Abstract
We developed a novel hyperthermia material for cancer therapy, cationic albumin-conjugated magnetite nanoparticles (MNPs), which absorb the energy of an alternating magnetic field and convert it into heat. MNPs of about 10 nm were synthesised through co-precipitation, and citric acid was used to stabilise the MNP suspension. Then albumin was cationised by replacing anionic side chain groups with cationic groups. The surface modification of the MNPs was provided by cationic albumin, which was covalently conjugated to carboxylic acid functions located at the distal end of the MNPs’ surface by carbodiimide chemistry. Finally, we obtained stable superparamagnetic suspensions with particle sizes of 140 nm and saturation magnetisation of 67 emu/g, which do not have the disadvantage of eventual desorption of physical attachment. We also analysed the potential of these particles for magnetic fluid hyperthermia by determination of the specific absorption rate at a constant frequency of 215 kHz; the temperature increase of the particles was 30.8 °C. This study experimentally demonstrates the high efficiency of these nano-heaters.
Introduction
The heating of certain tissues to temperatures between 41–46 °C especially for cancer therapy is called hyperthermia. Tumour cells are more sensitive to a temperature increase than healthy ones due to their higher rates of metabolism, which makes hyperthermia a very promising cancer treatment [Citation1]. Cancer cells are destroyed at temperatures higher than 43 °C, while the normal cells could survive at those temperatures [Citation2].
The use of hyperthermia in treatment of malignant tumours is as old as medicine itself. Hippocrates, the father of medicine, proposed that surface tumours should be cauterised by application of hot iron [Citation3]. In modern times, more advanced methods (hot water bath, high-frequency radiation, radiotherapy and magnetic nano-fluid hyperthermia) are employed to heat, and hopefully destroy tumours [Citation4].
Nano-thermotherapy has brought a great attention for researchers to kill the tumour cells, not with chemotherapeutic agents, but with locally targeted nano-heaters, having fewer side effects than chemotherapy and radiotherapy [Citation5]. Besides, this method provides a straightforward way of treating cancer in combination with chemotherapy and irradiation.
MNPs have been examined as potential hyperthermia agents due to their high specific absorption rate (SAR), described as the rate at which magnetic field energy is absorbed by a unit mass of a material. Experimental investigations of the magnetic materials for hyperthermia application date back to 1957 when Gilchrist et al. [Citation6] heated different tissue samples with 20–100 nm particles of γ-Fe2O3 exposed to a 1.2 MHz magnetic field.
For biomedical applications the surface of MNPs is often treated physically or chemically with biocompatible polymers such as albumin, chitosan, dextran and polyethylene glycol. Not only the surface modification could increase the chemical stability of the particles, but also it could improve the biocompatibility of the MNPs. A polymer shell can act as compatibiliser and be supplied with biologically functional sites for drug targeting [Citation7].
Between different polymers, albumin has attracted great attention because of its preferential accumulation in solid tumours and inflamed tissues, ready availability, biodegradeability, lack of inherent toxicity, immunogenicity and ability to bind to various ligands. All of these characteristics make it an ideal candidate for biomedical applications. Albumin is one of the smallest and the most abundant proteins present in blood plasma. Albumins have many physiological functions and play a key role in the transport of many endogenous and exogenous ligands [Citation8]. The enhanced uptake of albumin-based systems in solid tumours is mediated by the pathophysiology of tumour tissue, characterised by angiogenesis, hypervasculature, a defective vascular architecture and an impaired lymphatic drainage. In addition, it was shown that the accumulation is also due to transcytosis initiated by binding of albumin to over-expressed albumin-binding proteins (gp60 and SPARC) in advanced tumours [Citation9].
The extent of the chemical attachment between albumin and particles strongly depends on their surface groups and charges. Albumin is an acidic protein that is extremely robust towards pH. It is stable in the pH range of 4–9. Under physiological conditions albumin has a negative charge and so cannot react electrostatically with MNPs. However, the charge of the albumin can be modified by converting anionic side chain carboxylic groups with ethylenediamine [Citation10,Citation11], thereby producing a highly cationic albumin. Cationised albumin produced by this simple and highly reproducible technique [Citation12] demonstrated good biocompatibility and low cytotoxicity in vitro and in vivo, as well as enzymatic biodegradability [Citation13–15]. Besides, cationised albumin contains many primary amino groups which can be activated for conjugation with lipids and ligands such as antibodies [Citation16]. Modification of albumin can induce cell-specific targeting [Citation17,Citation18] using insulin bound cationised albumin for targeted gene transfer. Moreover, cationic albumin is a component which is in direct contact with the surrounding environment; it interacts electrostatically with the negative charge on glycocalyx, situated on the surface of KB cells [Citation19].
