the tumour tissue Citation[47]. Thus augmented by hyperthermia, blood flow facilitates the delivery of nanocarriers to tumours Citation[48]. This effect is supported by the positive influence of mild hyperthermia on tumour microvascular permeability. Hyperthermia-induced nanoparticle extravasation is size-dependent and does not affect compounds smaller than the size of vessel pores Citation[49]. Lefor and co-workers have investigated the effects of hyperthermia on vascular permeability to Evans blue in a liver metastasis rat model Citation[50]. Although no changes were observed at 40°C, heating to 43°C (still within the therapeutic range of hyperthermia) had resulted in a significant increase in vascular permeability. However, in some studies no increased extravasation of macromolecules has been registered after hyperthermia, as in reports of Song and co-workers regarding albumin Citation[51] and of Gerlowski and Jain regarding 150 kDa dextran Citation[52]; 100 nm liposomes were investigated for extravasation under hyperthermic conditions. Kong and co-workers have shown that the degree of extravasation had increased with temperature from 40°C to 42°C and lasted for up to 6 h Citation[53]. Interestingly, no extravasation was observed after re-heating, suggesting the development of vascular thermotolerance. In another study of extravasation of 100 nm nanoparticles mediated by hyperthermia (42°C) Liu and co-workers observed heterogeneous character of nanoparticle distribution with large amounts of liposomes extravasated at the edge of the tumour where angiogenesis was the most active Citation[54]. Newly formed vessels at the tumour periphery have been found to be more sensitive to heat due to the absence of connective tissue Citation[55] and an increased secretion of vascular endothelial growth factor (VEGF) Citation[56]. However hyperthermia affects extravasation from the normal vessels as well. A study performed on human umbilical vein endothelial cells (hUVECs) subjected to hyperthermia demonstrated a significant and reversible increase in permeability compared to untreated hUVECs Citation[57]. It was consistent with a marked decrease in VE-cadherin staining and contraction of F-actin at sites of endothelial cell–cell separation. Altogether these data suggest that hyperthermia contributes to a rapid and reversible change in endothelial cell permeability in association with a down regulation of VE-cadherin. There is evidence that in vivo hyperthermia affects other organs and tissues as well. Matthew and co-workers Citation[58] have quantified extravasation in the heart, liver, lung, kidney, spleen, gastrocnemius, and duodenum in normothermic and hyperthermic conditions (core temperature (Tc) = 41.5°, 42°, or 42.6°C) in rats. They have observed significantly higher level of Evans blue in the tissues of severely hyperthermic (42.6°C) animals compared to control ones.
Principles guiding the use of lysolipid-thermosensitive liposomes are illustrated in . Once in systemic circulation they may benefit from enhanced permeability retention (EPR) effect and accumulate in the tumour. It is also possible to opt for a release shortly after injection to take advantage of the maximum liposomal concentration in blood. Upon heating to mild hyperthermia (in general 39°–42°C) increased tumour blood volume and vascular permeability facilitate extravasation. As a result, high concentration of the drug is delivered locally. Depending on the timing and liposomes employed, part of the cargo may be released in the vasculature; however, due to the local character of hyperthermia overall conditions are still favourable for tumour uptake. Release protocols can be fine-tuned in function not only of carrier but also cargo: anti-angiogenic drugs incorporated into liposomes would benefit from intravascular release while cytotoxic drugs would benefit from the release to the interstitial space. Recent studies using MRI-controlled FUS and thermosensitive liposomes to achieve thermally mediated localised drug delivery in vivo under mild hyperthermia include those performed by Negussie et al. Citation[8], de Smet et al. Citation[59] and Staruch et al. Citation[60]. In these studies various liposome formulations co-loaded with doxorubicin and an MR contrast agent were investigated. Stability, imageability, and MR-HIFU monitoring and control of content release suggest that MR-HIFU combined with these imageable thermosensitive liposomes may enable real-time monitoring and spatial control of content release.
