Abstract
Purpose: Irreversible electroporation (IRE) is a new tumour ablation method used in cancer treatment procedures. In a successful IRE treatment it is crucial to impose minimum thermal damage to the tumour and its surrounding healthy tissue, while subjecting the entire tumour to a strong electric field. Method: Here we present a 3D model of a subcutaneous tumour in a four-layer skin using a geometry-based finite element approach. Four common needle electrode configurations were studied in this paper. The study evaluated six essential factors which are important in the electrical and thermal distributions in tumour and normal tissue. Results: The results revealed that a hexagonal 3 × 3 geometry provides the maximum electrical coverage of the tumour, compared to other electrode configurations. However, in some cases the hexagonal 2 × 2 geometry can ablate the entire tumour with less damage to normal tissue. We found that the deeper insertion of 2- and 4-electrode geometries can lead to more damage to healthy tissue. The results also indicate that the insertion of the electrodes into tumour tissue can increase thermal damage dramatically due to existing large electrical conductivity. Conclusion: These findings suggest that needle electrodes should not be placed within the tumour tissue if the goal is to prevent thermal damage. This method can be used as a trade-off between electric field coverage in tumour tissue and thermal damage to both tumour and normal tissue.
Introduction
Electroporation is a technique in which the cell membrane permeability to macromolecules is increased by exposing the cell to short interval high electric field pulses [Citation1,Citation2]. In general, electroporation can be categorised into reversible or irreversible electroporation in terms of electric field strength [Citation3,Citation4]. Reversible electroporation (RE) is commonly used in many biological and medical applications such as electrochemotherapy of tumours and electrogene transfection [Citation5–7]. Irreversible electroporation (IRE) occurs under large electric field magnitudes leading to permanent membrane permeabilisation and cell death [Citation3,Citation8].
One of the most important applications of electroporation is in the field of cancer treatment. For example, RE-based electrochemotherapy combines the treatment of cancer lesions with an anti-cancer drug, followed by introducing a small electric field to the lesion [Citation9–11]. The electric field lasts for less than a second and creates pores in the membrane of the cells within the treated area during this time [Citation12]. This allows more anti-cancer drug to enter the cancer cells, which leads to a dramatic increase in the effectiveness of the treatment [Citation12]. In electrochemotherapy (or other application of RE), cell survival is essential for success. Electrochemotherapy possesses less side effects than systemic chemotherapy because cytotoxic drugs are locally injected into the tumour [Citation13]. The RE method has been studied extensively during the last 30 years while the IRE method has been considered as an undesirable side effect [Citation3]. However, during the last decade, IRE has begun to be viewed as a minimally invasive ablation technique, without using any toxic anti-cancer drug even in local injection manner [Citation3,Citation13–15]. Since IRE requires delivering a strong electric field to viable cells, maintenance of cell viability during treatment is essential.
In general, an IRE study should consider two aspects, e.g. electrical and thermal damage. As mentioned above, IRE uses electric field strengths in the upper limit of electrochemotherapy. For example, the IRE threshold is 900 V/cm for a train of 8 pulses each100 µs duration delivered at a frequency of 1 Hz for subcutaneous tumour [Citation16,Citation17]. Note that this threshold value is adopted from previously published studies with the set of pulses mentioned above [Citation17–19]. Electric fields stronger than this value create irreversible pores in the tissue (i.e. electrical damage). Furthermore, it is well known that every electric field produces Joule heating, and the heat produced can ablate normal or tumour tissue through three mechanisms: (1) phase changing in subcutaneous lipid caused by high temperature; (2) tissue disruption caused by phase changing in water due to high temperature; 3) cell death caused by long exposure to thermal change [Citation20].
The Joule heating can be related to electrical potential by the following expression:
in which σ is the electrical conductivity of the tissue and φ is the electrical potential. According to Equation 1, since electric field and consequent Joule heating are related, both thermal and electrical damage should be considered to estimate the real damage.
Although all cancer treatments have side effects, the appropriate treatment should cause minimum damage to the normal tissue. Experimental tumour ablation with IRE surgery conducted on animal tissue shows that non-thermal IRE (NTIRE) has many beneficial effects, such as 1) extremely rapid regeneration of ablated tissue with healthy tissue without scar formation, inducing a good immune response [Citation21], and 2) the ability to treat the heart [Citation22] and blood vessels [Citation23] without the danger of coagulation and subsequent emboli.
Therefore, it is better that IRE becomes NTIRE. The research on tumour ablation using IRE with minimum thermal damage presents an interesting field for scientists studying IRE. This paper deals with an analysis on geometric and electrical parameters that can be set before the beginning of the IRE treatment, in order to maximise direct thermal damage to tumour and minimise it to healthy tissue.
The survey of various parameters on electrical and thermal distribution can be determined by means of a numerical model [Citation18,Citation24–26]. Numerical modelling can therefore be used as a treatment planning tool, like Monte Carlo N-particle (MCNP) transport code for radiotherapy, to provide invaluable insight before beginning the treatment.
Numerical modelling is a technique to guesstimate the solutions of mathematical equations that model the physical processes in a system. The system is defined by the geometry and the physical properties of the material (i.e. tissue).