In the present study a new nanostructure magnetic hyperthermia material, cationic albumin chemically attached citrate-capped MNPs, was synthesised. Characterisation of physical and chemical properties of these particles along with process parameters were studied to design the best material for hyperthermia treatment.
Magnetic hyperthermia
To induce hyperthermia, MNPs can absorb energy throughout the application of an alternating magnetic field and spread out it in the form of heat to the surrounding medium. The hyperthermia method relies on the Maxwell’s theory that current is induced when any metallic or metal oxide particles are placed in an AC magnetic field. The amount of current is proportional to the size of the magnetic field and the particles. As these currents flow within the particles, heat is produced due to the electrical resistance of the particles. Metals with higher magnetic property, such as cobalt and iron, show higher inductive heating. Consequently, when MNPs are exposed to alternative magnetic fields, the particles become powerful heat sources to destroy the targeted tumour cells [Citation20].
It is well known that the heating of magnetic particles in an AC magnetic field is mainly due to four energy loss processes [Citation21–24]: eddy current, resonance, hysteresis, and relaxation. Usually the contribution of the eddy current is neglected for small particles (less than 100 nm in diameter) if the frequency of the alternating field is also less than 10 GHz [Citation25]. The resonance loss can also be neglected when the applied magnetic field is of moderate frequency [Citation21]. For magnetic materials the hysteresis loss can be estimated from the area integration of the hysteresis loop by considering the proportionality of power with frequency [Citation21,Citation22]. When the frequency is increased, the hysteresis loop increases in width which is called dynamic hysteresis [Citation26].
In the case of relaxation loss, two principal mechanisms have been identified for MNP relaxation: Brownian and Neel. Heat dissipation from the small magnetic particles is caused by the delay in the relaxation of the magnetic moment through either the rotation within the particle (Neel) or the rotation of the particle itself (Brownian, viscous loss). When the particles are exposed to an AC magnetic field with the time of magnetic reversals less than the magnetic relaxation times of particles, the heat is dissipated due to the delay in the relaxation of the magnetic moment [Citation27]. Dominant relaxation loss mechanisms differ with particle size. If the average particle size is less than critical superparamagnetic diameter (25 nm) the Brownian mechanism is prevailing.
Heating capacity of MNPs can be affected by many factors. For example, Hergt et al. [Citation28] observed that the heating effect of magnetic particles strongly depended on the particle size. It was also found that the size distribution width of nanoparticles has a crucial influence on the SAR and can be raised greatly through a size selection process. High saturation magnetisation was shown to achieve higher temperatures under the same heating conditions [Citation29]. In addition, the SAR values of some MNPs increased with increasing frequency and amplitude of the alternating magnetic field [Citation30,Citation31]. Hergt [Citation28] reported that the SAR increased with increasing initial susceptibility. Also, the viscosity of the sample was shown to reduce the heating contribution from the Brownian mechanism [Citation30]. The SAR value was unaffected by the protein and blood sugar environments, but was significantly reduced in the electrolyte environment due to precipitation and aggregation with sodium ions [Citation32]. In addition, Chan et al. [Citation33] discovered that the preparation method strongly influenced the SAR, too, and it could be raised with an ultrasonic treatment.
Materials and methods
Materials
Ferric chloride hexahydrate, ferrous chloride tetrahydrate, citric acid (CA), ammonia solution (25%) and ethylenediamine (EDA) were obtained from Merck (Darmstadt, Germany). 1-ethyl-3-(3 dimethylaminopropyl) carbodiimide.HCl (EDC), N-hydroxysuccinimide (NHS), 2-(N-morpholino)ethanesulfonic acid (MES), bovine serum albumin (BSA) were supplied by Sigma (St. Louis, MO). All chemicals were of analytical grade and used as received.