Non-thermal effects of ultrasound
Non-thermal effects of ultrasound are mediated through acoustic cavitation (that is the formation and/or activity of gas-filled bubbles in a medium exposed to US Citation[61] and non-cavitational factors such as radiation and shear forces. The sustained growth of cavitation bubbles and their oscillations over several acoustic cycles is known as stable cavitation, whereas the violent growth and immediate collapse, when using a peak pressure approaching 1 MPa for clinically used microbubbles such as SonoVue (Bracco Diagnostics, Milan, Italy), is called inertial cavitation Citation[62]. Intensity of cavitation is proportional to the number of stabilised gas bubbles (cavitation nuclei) that can be either intrinsic or from an external source (such as microbubbles). The collapse of cavitating bubbles is thought to be the major factor affecting cell permeability, while their stable oscillation is considered to be responsible for enhanced extravasation Citation[29]. However, recent works have emphasised the role of non-cavitational mechanisms behind US-mediated bioeffects. Hancock and co-workers Citation[22] have shown that pulsed HIFU exposures are capable of generating non-destructive and reversible structural changes in the muscle for enhancing both extravasation and interstitial transport of large (i.e. 100 nm) nanospheres, being on the same scale as liposomal drug carriers and viral vectors. Acoustic cavitation alone does not seem to be the only non-thermal mechanism affecting drug delivery and uptake. Acoustic radiation forces have been suggested as the basis for a possible ultrasound mechanism for delivery enhancement Citation[22]. Recently, Krasovitski and co-workers have suggested that the bilayer membrane is capable of directly transforming acoustic energy into mechanical stresses and strains at the subcellular and cellular level, which do not require a prior existence of air voids in the tissue Citation[63]. In an attempt to unify the theories behind the non-thermal bioeffects of US, they suggest that many of the latter could potentially be interpreted in light of the ‘bilayer sonophore’ (BLS) model as progressive stages along a graded scale of induced phenomena that differ in a maximum area strain due to different ultrasound exposure parameters, mechanical tissue properties, proximity to a free surface, or the presence of extracellular gas bubbles, such as ultrasound contrast agents that were administered systemically. Some of bioeffects of US (membrane poration, membrane permeabilisation) are of a particular interest for drug delivery. Their application is discussed below.
Blood–brain barrier disruption
Blood–brain barrier, a composite of highly specialised endothelial and perivascular structures, severely limits transport of molecules into the brain. Focused ultrasound is capable of inducing non-invasive (through intact skull) and reversible disruption of BBB Citation[23], Citation[24]. Either pulsed HIFU or low intensity FUS with microbubbles are commonly considered for the treatment of brain tumours Citation[64], Citation[65]. Another possible application of US-mediated transient BBB disruption is the treatment of central nervous system pathologies such as Alzheimer's disease. Raymond and co-workers have administered intravenous trypan blue, an amyloid staining red fluorophore, and anti-amyloid antibodies, concurrently with focused ultrasound therapy in plaque-bearing, transgenic mouse models of Alzheimer's disease with amyloid pathology Citation[66]. MRI guidance permitted selective treatment and monitoring of plaque-heavy anatomical regions, such as the hippocampus. Treated brain regions exhibited a 16.5-fold increase in trypan blue fluorescence and 2.7-fold increase in anti-amyloid antibodies that localised to amyloid plaques. Ultrasound-enhanced delivery was consistently reproduced in two different transgenic strains, across a large age range (9–26 months), with and without MR guidance, and with little or no tissue damage. The mechanism by which delivery enhancement occurs is not precisely known. It is hypothesised that the stably oscillating cavitating bubbles generate mechanical stress in the adjacent blood vessel walls, while causing little or no damage to brain tissue, and in that manner increase vascular permeability both through structural and physiological processes Citation[23]. As a consequence, the BBB is reversibly disrupted, allowing extravasation of therapeutic agents Citation[29].
Enhanced extravasation
In many cases stable (non-inertial) cavitation is thought to be responsible for enhancing the permeability of blood vessels through the generation of local streaming and consequent shear that can open up gaps between endothelial cells Citation[22]. This property of FUS was used to deliver high-molecular weight dextran- and doxorubicin-containing thermosensitive liposomes Citation[67], plasmid DNA Citation[21] and monoclonal antibodies Citation[68] in a non-destructive manner. Interestingly, in some studies the cavitation that was not reported to be stable also resulted in successful β-galactosidase reporter gene delivery in vivo [69]. Two recent studies demonstrated that the HIFU effect on the extravasation of fluorescently labelled polystyrene nanospheres in murine muscle can last for 24 h Citation[19] or even up to 72 h Citation[20] after the exposure. These authors suggested that other less known mechanisms, possibly acoustic radiation forces rather than acoustic cavitation alone, may be responsible for enhancement of extravasation and interstitial transport in the tissue.