In this paper, we used the finite element method to calculate electrical and thermal distribution in tissue. Furthermore, unlike some papers that have considered a one-layer skin model [Citation16,Citation27], we considered several layers of skin with different electrical and thermal properties. The goal of this parametric study was to identify how geometric parameters of electrodes can be effective for electrical and thermal distributions in normal tissue and tumour.
Materials and methods
Electric field distribution in the tissue is determined by the governing equation [Citation24,Citation27]:
In Equation 2, σ and φ stand for the electrical conductivity of the tissue and the electrical potential, respectively.
A modified Pennes (bioheat) equation is used to determine temperature distribution. In order to consider the heat generated from the IRE procedure, Joule heating (Equation 1) is added to the original Pennes equation [Citation28]:
where k stands for the thermal conductivity of the tissue, T is the temperature, ωb is the blood perfusion, cb is the heat capacity of the blood, Ta is the arterial temperature, q″′ is the metabolic heat generation, ρ is the tissue density, and cp is the heat capacity of the tissue.
The purpose of the analysis is to determine the volume of normal and tumour tissue that undergo IRE (electrical analysis) and thermal damage caused by electrical pulse on both tissues (thermal analysis). The boundary between RE and IRE for subcutaneous tumour is 900 V/cm [Citation16], which is used as the criteria for calculating reversible and irreversible volume.
To examine thermal damage Ω, the Arrhenius equation was used [Citation24,Citation29]:
in which ξ is the frequency factor in 1/s, Ea is the activation energy in Joule/mole, R is the universal gas constant (Joule/mole.Kelvin), and T is the absolute temperature in Kelvin. It has been shown that Ω = 0.53 is the threshold for burn injuries in blood-perfused skin [Citation30,Citation31]. Since the Laplace and bioheat equations are linear, the produced data can be inter or extrapolated.
Model of the problem
In this article, we used a 3D model containing a subcutaneous tumour (a sphere with a diameter of 1.5 mm) in skin with four different layers of epidermis and stratum corneum (ED-SC), dermis (D), subcutaneous fat (SF), and subcutaneous tissue (ST) with thicknesses of 0.05, 1.1, 1.2, and 7.65 mm respectively (). The whole tissue was considered isotropic and homogeneous. Four different configurations of aluminium needle electrodes were considered in this model: 2-electrode, 4-electrode, hexagonal 2 × 2, and hexagonal 3 × 3 (). Based on similar works [Citation18,Citation21], needle electrodes were used in this work. The parameters of the tissues and electrodes are summarised in . It should be noted that the value of tumour conductivity was based on measurements taken at the end of an IRE procedure [Citation16].
Figure 1. Geometry of tumour and different layers of skin. (A) y–z view of tumour with respect to stratum corneum and epidermis (SC-ED), dermis (D), subcutaneous fat (SF) and subcutaneous tissue (ST) layers, (B) 3D geometry of model.
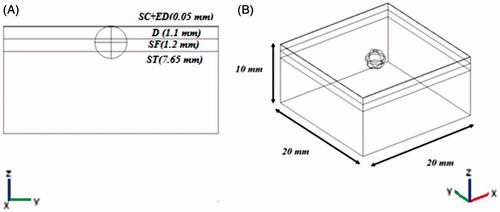
Figure 2. 3D configurations of different electrode geometry with respect to tumour and a four-layer skin model. (A) 2-electrode geometry, (B) 4-electrode geometry, (C) hexagonal geometry.
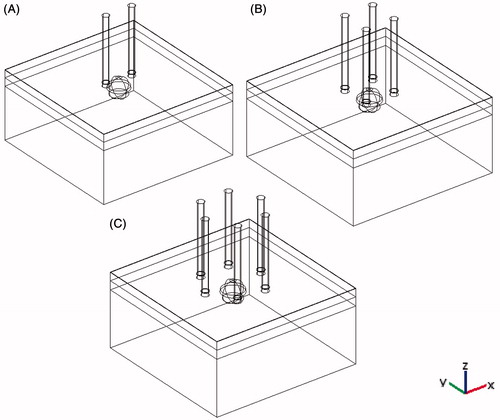
Table 1. Electrical and thermal properties of different tissues and electrodes used in simulations.
The selected parameters for estimating the electrical and thermal distribution in tissue are described as the distance between adjacent electrodes (b), distance between contrary electrodes (d), electrode insertion (i), electrode thickness (t), tumour depth (h), and voltage (v).These parameters are summarised in and depicted in . As shown in , b is defined only for hexagonal geometry. Because of symmetry in 2-electrode geometry, b and d are equal (). To survey each parameter, the base value of other parameters was fixed and its range changed as shown in .
Figure 3. Polarity of different electrode configurations and simulation parameters. (A) 2-electrode configuration, (B) 4-electrode configuration, (C) hexagonal 2 × 2 electrode configuration, (D) hexagonal 3 × 3 electrode configuration, (E) central y–z view of model and other geometric parameters.