Preparation and characterisation of magnetite nanoparticle-capped citric acid
Magnetite (Fe3O4) nanoparticles were prepared by co-precipitation of FeCl2.4H2O and FeCl3.6H2O (1:2 molar ratio) by the addition of NH4OH [Citation34]. In a typical synthesis, 3.68 g of FeCl3.6H2O and 1.35 g of FeCl2.4H2O were dissolved in 40 mL of deionised water in a three-necked flask and temperature was slowly increased to 80 °C in refluxing conditions under nitrogen atmosphere. The temperature was maintained at 80 °C for 30 min. While vigorously stirring the reaction mixture, turbulence was created by placing the reaction flask in an ultrasonic bath and controlling the homogenisation (overhead stirrer) rate (9,000 rpm) during the initial 2 min of the reaction. An aliquot of 20 mL of ammonia solution was added instantaneously to the reaction mixture, the resulting black suspension being further stirred for 30 min. The surface of MNPs must be modified to stabilise the nanoparticles and also tomake functional groups on the surface of the nanoparticles for the next binding step. In this way the MNP surfaces were treated with citrate ions by incubating for 1 h within 2 mL of 0.5 g/mL citric acid solution and reaction temperature was raised up to 90 °C. The reaction was completed for 60 min with continuous stirring. The black precipitates were acquired by cooling the reaction mixture to room temperature. Lastly, the suspensions were washed several times with deionised water. The reaction product as formed contained an excess of citric acid, and so the nanoparticle dispersion was centrifuged at 10,000 rpm for 5 min to precipitate the nanoparticles, then washed several times and finally freeze dried. Magnetite nanoparticles stabilised with citric acid are abbreviated as MNP-CA in this text.
Structural characterisation of MNP-CA
The synthesised nanoparticles were characterised by various analytical techniques. The X-ray diffraction (XRD) patterns were acquired from freezedried nanoparticle samples with a Bruker (Karlsruhe, Germany) X-ray powder diffractometer using CuKα1 (1.54060 Å) radiation. The scans of the selected diffraction peaks were carried out in the step mode (step size 0.02°, measurement time 2 s, measurement temperature 300 °K).
Transmission electron microscopy (TEM) images were acquired on a Phillips 400 TEM operating at 100 kV. TEM grids were prepared by depositing a drop of the diluted nanoparticle suspension on 300-mesh silicon-monoxide support films and drying the grids under vacuum for 2 h.
The infrared spectra were recorded in the range of 400–4,000 cm−1 on a Fourier transform infrared spectrometer (FTIR-Shimadzu-8000). Samples of the surface-modified nanoparticles were dried overnight using a VirTis Freeze mobile freeze dryer (SP Industries, Warminster, PA), Nanoparticle powder (2 mg) was then milled with potassium bromide and the mixture was pressed into a pellet for analysis.
Preparation of cationic albumin
The preparation of cationic albumin followed the method of Muckerheide et al. [Citation35] with some modifications. Briefly, 1 mL of ethylenediamine (EDA) was added to 1 mL of MES as conjugation buffer (pH 4.75) in an ice bath, the pH value was readjusted to 4.75 with 5 M HCl. Then the mixture was slowly added to 1 mL of the same buffer containing 100 mg of BSA and 60 mg of EDC, and incubated at room temperature for 2 h with magnetic stirring. The reaction was terminated using acetic acid and dialysed comprehensively against distilled water for 72 h, using a dialysis membrane (cut-off = 12 kDa, Sigma-Aldrich), with water replacement at least three times. The final volume reached was 20 mL.
The molecular mass and the isoelectric point of BSA and cationic BSA were determined by sodium dodecyl sulphate polyacrylamide gel electrophoresis (SDS-PAGE) and isoelectric focusing (IEF) using Biorad (Champaign, IL) apparatus and according to the company protocol. Native and cationised albumins were subjected to SDS-PAGE analysis (figure not shown), and all were found to give one band. The molecular weight did not change in comparison with that of albumin, approximately 67 kDa according to their mobility in SDS-PAGE. Low molecular weight fragmentation and high molecular weight aggregation was not detected.
Covalent attachment of cationic albumin and MNP-CA
Cationic albumin was grafted onto the MNP-CA surface by carbodiimide activation forming a chemical bond between carboxylic (−COOH) groups present in the MNP-CA structure and amine (−NH2) end groups from the cationic albumin molecules.