Enhanced internalisation
US cavitation can be used to alter the permeability of individual cells for improved delivery of genes and drugs at the level of individual cells Citation[29]. Multiple studies suggest that US and microbubbles facilitate the formation of the transient pores in the plasma membrane via cavitation. These pores were known to spontaneously reseal within a short time – seconds to minutes Citation[70–72]. However, in a recent study using intercalating dyes as ‘sensors’ for US-mediated internalisation,Yudina et al. have shown that the cell membrane can be reversibly permeabilised by US for at least 8 h with residual permeability lasting for up to 24 h. The debate about the mechanisms responsible for the US-mediated uptake is still ongoing. With thermal mechanism mostly ruled out Citation[22], in particular under the conditions of this study, cavitational or non-cavitational forces such as acoustic radiation force are possible explanations. Together or separately, they lead to enhancement of endocytosis Citation[73], Citation[74], transient hydrophilic pore formation Citation[71], and actively repairable ‘wounds’ in the cell membrane Citation[72]. The present study did not focus on elaborating the exact mechanism of US-mediated drug delivery; however, it may bring some useful considerations into account. Resulting nuclear staining suggests cytosolic delivery. On the other hand, if the hydrophilic pore or ‘wound’ formation does contribute under the conditions used, pores appear to exist for much longer than previously thought, since studies mentioning pore formation refer to short-lived pores with permeability enhancement lasting from several seconds to several minutes.
Combination of US-mediated permeabilisation and hyperthermia for delivery of cell-impermeable compounds
Much attention has been paid to the ‘combination’ approaches involving various US-induced bioeffects and therapeutic options, such as hyperthermia as adjuvant to chemotherapy Citation[75], Citation[76]. In a recent in vitro study antigen-dependent effector T cell activity has been found to be enhanced following mild hyperthermia which might be related to immunomodulatory function of hyperthermia Citation[77]. Other informative studies combining thermal and non-thermal effects of ultrasound for tumour ablation and gene delivery Citation[78] as well as multi-step approaches involving combinations of hyperthermia, chemotherapy and vaccination Citation[79] have been reported.
Recently we have suggested a new approach to combine pressure and temperature-mediated drug delivery strategies into one protocol (). This two-step protocol can be applied to drugs that benefit from temperature-induced liposomal delivery in terms of protection against plasma degradation, increased plasma half-life and high targeted concentration, while the pressure-mediated delivery step facilitates drug uptake into the cell. Multiple drug classes may gain from encapsulation into TSLs in terms of increase in half-life and protection from plasma degradation. Upon the cargo release the abovementioned systems primarily rely on therapeutic agent internalisation by passive diffusion to reach the nucleus. However, in the case of drugs (such as doxorubicin) that readily cross the plasma membrane, exposure of the healthy tissue is still not negligible, especially if the drug is released into the bloodstream. Drugs that do not cross the plasma membrane could readily circumvent this problem if a way to facilitate their internalisation locally could be established. Nucleic acids (pDNA or siRNA Citation[80]) and some chemotherapeutic agents, for example cisplatin and bleomycin Citation[81] are among the therapeutic molecules with intracellular mode of action that are not readily bioavailable, e.g. are not internalised into cells efficiently enough. In fact, of the 61 orally administered drugs on the Model list of Essential Medicines of the World Health Organization (WHO) that could be classified with certainty by their solubility/permeability properties, 24 (39%) belong to class III (highly soluble, poorly permeable) and 6 (10%) to class IV (poorly soluble, poorly permeable) Citation[82]. It suggests that their performance may be significantly improved and/or the dose could be reduced by local cell permeabilisation at the site of disease. Moreover, in the process of drug discovery in oncology, the development of many promising candidates is hampered by their poor bioavailability. Novel protocols may give a chance to cell-impermeable or poorly permeable candidates that are highly efficient when internalised locally at the tumour site. In a proof of concept in vitro study Citation[83] an example protocol for a two-step delivery has been demonstrated using the dye TO-PRO-3 as a model drug. TO-PRO-3 belongs to the class of DNA-intercalating agents that becomes fluorescent upon interaction with the nucleic acids. Due to its charge, it is not able to cross the intact lipid bilayer of viable cells and therefore may be employed as a ‘sensor’ for the nuclear delivery Citation[25], Citation[27]. For the first step, TO-PRO-3 is encapsulated into temperature sensitive liposomes that are stably encapsulating the dye at T = 37°C in the inner lumen and release it at temperatures above 40°C. In a second