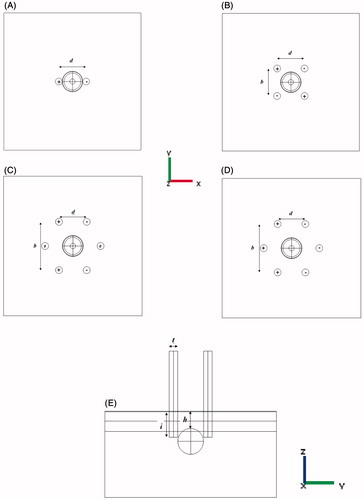
Table 2. Applied parameters in study, their step and base value.
Finite element simulation
In this study, the finite element method was used to solve Equations 2 and 3.
Creation of finite element model
Finite element analysis was performed using commercial finite element package Femlab v3.5a (Comsol AS, Stockholm, Sweden). A convergence test was done to ensure the adequate mesh density and validity of the simulation. More specifically, the simulation was begun with a mesh discretisation and then the solution was observed and recorded. Next step was to repeat the simulation with a finer mesh (i.e. more elements) and to compare the results with previous test. If the results were nearly similar (i.e. it was changed by less than 1%) then the previous mesh was probably good enough for that particular geometry. If the results differed by a large amount, it would be necessary to try a finer mesh. The number of elements for each electrode geometry (after doing the convergence test) is shown in . It should be noted that this number depends on the thickness of the electrodes.
Table 3. Number of elements for all electrode geometries. Number of elements in this table is for base value of geometric parameter.
Boundary and initial conditions
In order to solve Equation 2, we assume that the electrical boundary condition of the electrode is governed by
Electrical boundary condition of outer surface of tissue is electrically insulative and this will result in
Equations 5 and 6 are Dirichlet and Newman’s boundary conditions respectively.
To predict the maximum temperature rise in the tissue, it is considered that the outer surface domains are adiabatic:
Such boundary conditions that confine the heat into tissue therefore provide upper limit to the temperature distribution produced in the electroporation procedure [Citation24,Citation27].
Furthermore, the initial temperature of the tissue is set to 37 °C.
Eight to ten 100-μs pulses are normally used in typical RE and IRE procedures [Citation20,Citation29]. We selected 0.1 ms pulse width to estimate the maximum temperature rise in our model. It should be noted that tumour ablation with IRE can be done with shorter pulse widths [Citation40].
Results
Effect of parameters on electrical distribution
show the effect of six fundamental parameters on electrical distribution in normal and tumour tissue. For example, shows the effect of distance between contrary electrodes (d) on electric field distribution in both normal and tumour tissue for four different electrode configurations explained previously. For all electrode configurations, increasing d causes a slight increment in the volume of healthy tissue exposed to an electric field greater than 900 V/cm. (This volume was calculated using post processing features in FEMLAB software). This behaviour seems reasonable because as d increases, a greater volume of normal tissue becomes exposed to the electric field, even though the electric field between the electrodes decreases. The strong effect of d on electrical distribution in tumour is evident from these results. For hexagonal 3 × 3 geometry, a complete coverage of tumour was observed. However, for hexagonal 2 × 2 geometry, as the distance between electrodes increased, the volume of tumour exposed to electric field greater than 900 V/cm increased as well. This increment can be interpreted in a similar way as mentioned for the case of normal tissue. For 2- and 4-electrode geometries, the same increase was observed. However for d = 3 mm and greater distances, the volume was decreased. This decrement occurs since the larger distance between electrodes results in a weaker electric field. shows that for tumour ablation, regardless of thermal damage aspects, hexagonal 3 × 3 geometry for all d and hexagonal 2 × 2 geometry for d > 3 mm produced the best results (maximum tumour volume exposed to E > 900 V/cm and minimum one for normal tissue).
Figure 4. Relative irreversibly electroporated volume for normal and tumour tissue by distance between contrary electrodes (d).
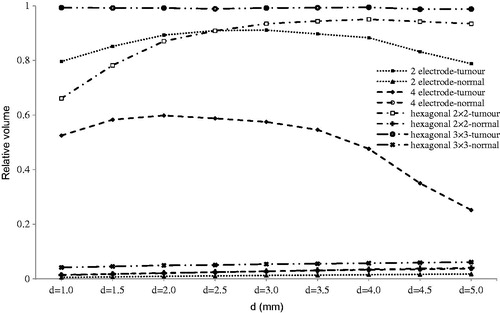
Figure 5. Relative irreversibly electroporated volume for normal and tumour tissue distance between adjacent electrodes (b).
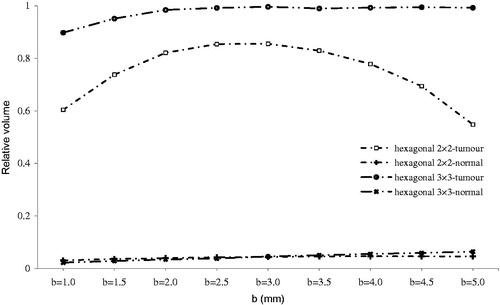
Figure 6. Relative irreversibly electroporated volume for normal and tumour tissue by electrode insertion (i).