Surface modification of MNP-CA with cationic albumin was carried out in aqueous media in neutral conditions using carbodiimide chemistry [Citation36]. The carboxylic groups of MNP-CA were activated using EDC and subsequently the activated esters formed their linkages with the amine groups previously grafted onto albumin. We used NHS as an additive to decrease the formation of byproducts and bias the formation of bonds between amine and carboxylic groups [Citation37]. Briefly, the prepared MNP-CA (10 mg) was dispersed in 2 mL of phosphate buffered solution at pH 8. Then 300 µL of cationic albumin solution was added, followed by addition of 10 mg EDC and 6 mg NHS. The pH of this solution was adjusted to 8 using phosphate buffer. The mixture was stirred at room temperature for 2 h. Afterwards, free cationic albumin molecules were removed by ultra-filtration with centrifugal device (Millipore, Court Vernon Hills, IL, cut-off =100 kDa) and washed several times with deionised water containing 10% NBu3 (3-Butylamine) to remove amine-carboxylic acid salt bridges. The bio-conjugation yield was determined by Bradford microassay from the recovered cationic BSA calculated from the calibration curve.
Structural characterisation of cationic albumin-conjugated MNP-CA
The morphology of cationic albumin-conjugated MNP-CA was investigated by scanning electron microscope (SEM) (Oxford S360).
Hydrodynamic diameter and zeta potential were measured using a Malvern Instruments (3000-HS, Malvern, UK) particle size and zeta potential analyser.
Magnetic properties
The saturation magnetisation (Ms) and coercive force (Hc) of the freeze-dried samples were measured using a vibrating sample magnetometer (VSM) (250, Dexing, Xiamen, China) with a sensitivity of 10−3 emu. The magnetic field was changed uniformly with a time rate of 66 Oe/s.
Heating properties
To determine the SAR of the nanoparticles we have prepared samples of the synthesised MNP-CA and cationic albumin-conjugated MNP-CA suspended in distilled water (deoxygenated by bubbling N2 gas for 60 min) with a magnetic composition of 1% w/v (g of Fe3O4/mL solution). These samples were placed in a magnetic field with a constant frequency of 215 kHz and magnetic field amplitude of 3 kAm−1. The temperature of the surrounding medium was kept constant using a heated chamber at 25 °C and the temperature was measured by infrared (IR) thermal camera under an alternating magnetic field.
Results and discussion
A schematic illustration for the formation of the cationic albumin-conjugated MNP-CA is shown in . MNPs were synthesised and then stabilised by citric acid (). Then albumin was cationised () and cationic albumin chains were conjugated to MNP-CA using carbodiimide amidation chemistry ().
Figure 1. Scheme for the functionalisation procedure of magnetite nanoparticles (MNP) described in this work. (A) Preparation of stabilised magnetite nanoparticle by citric acid (CA). (B) Preparation of cationised albumin by modification of the carboxylate groups with an ethylenediamine. (C) Surface modification of MNP-CA by cationic albumin.
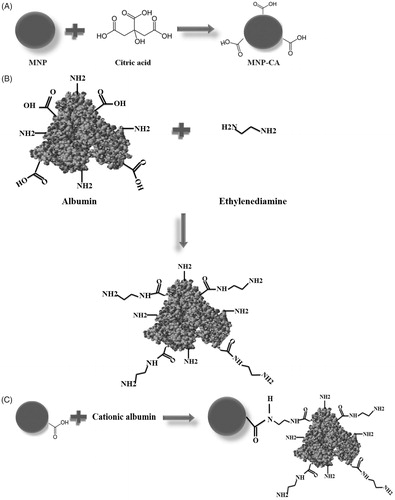
XRD analysis
shows the single-phase spinel structure of the MNPs which was confirmed by the XRD pattern. The XRD pattern of the nanoparticles matches the pattern for the magnetite listed in ASTM XRD standard card (19-0629), with expected inverse spinel structure and without any trace of impurity peaks. The XRD peaks can be indexed into the spinel cubic lattice type with a lattice parameter of 8.370 Å which is very close to the reported value of magnetite [Citation38]. The attendance of intense and sharp peaks substantiated the formation of highly crystalline nanoparticles [Citation39].
The comparatively broad reflections reveal the nanosize of the crystals. Applying the Rietveld refinement of XRD pattern [Citation40], the average grain size from the broadening of the XRD peaks of MNPs was calculated. The crystallite size was 12 nm and the Rietveld refinement parameters (Rwp = 7.78 and Rp = 5.91) were obtained.
TEM analysis
The size and morphology of the lyophilised MNP-CA without any size selection are shown in . The TEM result shows small and uniform rounded nanoparticles. The average size was about 10 nm.