step, microbubbles are cavitated by ultrasound inducing a permeabilisation of the cellular membrane for TO-PRO-3. We show with fluorescence microcopy that the combination of both steps leads to delivery of TO-PRO-3 into the cytosol and subsequently to the nucleus (). An important aspect to consider is the timing of the individual steps. In above-mentioned work, the temperature-mediated release step was applied first, followed by a pressure-mediated delivery step. Here, the drug is released from its carrier but is still outside the cell. The possible time window until the pressure-mediated cell permeabilisation needs to be applied is determined by the half-life of the drug outside of the cell. The amount of available free drug can rapidly decrease due to degradation, adsorption to other compounds present (e.g. albumin) or – under in vivo conditions – wash-out from the interstitial space. It will therefore be beneficial to apply the pressure-mediated step as quickly as possible after the temperature-mediated release step. Though the actual in vivo experiment was not performed here, recent studies that probed the time window of ultrasound-induced cell permeability suggest that the order of the two steps could be reversed Citation[27]. In vitro cell uptake studies with TO-PRO-3 showed that under certain conditions the pressure-induced permeability of tumour cells can last for several hours. If these results are translatable to the in vivo situation, tissue and cells could be permeabilised first using ultrasound in combination with microbubbles, before the temperature-induced delivery step. Considering the above-mentioned points, several variations of the proposed two-step drug delivery approach are possible. For applications in oncology, a protocol could comprise a drug encapsulated in a temperature-sensitive liposome that subsequently accumulates in the tumour utilising the EPR effect. Next, mild hyperthermia is applied to release the drug followed by intravascular administration of microbubbles followed by ultrasound treatment to mediate cellular uptake. The open question here is whether microbubbles with a diameter of 1–5 µm confined to the vasculature are able to mediate drug uptake in the tumour cells at some distance from the microvessels. There are some indications from pDNA delivery that this is possible Citation[84]. Another protocol may aim for improved extravasation of the drug carrier facilitated by ultrasound, followed by a temperature-controlled release in the tumour tissue. Ultrasound can contribute to the EPR effect by opening the endothelial junctions. Literature studies have shown that the effect of pulsed high intensity focused ultrasound on the extravasation of polystyrene nanospheres persists for more than 24 h Citation[85] or even 72 h Citation[22] after application of ultrasound. In this case, the pressure-mediated step would first open endothelial junctions to mediate extravasation and facilitate long-lived permeability of tumour cells while the subsequent heating releases the drug from the liposomes.
Figure 2. Schematic representation of US-mediated intracellular drug delivery utilizing temperature-sensitive liposomes (step 1) and membrane permeabilization in the presence of microbubbles (step 2).
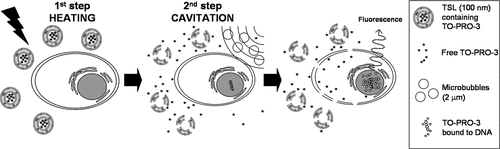
Figure 3. Epifluorescence imaging of two-step delivery. Only the combination of two consecutive steps – heating and sonication in presence of Sonovue microbubbles lead to the model drug TO-PRO-3 release from the thermosensitive liposomes and its intracellular uptake (visible as nuclear staining, H, exposure time 100 ms). Under other conditions the dye either remained encapsulated in the liposomes (A-D, exposure time 500 ms) or was not able to enter the cytoplasm and diffuse to the nucleus (E-G, exposure time 500 ms). (From Yudina et al, J Contr Release 2011).
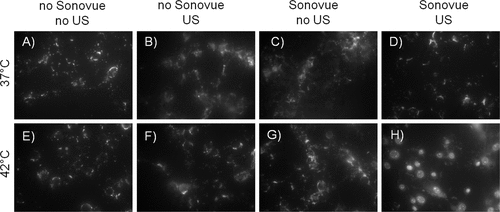
Conclusion
The bioeffects of US-mediated hyperthermia and cavitation, useful for local drug delivery, have been discussed in this review. Thermosensitive liposomes releasing the contents at mild hyperthermia conditions hold great promise in local treatment of cancer, cardio-vascular and central nervous system pathologies. FUS under MRI guidance provides excellent means for the spatio-temporal control of heating and imaging of drug release when MRI contrast agents are co-released. In addition, another property of US, namely the ability to permeabilise cell membranes to previously non-permeable compounds, is also being exploited extensively for local drug delivery. Combining these two approaches for delivery of cell-impermeable or weakly cell-permeable drugs encapsulated into thermosensitive liposomes may even further reduce systemic toxicity while boosting efficacy. In this scenario, targeted cells are first permeabilised by US and then heated to release the liposome contents. Further research is needed to evaluate which compounds benefit most from such two-step delivery. A limitation of these novel protocols is that the tumour has to be visualised first in order to target it with US.
Declaration of interest: This work was supported by EU project SonoDrugs (FP7-NMP4-LA-2008-213706) and ERC project 268906 ‘Sound Pharma’. The authors alone are responsible for the content and writing of the paper.