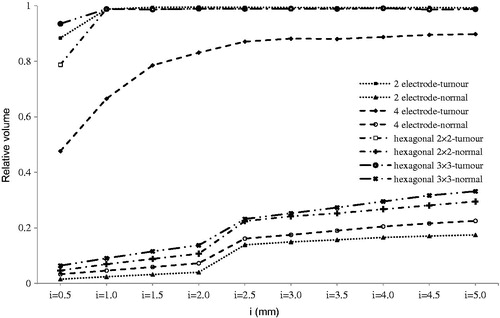
Figure 7. Relative irreversibly electroporated volume for normal and tumour tissue by electrode thickness (t).
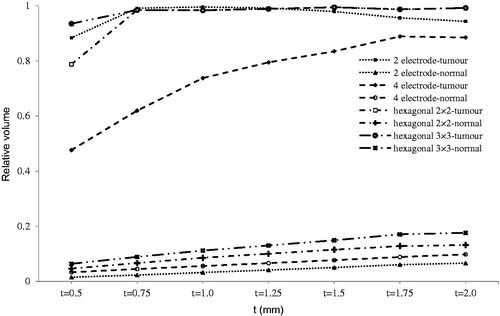
Figure 8. Relative irreversibly electroporated volume for normal and tumour tissue by tumour depth (h).
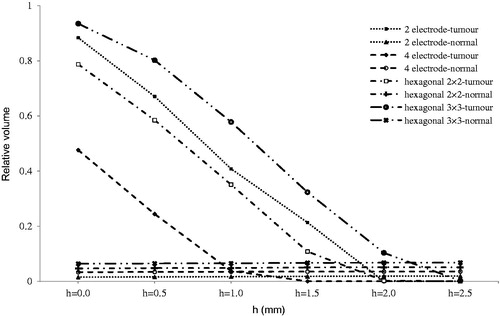
The results of evaluating the effect of distance between adjacent electrodes (b) are displayed in . This parameter is used only for hexagonal configurations, since in the case of 2-electrode configuration, there is no adjacent electrode, and for 4-electrode configuration the b and d are equal due to symmetry. We see that for hexagonal 3 × 3 there is a good tumour coverage for IRE while for hexagonal 2 × 2, in the best situation (b = 2.5 mm), less than 85% of the tumour was electroporated irreversibly.
As illustrated in , electrode insertion had considerable effect on normal tissue, unlike other parameters, in that irreversibly electroporated volume of normal tissue suddenly increased for i > 2 mm due to entering the electrode into subcutaneous tissue. As shown in , electrical conductivity of subcutaneous tissue is 0.41, which is approximately 20 times greater compared to electrical conductivity of subcutaneous fat (0.02). Therefore, when the electrode entered the subcutaneous tissue, irreversibly electroporated volume of normal tissue increased. When inserting the electrode into a deeper layer, coverage of tumour tissue has been improved, as shown in . However, it should be noted that damage to normal tissue increased as the electrode entered deeper into the tissue. According to , I = 2.5 mm produced the optimum results for all configurations, regardless of thermal aspects.
Figure 10. The effect of electrode insertion on electric distribution in tumour and normal tissue for 4-electrode configuration. (A) i = 0.5 mm, y–z view, (B) i = 0.5, x–y view, (C) i = 5 mm, y–z view, (D) i = 5 mm, x–y view. The effect of electrode insertion on irreversibly electroporated volume is obvious especially on normal tissue.
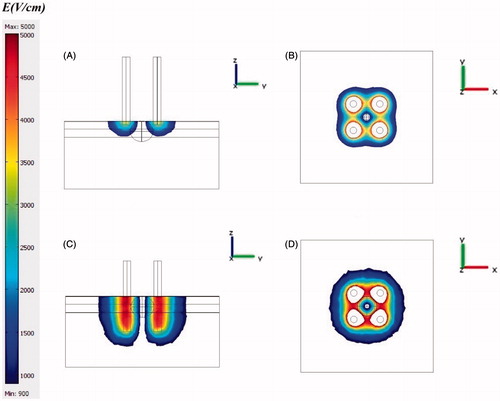
The effect of electrode thickness is shown in . Except for the 4-electrode configuration, complete tumour ablation was happening at t = 1 mm. Thicker electrodes increased damage to healthy tissue. In the 4-electrode geometry, 2 mm thickness could not ablate the entire tumour tissue, despite 6% electrical damage to normal tissue. Although increasing electrode thickness can increase IRE of the area (in both healthy and tumour tissue), it can be used to control the shape of the ablated area especially for the 4-electrode configuration. As shown in , although the IRE of the area was not contiguous, for t > 1.5 mm specified in this study, this area was contiguous. Such contiguity depends on distance between electrodes, voltage, and selected electrode configuration. Davalos and co-workers reported that such contiguity occurs for 1.5 mm electrode thickness, 1 mm electrode distance, and 1331 V [Citation40].
As shown in , treatment effectiveness is strongly influenced by depth of tumour. Therefore, it is strongly recommended to insert electrodes deeper to compensate for this effect.
According to , the 2-electrode configuration produced better results at low voltage, but for better interpretation, the thermal aspects that would produce minimum thermal damage need to be considered. In any case, it is obvious that increasing the applied voltage increases the normal and tumour electroporated volume.