FTIR analysis
shows the FTIR spectra of pure citric acid (CA) and magnetite nanoparticles stabilised with citric acid (MNP-CA), the absorption bands for the pure CA are resolved, but those of the MNP-CA are broad and few. In , an intense band at 3,300 cm–1 proposes the presence of non-dissociated OH groups of citric acid. The peak around 2,940 cm−1 is due to CH2 stretching; peak at 1,618 cm–1 may be assigned to the symmetric stretching of OH from COOH group, displaying the binding of a citric acid radical to the magnetite surface. The 1,755 cm−1 peak of CA, attributable to the C = O vibration from the COOH group of CA, this peak shifts to an intense band at about 1,618 cm−1 for MNP-CA displaying the binding of a CA radical to the surface of MNPs by chemisorptions of carboxylate (citrate) ions [Citation17,Citation37]. Carboxylate groups of CA form complexes with Fe atoms on the surface of the MNPs representing partial single bond character to the C = O bond. The next strong FTIR band around 575 cm−1 can be attributed to the FeO stretching vibration mode of Fe3O4 [Citation38]. Therefore, we could say that the citric acid binds to the magnetite surface by carboxylate.
Figure 4. (A) FTIR of citric acid and citrate-capped iron oxide nanoparticles. (B) FTIR of albumin and cationised albumin.
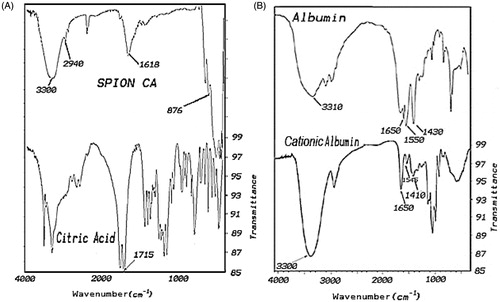
exhibits the FTIR spectra of albumin and cationised albumin. The strong band at 1,650 cm−1 was attributed to amide bond in both albumin and cationised albumin sample, but with different intensity.
The strong band at 1,410 cm–1 was associated to the C = O vibration from the COOH group in albumin but the cationised albumin had a weak band at this wave number, indicating the decrease of carboxyl group in cationised albumin.
A broad strong band at 3,380 cm–1 was attributable to the NH vibration from the NH2 group in cationised albumin. In the FTIR spectra of albumin, a similar band was observed compared with cationised albumin, but the albumin had a weak band at this wave number, indicating the increase of amine group in cationised albumin.
Vibrating sample magnetometer analysis
shows VSM plot of MNP-CA and cationic albumin-conjugated MNP-CA at 300 °K. It can be seen that no coercivity or remanence could be observed for samples, suggesting the superparamagnetic properties of the particles. This can be ascribed to the small size of particles, which were smaller than the superparamagnetic critical size (25 nm) [Citation41]. The Ms of the MNP-CA was 74 emu/g (g of solid particle). The high saturation magnetisation indicated good crystal structure. The saturation magnetisation of the cationic albuminconjugated MNP-CA was 67 emu/g which was slightly lower than that of MNP-CA. It was due to the existence of the large amount of diamagnetic albumin in the particles. This high magnetic response makes these new nanoparticles more effective compared with similar polymer-coated MNP systems which can be used for hyperthermia treatment. For biomedical applications such as hyperthermia, it is required that the MNPs have high saturation magnetisation, uniform particle size and superparamagnetism.
Zeta potential analysis
shows zeta potential of MNP (A), MNP-CA (B), cationic albumin () and cationic albuminconjugated MNP-CA (). As can be seen, the zeta potential increases from −4.8 mV for MNP solution to −16.3 mV for MNP-CA. This high negative value of zeta potential for the MNP-CA could be attributed to the adsorption of citric acid onto the surface of MNPs and thus presence of free (−COOH) groups remaining in the MNP-CA surface.
Figure 6. Zeta potential measurements of MNP (A), MNP-CA (B), cationic albumin (C), and cationic albumin-conjugated MNP-CA (D).
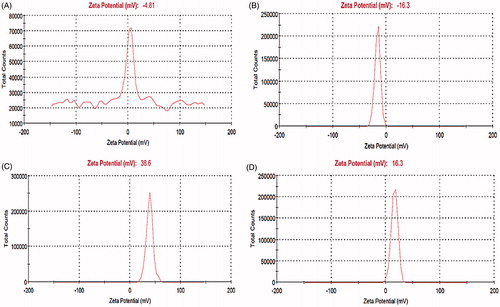
The high positive zeta potential of about 38.6 mV for cationic albumin can be attributed to the substituting anionic side chain carboxyl groups with amine groups. In the case of cationic albumin-conjugated MNP-CA, we observed a transition in the zeta potential measurement from −16.3 mV to 16.3 mV due to the presence of (−NH2) free groups remaining onto the cationic albumin-conjugated MNP-CA.