References
- Deckers R, Moonen CT. Ultrasound triggered, image guided, local drug delivery. J Control Release 2010; 148: 25–33
- Kong G, Anyarambhatla G, Petros WP, Braun RD, Colvin OM, Needham D, et al. Efficacy of liposomes and hyperthermia in a human tumor xenograft model: Importance of triggered drug release. Cancer Res 2000; 60: 6950–6957
- Viglianti BL, Abraham SA, Michelich CR, Yarmolenko PS, MacFall JR, Bally MB, et al. In vivo monitoring of tissue pharmacokinetics of liposome/drug using MRI: Illustration of targeted delivery. Magn Reson Med 2004; 51: 1153–1162
- Bos C, Lepetit-Coiffe M, Quesson B, Moonen CT. Simultaneous monitoring of temperature and T1: Methods and preliminary results of application to drug delivery using thermosensitive liposomes. Magn Reson Med 2005; 54: 1020–1024
- Khoobehi B, Peyman GA, Niesman MR, Oncel M. Hyperthermia and temperature-sensitive liposomes: Selective delivery of drugs into the eye. Jpn J Ophthalmol 1989; 33: 405–412
- Dromi S, Frenkel V, Luk A, Traughber B, Angstadt M, Bur M, et al. Pulsed high intensity focused ultrasound and low temperature-sensitive liposomes for enhanced targeted drug delivery and antitumor effect. Clin Cancer Res 2007; 13: 2722–2727
- McDannold N, Fossheim SL, Rasmussen H, Martin H, Vykhodtseva N, Hynynen K. Heat-activated liposomal MR contrast agent: Initial in vivo results in rabbit liver and kidney. Radiology 2004; 230: 743–752
- Negussie AH, Yarmolenko PS, Partanen A, Ranjan A, Jacobs G, Woods D, et al. Formulation and characterisation of magnetic resonance imageable thermally sensitive liposomes for use with magnetic resonance-guided high intensity focused ultrasound. Int J Hyperthermia 2011; 27: 140–155
- Yatvin MB, Weinstein JN, Dennis WH, Blumenthal R. Design of liposomes for enhanced local release of drugs by hyperthermia. Science 1978; 202: 1290–1293
- Needham D, Anyarambhatla G, Kong G, Dewhirst MW. A new temperature-sensitive liposome for use with mild hyperthermia: Characterization and testing in a human tumor xenograft model. Cancer Res 2000; 60: 1197–1201
- Sandstrom MC, Ickenstein LM, Mayer LD, Edwards K. Effects of lipid segregation and lysolipid dissociation on drug release from thermosensitive liposomes. J Control Release 2005; 107: 131–142
- Bikram M, West JL. Thermo-responsive systems for controlled drug delivery. Expert Opin Drug Deliv 2008; 5: 1077–1091
- Tagami T, Ernsting MJ, Li SD. Efficient tumor regression by a single and low dose treatment with a novel and enhanced formulation of thermosensitive liposomal doxorubicin. J Control Release 2011; 152: 303–309
- Sharma D, Chelvi TP, Kaur J, Ralhan R. Thermosensitive liposomal taxol formulation: Heat-mediated targeted drug delivery in murine melanoma. Melanoma Res 1998; 8: 240–244
- Nie S, Hsiao WL, Pan W, Yang Z. Thermoreversible Pluronic F127-based hydrogel containing liposomes for the controlled delivery of paclitaxel: In vitro drug release, cell cytotoxicity, and uptake studies. Int J Nanomedicine 2011; 6: 151–166
- Kakinuma K, Tanaka R, Takahashi H, Sekihara Y, Watanabe M, Kuroki M. Drug delivery to the brain using thermosensitive liposome and local hyperthermia. Int J Hyperthermia 1996; 12: 157–165
- Aoki H, Kakinuma K, Morita K, Kato M, Uzuka T, Igor G, et al. Therapeutic efficacy of targeting chemotherapy using local hyperthermia and thermosensitive liposome: Evaluation of drug distribution in a rat glioma model. Int J Hyperthermia 2004; 20: 595–605
- Poon RT, Borys N. Lyso-thermosensitive liposomal doxorubicin: A novel approach to enhance efficacy of thermal ablation of liver cancer. Expert Opin Pharmacother 2009; 10: 333–343
- Poon RT, Borys N. Lyso-thermosensitive liposomal doxorubicin: An adjuvant to increase the cure rate of radiofrequency ablation in liver cancer. Future Oncol 2011; 7: 937–945
- Ferrara KW. Driving delivery vehicles with ultrasound. Adv Drug Deliv Rev 2008; 60: 1097–1102