Our results show that in all configurations the maximum electric field is obtained for the adjacent electrode, which is consistent with previous studies [Citation18,Citation29,Citation41]. Furthermore, increasing the number of electrodes can increase the electroporation volume (reversible and irreversible), which is also in line with these studies. In summary, the results indicated that geometry and position of electrode have significant influence on consequent electric field distribution, which is in accordance with other research in this area [Citation40–42]. Indeed, when considering electrode size, distance between electrodes, voltage, and electrode insertion, it is possible to extend or control the ablated area. The current study illustrated how each geometric or electrical parameters can change electric field distribution coverage in both target and healthy tissue.
Effect of parameters on thermal distribution
One of the most important features of IRE is that it does not induce thermal damage [Citation21,Citation43]. Thermal damage depends on both time of exposure and temperature [Citation44]. Therefore, exposure time and temperature of tissue are required parameters to complete calculation of thermal damage using the Arrhenius equation (Equation 4). It is assumed that tissue temperature is constant. If the temperature is constant, duration of heating with units of time can be used to express thermal dose. In reality, however, a constant temperature is not maintained; therefore, it is necessary to find a method to introduce an equivalent time at a specified reference temperature. Sapareto and Dewey introduced one such method to calculate thermal dose for changing temperature exposure [Citation45]. This technique uses numerical integration to calculate the time that would give an equivalent thermal dose at a reference temperature under different temperature conditions. The reference temperature has been set at 43 °C [Citation45]. The following expression is the duration required to lead to an equivalent thermal damage at 43 °C:
in which t43 is the equivalent time at 43 °C, Tt is the average temperature during Δt. A value of R equal to 0.25 was chosen for temperatures smaller than 43 °C and a value equal to 0.5 for temperatures higher than 43 °C [Citation24,Citation44,Citation45].
After calculating t43, it is possible to use the Arrhenius equation with constant temperature (43 °C) to calculate thermal damage.
As mentioned previously, thermal damage depends on both time of exposure and temperature. For example, thermal damage begins at 42 °C, with exposure between several seconds to hours. An exposure time of less than 1 s does not produce significant thermal damage [Citation46]. Nevertheless, damage is relatively low up to 50–60 °C, at which point the damage increases dramatically [Citation46,Citation47]. According to the literature, thermal damage (Ω) for minimum degree burns is 0.53 while it increases to 10,000 for third degree burns.
show logarithms of thermal damage in comparison to different parameters. In , distance between the opposing electrodes (d) can produce thermal damage only for 2-electrode geometry. In this case, when d was less than 3.5 mm, thermal damage increased dramatically. This sudden increase occurred because less than 3 mm of the electrode was inserted into the tumour; moreover when d was decreased the electric field increased, and this could be the other reason for the sudden rise mentioned.
Figure 11. Logarithm of thermal damage on normal and tumour tissue by distance between contrary electrodes (d).
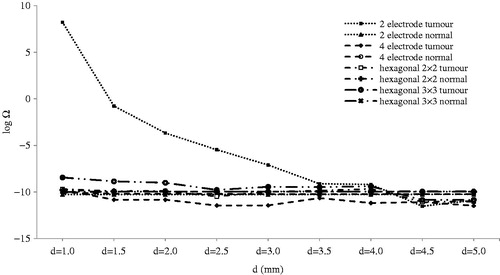
Figure 12. Logarithm of thermal damage on normal and tumour tissue by distance between adjacent electrodes (b).
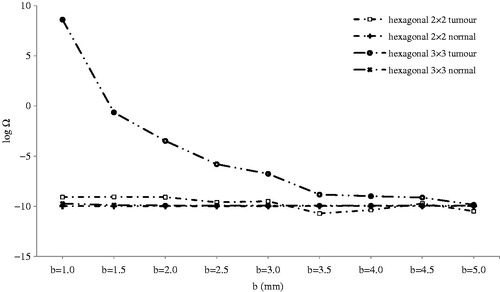
The same result was obtained for the distance between adjacent electrodes (b) for the hexagonal 3 × 3 configuration, as shown in . Except for tumour damage in hexagonal 3 × 3 geometry for b below 3.5 mm, other geometries incurred no critical thermal damage; therefore, they could be used without being concerned about thermal damage.
illustrates the effect of electrode insertion on thermal damage. Although thermal damage was lower than minimum degree burns for 1000 V, it should be noted that in addition to 2-electrode geometry, other geometries could potentially produce thermal damage, especially at a higher voltage at i > 2.5 mm. In these situations, electric field distribution should also be considered. Considering , we can see that for all geometries, electrodes inserted 2.5 mm (if 4-electrode geometry is not considered) into tumour tissue can lead to IRE of almost an entire tumour. Further insertion of electrodes increased electrical damage to normal tissue () in addition to producing a greater thermal damage to both normal and tumour tissues ().