SEM analysis and particle size analysis
shows the SEM image of the prepared cationic albumin-conjugated MNP-CA. As is shown, particles are spherical in shape with a good dispersity. Also, particle sizes are measured by particle size analyser and zetasiser. These measurements were done without any size selection. As can be seen, particle sizes from all these analyses resulted in almost the same values. The average size was approximately 147 nm.
Bradford assay of degree of MNP-CA functionalised with cationic albumin
Degree of MNP-CA modification with cationic albumin was determined by the percentages of cationic albumin recovery on MNP-CA by Bradford assay with MNP-CA as reference particles. We performed the microassay protocol (300 µL microplate assay) and used the typical standard curve of BSA. The degree of modification showed 28% cationic albumin-conjugated MNP-CA.
Specific absorption rate (SAR) analysis
Our formulations were tested for these heating effects and results are presented in . The temperature of formulations is plotted as a function of time at a constant frequency of 215 kHz. To obtain an accurate SAR value an adiabatic environment is assumed, where the initial temperature of the sample must be equal to the temperature of the surrounding medium. In our experimental set-up, a sample of the functionalised MNP-CA was placed in an isolated environment. The temperature of the surrounding medium was kept constant using a heated chamber at 25 °C.
Figure 8. Temperature as a function of time for MNP-CA and cationic albumin-conjugated MNP-CA suspended in distilled water under alternating magnetic field of 215 kHz.
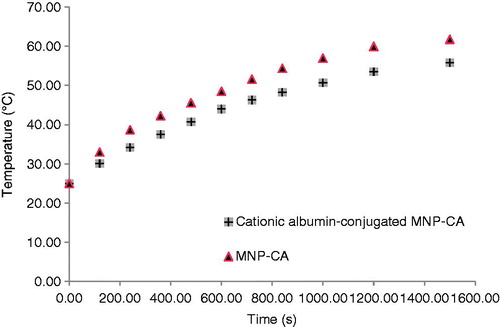
The main parameter determining the heating of the particles is the SAR, which is expressed in W/kg. The SAR formula for the adiabatic case [Citation42] is
where C, is the specific heat of solvent (C = Cwater = 4.18 J/g °C), ΔT/Δt is the initial slope of the time-dependent temperature curve and mFe is mass fraction of Fe in the sample.
We used Equation 1 to calculate the SAR of the prepared magnetic samples assuming the heat capacity of these diluted samples as the heat capacity of water (4.18 J/g °C). For MNP-CAs and cationic albumin-conjugated MNP-CAs we have calculated SAR of about 16.72 W/g and 10.66 W/g, respectively.
As shown in , the highest temperature change of 36 °C was measured in MNP-CA suspension. The cationic albuminconjugated MNP-CA suspension had lower value temperature change of 30.8 °C. These nanoparticles had remarkable heating effect which has great potential in hyperthermia.
As was expected, smaller clustered particles generate higher heat because of their higher specific surface area. Also, the higher Ms of smaller particles (MNP-CA) was another reason to show higher SAR.
Conclusions
Advancements in our ability to fabricate nanoparticles with great control over physical and chemical properties have led to new nanoparticle candidates for hyperthermia application. In this project nanoparticles were fabricated cautiously considering design parameters. A simple procedure was described for the preparation of citric acid-stabilised MNPs, averagely sized about 10 nm with a Ms of 74 emu/g. Also, a convenient route was defined for covalently bonding cationic albumin onto the citrate-capped MNPs, using carbodiimide chemistry. This surface modification is carried out between carboxylic groups (−COOH) previously introduced in the MNP-CA surface and amine groups (−NH2) grafted onto the cationic albumin. Synthesised cationic albumin-conjugated MNP-CA showed a spherical shape and good dispersity. Due to the small size of the cationic albumin-conjugated MNP-CA (147 nm) and their superparamagnetic behaviour (Ms = 67 emu/g), these particles were able to dissipate energy at a rate of 10.66 W/g during the application of a magnetic field (215 kHz). These nanoparticles also show good colloidal stability upon mixing with phosphate-buffered saline and serum containing culture medium. Therefore, we consider these nanoparticles’ formulation would be highly useful for localised hyperthermia treatment of cancers.