- Dittmar KM, Xie J, Hunter F, Trimble C, Bur M, Frenkel V, et al. Pulsed high-intensity focused ultrasound enhances systemic administration of naked DNA in squamous cell carcinoma model: Initial experience. Radiology 2005; 235: 541–546
- Hancock HA, Smith LH, Cuesta J, Durrani AK, Angstadt M, Palmeri ML, et al. Investigations into pulsed high-intensity focused ultrasound-enhanced delivery: Preliminary evidence for a novel mechanism. Ultrasound Med Biol 2009; 35: 1722–1736
- Hynynen K. Focused ultrasound for blood–brain disruption and delivery of therapeutic molecules into the brain. Expert Opin Drug Deliv 2007; 4: 27–35
- Hynynen K. Ultrasound for drug and gene delivery to the brain. Adv Drug Deliv Rev 2008; 60: 1209–1217
- Hallow DM, Mahajan AD, Prausnitz MR. Ultrasonically targeted delivery into endothelial and smooth muscle cells in ex vivo arteries. J Control Release 2007; 118: 285–293
- Larkin JO, Casey GD, Tangney M, Cashman J, Collins CG, Soden DM, et al. Effective tumor treatment using optimized ultrasound-mediated delivery of bleomycin. Ultrasound Med Biol 2008; 34: 406–413
- Yudina A, Lepetit-Coiffe M, Moonen CT. Evaluation of the temporal window for drug delivery following ultrasound-mediated membrane permeability enhancement. Mol Imaging Biol 2011; 13: 239–249
- Deckers R, Quesson B, Arsaut J, Eimer S, Couillaud F, Moonen CT. Image-guided, noninvasive, spatiotemporal control of gene expression. Proc Natl Acad Sci USA 2009; 106: 1175–1180
- Frenkel V. Ultrasound mediated delivery of drugs and genes to solid tumors. Adv Drug Deliv Rev 2008; 60: 1193–1208
- Tempany CM, McDannold NJ, Hynynen K, Jolesz FA. Focused ultrasound surgery in oncology: Overview and principles. Radiology 2011; 259: 39–56
- Kennedy JE. High-intensity focused ultrasound in the treatment of solid tumours. Nat Rev Cancer 2005; 5: 321–327
- Kim SH, Jung SE, Kim HL, Hahn ST, Park GS, Park WC. The potential role of dynamic MRI in assessing the effectiveness of high-intensity focused ultrasound ablation of breast cancer. Int J Hyperthermia 2010; 26: 594–603
- Illing RO, Kennedy JE, Wu F, ter Haar GR, Protheroe AS, Friend PJ, et al. The safety and feasibility of extracorporeal high-intensity focused ultrasound (HIFU) for the treatment of liver and kidney tumours in a western population. Br J Cancer 2005; 93: 890–895
- Wang Y, Wang W, Wang Y, Tang J. Ultrasound-guided high-intensity focused ultrasound treatment for needle-track seeding of hepatocellular carcinoma: Preliminary results. Int J Hyperthermia 2010; 26: 441–447
- Wang Y, Wang W, Tang J. Ultrasound-guided high intensity focused ultrasound treatment for extra-abdominal desmoid tumours: Preliminary results. Int J Hyperthermia 2011; 27: 648–653
- Huisman M, van den Bosch MA. MR-guided high-intensity focused ultrasound for noninvasive cancer treatment. Cancer Imaging 2011; 11: S161–166
- Zhou YF. High intensity focused ultrasound in clinical tumor ablation. World J Clin Oncol 2011; 2: 8–27
- Chua TC, Thornbury K, Saxena A, Liauw W, Glenn D, Zhao J, et al. Radiofrequency ablation as an adjunct to systemic chemotherapy for colorectal pulmonary metastases. Cancer 2010; 116: 2106–2114
- Patel PR, Luk A, Durrani A, Dromi S, Cuesta J, Angstadt M, et al. In vitro and in vivo evaluations of increased effective beam width for heat deposition using a split focus high intensity ultrasound (HIFU) transducer. Int J Hyperthermia 2008; 24: 537–549
- Frenkel V, Li KC. Potential role of pulsed high intensity focused ultrasound in gene therapy. Future Oncol 2006; 2: 111–119
- Fotopoulou C, Cho CH, Kraetschell R, Gellermann J, Wust P, Lichtenegger W, et al. Regional abdominal hyperthermia combined with systemic chemotherapy for the treatment of patients with ovarian cancer relapse: Results of a pilot study. Int J Hyperthermia 2010; 26: 118–126
- Issels RD, Lindner LH, Verweij J, Wust P, Reichardt P, Schem BC, et al. Neo-adjuvant chemotherapy alone or with regional hyperthermia for localised high-risk soft-tissue sarcoma: A randomised phase 3 multicentre study. Lancet Oncol 2010; 11: 561–570