Thermal effect of electrode thickness () is much more important when inserting electrodes into tumour tissue. Thermal damage is negligible when electrodes are not inserted into the tumour. This situation occurs in t ≥ 1 mm for 2-electrode geometry and t ≥ 1.75 mm for 4-electrode geometry. As shown in , thermal damage of tumour tissue increases dramatically when electrodes are inserted into the tumour tissue.
Tumour depth and voltage did not result in thermal damage in this study ( and ). Since there is no thermal damage, complete electrical coverage of the tumour with minimum electrical damage to normal tissue becomes the criteria for selection of appropriate geometry.
According to the thermal simulation, it is strongly recommended that electrodes are not inserted directly into tumour. This is because of the high electrical conductivity of tumour tissue to adjacent tissues () which results in severe thermal damage. Moreover, it was observed that due to Joule heating, tissue temperature depended strongly on electrode geometry and tissue electrical conductivity.
Our results confirmed that maximum temperature rise, and accordingly the highest electric field, is in vicinity of the electrode, which is also consistent with the results of previous studies [Citation27,Citation40]. It should be noted that increasing distance from the electrodes led to rapid temperature decrease, which is in agreement with pervious studies [Citation24,Citation27]. It means that immediately after delivering the pulse, thermal energy of high intensity does not reach distant electrodes. However, this thermal energy can spread throughout the tissue as time passes. Due to this fact, any geometric parameter that leads to more contact surface between the electrodes and tissues such as greater electrode thickness, deeper electrode insertion, or more electrodes can increase thermal damage to the tissue.
Limitations of the model
This parametric study showed the effect of geometric and electrical parameters on thermal and electrical damage. The effect of temperature on electrical and thermal conductivity, which is an important issue in heterogeneous tissue, was not within the scope of this modelling study. However, based on the fact that the electrical conductivity of tissue increases during electroporation procedure (e.g. up to 25% more than its initial value) [Citation48,Citation49], we considered a change in the conductivity of tumour at the end of IRE procedure [Citation17,Citation18]. As an example, ex vivo studies on rat liver showed the conductivity of 0.2 S/m when the tissue is exposed to an electric field of 200 V/cm, while the initial conductivity is 0.14 S/m under no electric field [Citation50]. It was shown that there was a sigmoid relationship between the specific conductivity and the magnitude of the electric field [Citation48,Citation49]. It should be mentioned that in this study we are dealing with relative variations in IRE of the area. Concerning absolute volume, changes in σ should be also taken into account. It means such a dynamic change in conductivity is important when the irreversibly electroporated area is reported as an absolute value. However, in situations where the aim of simulations is to evaluate how the irreversibly electroporated volume changes, the trend of relative variations is not affected. Therefore the same graphs will be produced even in the case of having a dynamic change in conductivity. In other words, such dynamic changes in σ have the same effect in all simulations and do not affect the relative results due to geometric changes. This study does not consider different tumour shapes and we assume that the tumour is surrounded by a sphere. For deeper tumours and/or tumours of a greater diameter, the thermal damage is more significant. In the case of deeper tumours, the electrodes must be inserted deeper into the tissue, which increases the thermal damage (). Similarly, for larger diameter tumours, the distance between electrodes (b or d) inevitably increases. Increasing the applied voltage can compensate the reduced applied electric field due to increased electrode separation, which in turn increases the thermal damage ().
Conclusion and summary
We demonstrated the effect of geometrical and electrical parameters on tissue ablation outcome with IRE based on a numerical modelling approach. This study considered both electrical and thermal aspects. The results showed that for complete tissue ablation with a minimum damage to normal tissue, it is necessary to consider both electrical and thermal distributions. Such scrutiny can be used to improve many electroporation-based clinical treatments, especially those involving tumour ablation with IRE.
As a recently introduced method of tumour ablation, IRE works based on the application of electric field, which produces Joule heating during IRE surgery. For tumour ablation, it is of utmost importance to cover the entire volume of the treated tumour with a sufficiently strong electric field. However unwanted side effects, especially thermal damage to normal and tumour tissue, should be minimised. Note that thermal damage can led to apoptosis (for lower temperature) or necrosis (for higher temperature) [Citation51]. Furthermore IRE ablation is based on voltage (or energy) applied to the tissue and there is no separation between ablated and viable tissue [Citation3,Citation40,Citation52]. Therefore it seems that treatment planning is needed to prevent extension of ablation zones into unwanted tissue.
Numerical modelling of RE and IRE has been shown to be an efficient tool to analyse outcomes of electroporation. Based on the finite element method we used numerical modelling to study the effects of both electrical and thermal distributions during IRE surgery. Investigation of the effects of geometrical and electrical parameters on the volume of the irreversibly electroporated tissue, and performing a thermal study on normal and tumour tissues were the objectives of this study. The results may be employed by clinicians to use a proper set of geometrical and electrical parameters of electrodes for IRE surgery. In this regard our findings show that the complete coverage of tumour by electric field can lead to dramatic thermal damage which may affect the entire treatment process. Therefore, the complete coverage of tumour should not be used as the only criterion to select electrode parameters. In addition, it was shown that some parameters, such as tumour depth as well as voltage and electrode insertion depth, do not contribute considerably in the process of thermal damage. However, the distance between electrodes and their thickness has remarkable effects on thermal damage distribution. Furthermore, our findings demonstrated the importance of considering the combined effect of parameters. For example, increasing the electrode insertion-depth and applying voltage at the same time can lead to tissue burns.