Declaration of interest
The authors report no conflicts of interest. The authors alone are responsible for the content and writing of the paper.
Acknowledgements
The authors wish to thank the staff of the Department of Pharmaceutics, Shiraz University of Medical Sciences, Iran.
References
- Tran N, Webster TJ. Magnetic nanoparticles: Biomedical applications and challenges. J Mater Chem 2010;20:8760–7
- Bulte JWM, Brooks RA. Magnetic particles as contrast agents for MR imaging. In: Häfeli U, Schütt W, Teller J, Zborowski M, editors. Scientific and Clinical Applications of Magnetic Carriers. New York: Plenum; 1997. pp 527–43
- Hofer KG. Hyperthermia and cancer. Eur Cells Mater 2002;3:S67–69
- Nielsen OS, Horsman M, Overgaard J. A future for hyperthermia in cancer treatment. Eur J Cancer 2001;37:1587–9
- Gazeau F, Levy M, Wilhelm C. Optimizing magnetic nanoparticle design for nanothermotherapy. Nanomedicine 2008;3:831–44
- Gilchrist RK, Shorey WD, Hanselman RC, Parrott JC, Taylor CB. Selective inductive heating of lymph. Ann Surg 1957;146:596–606
- Liu TY, Hu SH, Liu KH, Liu DM, Chen SY. Study on controlled drug permeation of magnetic-sensitive ferrogels: Effect of Fe3O4 and PVA. J Control Release 2008;126:228–36
- Klajnerta B, Klajnerta L, Stanislawska M, Bryszewska B. Interactions between PAMAM dendrimers and bovine serum albumin. Biochim Biophys Acta 2003;1648:115–26
- Desai N, Trieu V, Damascelli B, Soon-Shiong P. SPARC expression correlates with response to nab-paclitaxel in head and neck patients. Transl Oncol 2009;2:59–64
- Hoare DG, Koshland DE. Method for the quantitative, modification and estimation of carboxylic acid groups in proteins. J Biol Chem 1967;10:2447–53
- Kumagai AK, Eisenberg JB, Pardridge WM. Absorptive-mediated endocytosis of cationized albumin and a β-endorphin-cationized albumin chimeric peptide by isolated brain capillaries. J Biol Chem 1987;262:15214–9
- Bass PS, Drake AF, Wang Y, Thomas JH, Davies DR. Cationization of bovine serum albumin alters its conformation as well as its charge. Lab Invest 1990;62:185–8
- Pardridge WM, Triguero D, Buciak JL, Yang J. Evaluation of cationized rat albumin as a potential blood–brain barrier drug transport vector. J Pharmacol Exp Ther 1990;255:893–9
- Choksakulnimitr S, Masuda S, Tokuda H, Takakura Y, Hashida M. In vitro cytotoxicity of macromolecules in different cell culture systems. J Contr Rel 1995;34:233–41
- Fischer D, Zange R, Kissel T. Comparative in vitro cytotoxicity studies of polycations for gene therapy. Proc Intern Symp Control Rel Bioact Mater 1997;24:647–8
- Ouellette AD, Ruan KH, Tsai AL, Wu KK, Mikos AG. A poly(L-lysine)/anti-thrombomodulin conjugate for targeted gene delivery to endothelial cells. Proc Intern Symp Control Rel Bioact Mater 1996;23:889–90
- Miller N, Vile R. Targeted vectors for gene therapy. FASEB J 1995;9:190–9
- Huckett B, Ariatti M, Hawtrey AO. Evidence for targeted gene transfer by receptor-mediated endocytosis stable expression following insulin-directed entry of NEO into HepG2 cells. Biochem Pharmacol 1990;40:253–63
- Kim D-H, Kim K-N, Kim K-M, Lee Y-K. Targeting to carcinoma cells with chitosan- and starch-coated magnetic nanoparticles for magnetic hyperthermia. J Biomed Mater Res A 2008;88A:1–11
- Babincova M, Sourivong P, Leszczynska D, Babinec P. Blood-specific whole-body electromagnetic hyperthermia. Med Hypoth 2000;55:459–60
- Li-Ying Z, Hong-Chen G, Xu-Man W. Magnetite ferrofluid with high specific absorption rate for application in hyperthermia. J Magn Magn Mater 2007;311:228–33