- Craciunescu OI, Blackwell KL, Jones EL, Macfall JR, Yu D, Vujaskovic Z, et al. DCE-MRI parameters have potential to predict response of locally advanced breast cancer patients to neoadjuvant chemotherapy and hyperthermia: A pilot study. Int J Hyperthermia 2009; 25: 405–415
- Kong G, Dewhirst MW. Hyperthermia and liposomes. Int J Hyperthermia 1999; 15: 345–370
- Song CW. Effect of local hyperthermia on blood flow and microenvironment: A review. Cancer Res 1984; 44: 4721s–4730s
- Horsman MR, Overgaard J. Can mild hyperthermia improve tumour oxygenation?. Int J Hyperthermia 1997; 13: 141–147
- Jain RK. Delivery of molecular medicine to solid tumors. Science 1996; 271: 1079–1080
- Song CW, Park HJ, Lee CK, Griffin R. Implications of increased tumor blood flow and oxygenation caused by mild temperature hyperthermia in tumor treatment. Int J Hyperthermia 2005; 21: 761–767
- Kong G, Braun RD, Dewhirst MW. Hyperthermia enables tumor-specific nanoparticle delivery: Effect of particle size. Cancer Res 2000; 60: 4440–4445
- Lefor AT, Makohon S, Ackerman NB. The effects of hyperthermia on vascular permeability in experimental liver metastasis. J Surg Oncol 1985; 28: 297–300
- Song CW, Kang MS, Rhee JG, Levitt SH. Effect of hyperthermia on vascular function in normal and neoplastic tissues. Ann NY Acad Sci 1980; 335: 35–47
- Gerlowski LE, Jain RK. Effect of hyperthermia on microvascular permeability to macromolecules in normal and tumor tissues. Int J Microcirc Clin Exp 1985; 4: 363–372
- Kong G, Braun RD, Dewhirst MW. Characterization of the effect of hyperthermia on nanoparticle extravasation from tumor vasculature. Cancer Res 2001; 61: 3027–3032
- Liu P, Zhang A, Xu Y, Xu LX. Study of non-uniform nanoparticle liposome extravasation in tumour. Int J Hyperthermia 2005; 21: 259–270
- Nishimura Y, Hiraoka M, Jo S, Akuta K, Yukawa Y, Shibamoto Y, et al. Microangiographic and histologic analysis of the effects of hyperthermia on murine tumor vasculature. Int J Radiat Oncol Biol Phys 1988; 15: 411–420
- Fajardo LF, Schreiber AB, Kelly NI, Hahn GM. Thermal sensitivity of endothelial cells. Radiat Res 1985; 103: 276–285
- Friedl J, Turner E, Alexander HR, Jr. Augmentation of endothelial cell monolayer permeability by hyperthermia but not tumor necrosis factor: Evidence for disruption of vascular integrity via VE-cadherin down-regulation. Int J Oncol 2003; 23: 611–616
- Matthew CB, DuBose DA, Sils II, Tartartini KA. Hyperthermia-induced changes in the vascular permeability of rats: A model system to examine therapeutic interventions. J Therm Biol 2000; 25: 381–386
- de Smet M, Heijman E, Langereis S, Hijnen NM, Grull H. Magnetic resonance imaging of high intensity focused ultrasound mediated drug delivery from temperature-sensitive liposomes: An in vivo proof-of-concept study. J Control Release 2011; 150: 102–110
- Staruch R, Chopra R, Hynynen K. Localised drug release using MRI-controlled focused ultrasound hyperthermia. Int J Hyperthermia 2011; 27: 156–171
- Apfel RE. Acoustic cavitation: A possible consequence of biomedical uses of ultrasound. Br J Cancer Suppl 1982; 5: 140–146
- Wu J, Nyborg WL. Ultrasound, cavitation bubbles and their interaction with cells. Adv Drug Deliv Rev 2008; 60: 1103–1116
- Krasovitski B, Frenkel V, Shoham S, Kimmel E. Intramembrane cavitation as a unifying mechanism for ultrasound-induced bioeffects. Proc Natl Acad Sci USA 2011; 108: 3258–3263
- Kinoshita M, McDannold N, Jolesz FA, Hynynen K. Noninvasive localized delivery of Herceptin to the mouse brain by MRI-guided focused ultrasound-induced blood-brain barrier disruption. Proc Natl Acad Sci USA 2006; 103: 11719–11723
- Kinoshita M, McDannold N, Jolesz FA, Hynynen K. Targeted delivery of antibodies through the blood–brain barrier by MRI-guided focused ultrasound. Biochem Biophys Res Commun 2006; 340: 1085–1090