In summary a delicate balance between applied electric field and the expected thermal damage should be considered by clinicians as crucial criteria in choosing the parameters of IRE surgery.
Declaration of interest
The authors report no conflicts of interest. The authors alone are responsible for the content and writing of the paper.
References
- Miklavcic D, Puc M. Electroporation. Wiley Encyclopedia of Biomedical Engineering 2006. doi: 10.1002/9780471740360.ebs1390
- Neumann E, Sowers A, Jordan C. Electroporation and Electrofusion in Cell Biology. New York: Plenum; 1989
- Rubinsky B. Irreversible electroporation in medicine. Technol Cancer Res Treat 2007;6:255–60
- Miklavcic D, Mali B, Kos B, Heller R, Sersa G. Electrochemotherapy: From the drawing board into medical practice. Biomed Eng Online. Available from: http://www.ncbi.nlm.nih.gov/pmc/articles/PMC3995705/
- Puc M, Flisar K, Rebersek S, Miklavcic D. Electroporator for in vitro cell permeabilization. Radiol Oncol 2001;35:203–7
- Pavselj N, Preat V. DNA electrotransfer into the skin using a combination of one high- and one low-voltage pulse. J Control Release 2005;106:407–15
- Oblak J, Krizaj D, Amon S, Macek-Lebar A, Miklavcic D. Feasibility study for cell electroporation detection and separation by means of dielectrophoresis. Bioelectrochemistry 2007;71:164–71
- Al-Sakere B, Andre F, Bernat C, Connault E, Opolon P, Davalos RV, et al. Tumor ablation with irreversible electroporation. PLOS ONE 2007;2:e1135
- Mir LM, Orlowski S. Mechanisms of electrochemotherapy. Adv Drug Deliv Rev 1999;35:107–18
- Cemazar M, Miklavcic D, Mir LM, Belehradek J, Bonnay M, Fourcault D, et al. Electrochemotherapy of tumours resistant to cisplatin: A study in a murine tumour model. Eur J Cancer 2001;37:1166–72
- Sadadcharam M, Soden DM, O’Sullivan GC. Electrochemotherapy: An emerging cancer treatment. Int J Hyperthermia 2008;24:263–73
- Sersa G, Cemazar M, Miklavcic D, Rudolf Z. Electrochemotherapy for tumors. Radiol Oncol 2006;40:163–74
- Sersa G, Stabuc B, Cemazar M, Miklavcic D, Rudolf Z. Electrochemotherapy with cisplatin: The systemic antitumour effectiveness of cisplatin can be potentiated locally by the application of electric pulses in the treatment of malignant melanoma skin metastases. Melanoma Res 2000;10:381–5
- Lu DSK, Kee ST, Lee EW. Irreversible electroporation: Ready for prime time? Tech Vasc Intervent Radiol 2013;16:277–86
- Dunki-Jacobs EM, Philips P, Martin RC II. Evaluation of resistance as a measure of successful tumor ablation during irreversible electroporation of the pancreas. J Am Coll Surg 2014;218:179–87
- Corovic S, Zupanic A, Miklavcic D. Numerical modeling and optimization of electric field distribution in subcutaneous tumor treated with electrochemotherapy using needle electrodes. IEEE Trans Plasma Science 2008;36:1665–72
- Pavselj N, Bregar Z, Cukjati D, Batiuskaite D, Mir LM, Miklavcic D. The course of tissue permeabilization studied on a mathematical model of a subcutaneous tumor in small animals. IEEE Trans Biomed Eng 2005;52:1373–81
- Semrov D, Miklavcic D. Calculation of the electrical parameters in electrochemotherapy of solid tumours in mice. Comput Biol Med 1998;28:439–48
- Zupanic A, Corovic S, Miklavcic D. Optimization of electrode position and electric pulse amplitude in electrochemotherapy. Radiol Oncol 2008;42:93–101
- Martin GT, Pliquett UF, Weaver JC. Theoretical analysis of localized heating in human skin subjected to high voltage pulses. Bioelectrochemistry 2002;57:55–64
- Rubinsky B, Onik G, Mikus P. Irreversible electroporation: A new ablation modality – Clinical implications. Technol Cancer Res Treat 2007;6:37–48
- Lavee J, Onik G, Mikus P, Rubinsky B. A novel nonthermal energy source for surgical epicardial atrial ablation: Irreversible electroporation. Heart Surg Forum 2007;10:E162–7
- Maor E, Ivorra A, Leor J, Rubinsky B. The effect of irreversible electroporation on blood vessels. Technol Cancer Res Treat 2007;6:307–12
- Edd JF, Davalos RV. Mathematical modeling of irreversible electroporation for treatment planning. Technol Cancer Res Treat 2007;6:275–86
- Miklavcic D, Corovic S, Pucihar G, Pavselj N. Importance of tumour coverage by sufficiently high local electric field for effective electrochemotherapy. Eur J Cancer Suppl 2006;4:45–51