- Hergt R, Dutz S, Muller R, Zeisberger M. Magnetic particle hyperthermia: Nanoparticle magnetism and materials development for cancer therapy. J Phys Condens Matter 2006;18:2919–34
- Lao L, Ramanujan V. Magnetic and hydrogel composite materials for hyperthermia applications. J Mater Sci Mater Med 2004;15:1061–4
- Pavel M, Gradinariu G, Stancu A. Study of the optimum dose of ferromagnetic nanoparticles suitable for cancer therapy using MFH. IEEE Trans Magn 2008;44:3205–8
- Ramprasad R, Zurcher P, Petras M, Miller M, Renaud P. Magnetic properties of metallic ferromagnetic nanoparticle composites. J Appl Phys 2004;96:519--29
- Ribbenfjärd D. A lumped element transformer model including core losses and winding impedances. Licentiate thesis in electromagnetic engineering, Stockholm, Sweden, 2007
- Suto M, Hirota Y, Mamiya H, Fujita A, Kasuya R, Tohji K, et al. Heat dissipation mechanism of magnetite nanoparticles in magnetic fluid hyperthermia. J Magn Magn Mater 2009;321:1493–6
- Hergt R, Hiergeist R, Zeisberger M, Glöckl G, Weitschies W, Ramirez LP, et al. Enhancement of AC-losses of magnetic nanoparticles for heating applications. Magn Magn Mater 2004;280:358–68
- Zhao DL, Zeng XW, Xia QS, Tang JT. Preparation and coercivity and saturation magnetization dependence of inductive heating property of Fe3O4 nanoparticles in an alternating current magnetic field for localized hyperthermia. J Alloys Compd 2009;469:215–8
- Fortin JP, Wilhelm C, Servais J, Enager CM, Bacri JC, Gazeau F. Size-sorted anionic iron oxide nanomagnets as colloidal mediators for magnetic hyperthermia. J Am Chem Soc 2007;129:2628–35
- Jordan A, Wust P, Faehling H, Krause J, John W, Hinz A, Felix R. Inductive heating of ferrimagnetic particles and magnetic fluids: Physical evaluation of their potential for hyperthermia. Int J Hyperthermia 1993;9:51–68
- Chen S, Chiang C, Hsieh S. Simulating physiological conditions to evaluate nanoparticles for magnetic fluid hyperthermia (MFH) therapy applications. J Magn Magn Mater 2010;322:247–52
- Chan DC, Kirpotin DB, Bunn PA. Scientific and Clinical Applications of Magnetic Carriers. New York: Plenum Press; 1997. pp 607–18
- Cheraghipour E, Tamaddon AM, Javadpour S, Bruce IJ. PEG conjugated citrate-capped magnetite nanoparticles for biomedical applications. J Magn Magn Mater 2013;328:91–9
- Muckerheide A, Apple RJ, Pesce AJ, Michael JG. Cationization of protein antigens. I. Alteration of immunogenic properties. J Immunol 1987;138:833–7
- Sehgal D, Vijay I. A method for the high efficiency of water-soluble carbodiimide mediated amidation. Anal Biochem 1994;218:87–91
- Kynclova E, Elsner E, Kopf A, Hawa G, Schalkhammer T, Pittner F. Novel method for coupling of poly(ethyleneglycol) to carboxylic acid moieties of proteins. J Mol Recognit 1996;9:644–51
- Levy L, Sahoo Y, Kim K, Bergey EJ, Prasad PN. Nanochemistry: Synthesis and characterization of multifunctional nanoclinics for biological applications. Chem Mater 2002;14:3715–21
- Nigam S, Barick KC, Bahadur D. Development of citrate-stabilized Fe3O4 nanoparticles: Conjugation and release of doxorubicin for therapeutic applications. J Magn Magn Mater 2011;323:237–43
- Rietveld H. A profile refinement method for nuclear and magnetic structures. J Appl Cryst 1969;2:65–7
- Pradhan P, Giri J, Samanta G, Sarma HD, Mishra K, Bellare JR, et al. Comparative evaluation of heating ability and biocompatibility of different ferrite based magnetic fluids for hyperthermia application. J Biomed Mater Res B 2007;81:12–22
- Giri J, Pradhan P, Sriharsha T, Bahadur D. Preparation and investigation of potentiality of different soft ferrites for hyperthermia applications. J Appl Phys 2005;97:910–6