- Raymond SB, Treat LH, Dewey JD, McDannold NJ, Hynynen K, Bacskai BJ. Ultrasound enhanced delivery of molecular imaging and therapeutic agents in Alzheimer's disease mouse models. PLoS One 2008; 3: e2175
- Yuh EL, Shulman SG, Mehta SA, Xie J, Chen L, Frenkel V, et al. Delivery of systemic chemotherapeutic agent to tumors by using focused ultrasound: Study in a murine model. Radiology 2005; 234: 431–437
- Khaibullina A, Jang BS, Sun H, Le N, Yu S, Frenkel V, et al. Pulsed high-intensity focused ultrasound enhances uptake of radiolabeled monoclonal antibody to human epidermoid tumor in nude mice. J Nucl Med 2008; 49: 295–302
- Huber PE, Mann MJ, Melo LG, Ehsan A, Kong D, Zhang L, et al. Focused ultrasound (HIFU) induces localized enhancement of reporter gene expression in rabbit carotid artery. Gene Ther 2003; 10: 1600–1607
- Mehier-Humbert S, Yan F, Frinking P, Schneider M, Guy RH, Bettinger T. Ultrasound-mediated gene delivery: Influence of contrast agent on transfection. Bioconjug Chem 2007; 18: 652–662
- van Wamel A, Kooiman K, Harteveld M, Emmer M, ten Cate FJ, Versluis M, et al. Vibrating microbubbles poking individual cells: Drug transfer into cells via sonoporation. J Control Release 2006; 112: 149–155
- Schlicher RK, Radhakrishna H, Tolentino TP, Apkarian RP, Zarnitsyn V, Prausnitz MR. Mechanism of intracellular delivery by acoustic cavitation. Ultrasound Med Biol 2006; 32: 915–924
- Lionetti V, Fittipaldi A, Agostini S, Giacca M, Recchia FA, Picano E. Enhanced caveolae-mediated endocytosis by diagnostic ultrasound in vitro. Ultrasound Med Biol 2009; 35: 136–143
- Meijering BD, Juffermans LJ, van Wamel A, Henning RH, Zuhorn IS, Emmer M, et al. Ultrasound and microbubble-targeted delivery of macromolecules is regulated by induction of endocytosis and pore formation. Circ Res 2009; 104: 679–687
- Cruciani G, Molinari AL, Marangolo M, Morelli M, Gnani G, Mazzotti A, et al. Applicability of local hyperthermia as adjuvant to systemic chemotherapy. Tumori 1987; 73: 629–633
- Issels RD, Lindner LH, Verweij J, Wust P, Reichardt P, Schem BC, et al. Neo-adjuvant chemotherapy alone or with regional hyperthermia for localised high-risk soft-tissue sarcoma: A randomised phase 3 multicentre study. Lancet Oncol 2010; 11: 561–570
- Mace TA, Zhong L, Kokolus KM, Repasky EA. Effector CD8+ T cell IFN-gamma production and cytotoxicity are enhanced by mild hyperthermia. Int J Hyperthermia 2012; 28: 9–18
- Miller DL, Song J. Tumor growth reduction and DNA transfer by cavitation-enhanced high-intensity focused ultrasound in vivo. Ultrasound Med Biol 2003; 29: 887–893
- Tschoep-Lechner K, Drexler I, Hammer D, Neumann D, Pohla H, Sutter G, et al. Modified vaccinia virus Ankara delivers a robust surrogate marker for immune monitoring to sarcoma cells even if cells are being exposed to chemotherapy and heat treatment. Int J Hyperthermia 2012; 28: 33–42
- Pathak A, Patnaik S, Gupta KC. Recent trends in non-viral vector-mediated gene delivery. Biotechnol J 2009; 4: 1559–1572
- Mir LM, Tounekti O, Orlowski S. Bleomycin: Revival of an old drug. Gen Pharmacol 1996; 27: 745–748
- Lindenberg M, Kopp S, Dressman JB. Classification of orally administered drugs on the World Health Organization Model list of Essential Medicines according to the biopharmaceutics classification system. Eur J Pharm Biopharm 2004; 58: 265–278
- Yudina A, de Smet M, Lepetit-Coiffe M, Langereis S, Van Ruijssevelt L, Smirnov P, et al. Ultrasound-mediated intracellular drug delivery using microbubbles and temperature-sensitive liposomes. J Control Release 2011; 155: 442–448
- Unger EC, Hersh E, Vannan M, Matsunaga TO, McCreery T. Local drug and gene delivery through microbubbles. Prog Cardiovasc Dis 2001; 44: 45–54
- O'Neill BE, Vo H, Angstadt M, Li KP, Quinn T, Frenkel V. Pulsed high intensity focused ultrasound mediated nanoparticle delivery: Mechanisms and efficacy in murine muscle. Ultrasound Med Biol 2009; 35: 416–424