- Edd JF, Horowitz L, Davalos RV, Mir LM, Rubinsky B. In vivo results of a new focal tissue ablation technique: Irreversible electroporation. IEEE Trans Biomed Eng 2006;53:1409–15
- Davalos RV, Rubinsky B. Temperature considerations during irreversible electroporation. Int J Heat Mass Transfer 2008;51:5617–22
- Pennes HH. Analysis of tissue and arterial blood flow temperatures in the resting forearm. J Appl Physiol 1948;1:93–122
- Shafirstein G, Novák P, Moros EG, Siegel E, Hennings L, Kaufmann Y, et al. Conductive interstitial thermal therapy device for surgical margin ablation: In vivo verification of a theoretical model. Int J Hyperthermia 2007;23:477–92
- Jiang SC, Ma N, Li HJ, Zhang XX. Effects of thermal properties and geometrical dimensions on skin burn injuries. Burns 2002;28:713–17
- Diller KR, Hayes LJ. A finite element model of burn injury in blood-perfused skin. J Biomech Eng 1983;105:300–7
- Duck FA. Physical Properties of Tissues: A Comprehensive Reference Book. London: Academic Press; 1990
- Svaasand L, Randeberg L, Aguilar G, Majaron B, Kimel S, Lavernia E, et al. Cooling efficiency of cryogen spray during laser therapy of skin. Lasers Surg Med 2003;32:137–42
- Chato JC, Lee RC. The future of biothermal engineering. Ann New York Acad Sci 1998;858:1–20
- Majchrzak E, Paruch M. Numerical modelling of the cancer destruction during hyperthermia treatment. Comput Methods Mech (Warsaw) 2011;9–12 May:1–2
- Wilson SB, Spence VA. A tissue heat transfer model for relating dynamic skin temperature changes to physiological parameters. Phys Med Biol. Available from: http://iopscience.iop.org/0031-9155/33/8/001;jsessionid=B93C593EBF7A4C66F31EEE8997F22569.c4
- He Y, Shirazaki M, Liu H, Himeno R, Sun Z. A numerical coupling model to analyze the blood flow, temperature, and oxygen transport in human breast tumor under laser irradiation. Comput Biol Med 2006;36:1336–50
- Becker SM, Kuznetsov AV. Thermal damage reduction associated with in vivo skin electroporation: A numerical investigation justifying aggressive pre-cooling. Int J Heat Mass Transfer 2007;50:105–16
- Wainwright P. Thermal effects of radiation from cellular telephones. Phys Med Biol 2000;45:2363–72
- Davalos RV, Mir IL, Rubinsky B. Tissue ablation with irreversible electroporation. Ann Biomed Eng 2005;33:223–31
- Corovic S, Pavlin M, Miklavcic D. Analytical and numerical quantification and comparison of the local electric field in the tissue for different electrode configurations. Biomed Eng Online. Available from: http://www.biomedical-engineering-online.com/content/6/1/37
- Miklavcic D, Beravs K, Semrov D, Cemazar M, Demsar F, Sersa G. The importance of electric field distribution for effective in vivo electroporation of tissues. Biophys J 1998;74:2152–8
- Maor E, Ivorra A, Rubinsky B. Non-thermal irreversible electroporation: Novel technology for vascular smooth muscle cells ablation. PLoS One 2009;4:e4757
- Damianou C, Hynynen K, Fan X. Application of the thermal dose concept for predicting the necrosed tissue volume during ultrasound surgery. Proc Ultrason Symp IEEE 1993;2:1199–202
- Sapareto SA, Dewey WC. Thermal dose determination in cancer therapy. Int J Radiat Oncol Biol Phys 1984;10:787–800
- Tropea BI, Lee RC. Thermal injury kinetics in electrical trauma. J Biomech Eng 1992;114:41–250
- Diller KR, Young IC. Modeling of bioheat transfer processes at high and low temperatures. Adv Heat Transfer 1992;22:157–357
- Neal RE, Garcia PA, Robertson JL, Davalos RV. Experimental characterization and numerical modeling of tissue electrical conductivity during pulsed electric fields for irreversible electroporation treatment planning. IEEE Trans Biomed Eng 2012;59:1076–85
- Sel D, Cukjati D, Batiuskaite D, Slivnik T, Mir LM, Miklavcic D. Sequential finite element model of tissue electropermeabilization. IEEE Trans Biomed Eng 2005;52:816–27
- Davalos RV, Otten DM, Mir LM, Rubinsky B. Electrical impedance tomography for imaging tissue electroporation. IEEE Trans Biomed Eng 2004;51:761–7
- Faroja M, Ahmed M, Appelbaum L, Ben-David E, Moussa M, Sosna J, et al. Irreversible electroporation ablation: Is all the damage nonthermal? Radiology 2013;266:462–70
- Wimmer T, Srimathveeravalli G, Gutta N, Ezell PC, Monette S, Maybody M, et al. Planning irreversible electroporation in the porcine kidney: Are numerical simulations reliable for predicting empiric ablation outcomes? Cardiovasc Intervent Radiol 2014 [E-pub ahead of publication]