Abstract
Purpose: Heat generated by magnetic nanoparticle clusters (MNCs) in an alternating magnetic field (AMF) can be used for hyperthermia cancer treatment. Here, we have synthesised polyacrylic acid-coated MNCs according to previous report, with the ability to increase particle stability in suspension. Radiosensitisation effects of the MNCs under an AMF were investigated in vitro and in vivo.
Materials and methods: MTT assay, flow cytometry, clone formation assay, Western blotting, and a γ-H2AX experiment were used to explore the biocompatibility and radiosensitisation effect of the MNCs and their putative radiosensitisation mechanism. An NCI-H460 mouse xenograft model was used to investigate the anti-tumour effect under an AMF in vivo.
Results: The temperature of MNC fluids at different concentrations (200 μg/mL to 2 mg/mL) increased rapidly. The MNCs were endocytosed by the cells and were found to be biocompatible. Hsp70 and caspase-3 were found to be up-regulated upon MNCs under an AMF, radiation, and combination of both treatments. MNCs under an AMF efficiently radiosensitised both CNE-2 cells and NCI-H460 cells. Finally, the tumour inhibition rate after treatment with MNCs under an AMF and radiation was significantly higher than that after either treatment alone. The mechanism of radiosensitisation putatively involves inhibition of DNA repair and induction of apoptosis.
Conclusions: The MNC fluids under an AMF enhanced the radiosensitivity of tumour cells both in vitro and in vivo.
Introduction
To date, cancer is still one of the leading causes of death worldwide, despite decades of research and numerous clinical trials. Apart from the fact that the disease has many aetiologies and pathological manifestations, the lack of understanding of the mechanisms underlying tumour development limit the development of effective new therapies. Chemotherapy, radiation therapy, and surgery have been the principle therapies for treating cancer to date, while additional procedures such as therapeutic hyperthermia have been explored.
Hyperthermia, which refers to the therapeutic killing and destruction of cancer cells when exposed to temperatures above 42 °C, offers a promising avenue to cancer treatment, as pointed out by decades of clinical research and pre-clinical trials [Citation1]. Traditional hyperthermia methods including microwave ablation, radiofrequency ablation, and high-intensity focused ultrasound have been introduced to treat solid tumours. However, there are still some limitations to these methods that have to be overcome prior to their standard therapeutic application. Due to poor intratumoural perfusion of heat and the inability to target hyperthermia directly to tumours, which results in damage to healthy tissues, traditional hyperthermia often has an unsatisfactory therapeutic effect [Citation2–5].
The effectiveness of hyperthermia for nasopharyngeal carcinoma (NPC) and lung cancer has been studied in both theoretical and clinical research. Hyperthermia combined with radiotherapy or chemotherapy can increase the drug concentration in tumour cells or reduce the required radiation dose, thus augmenting anticancer effects and mitigating side effects. The long-term efficacy of microwave hyperthermia combined with chemoradiotherapy for treating NPC with metastatic foci in cervical lymph nodes was evaluated by Kang et al. [Citation6]. It has been shown that microwave hyperthermia combined with chemoradiotherapy can increase local control, disease-free survival and 3- and 5-year overall survival rates of patients with N2 ∼ N3 stage NPC. Tamarov et al. studied the effect of strong radio frequency (RF) radiation-induced heating of semiconductor silicon-based nanoparticles in aqueous colloidal solutions to treat Lewis lung carcinoma in vivo [Citation7]. They demonstrated that Si nanoparticles excited by the RF radiation of relatively low intensity strongly inhibited the tumour growth, thus providing a promising approach for hyperthermia and/or combined therapy of cancer. These studies indicated that hyperthermia alone or in combination with chemoradiotherapy can be effectively applied to treat NPC and lung cancer.
The advent of nanotechnology provided a promising novel solution with magnetic hyperthermia, which can induce heat locally. Superparamagnetic nanoparticles can generate heat under an alternating magnetic field (AMF) through Neel and Brownian relaxation mechanisms; this has attracted considerable interest among researchers in recent years [Citation8,Citation9]. Because the heat can be adjusted by modifying the size and concentration of the magnetic particles, this technique overcomes some obstacles of traditional hyperthermia.
A series of in vitro experiments and clinical trials have shown magnetic hyperthermia to be effective for cancer treatment when combined with radiotherapy or chemotherapy [Citation10–14]. Magnetic hyperthermia shows a synergistic effect when combined with radiation therapy because hyperthermia is most effective against S-phase cells, which are the least sensitive to radiation [Citation15]. Additionally, tumour tissues are poorly oxygenated, and while they are resistant to radiotherapy, hypoxic cells are more sensitive to hyperthermia [Citation16]. Radiation is known to induce cell death owing to double-strand breaks (DSBs) [Citation17]. To date it is not yet clear whether magnetic hyperthermia can enhance DSB repair, thus leading to a better therapeutic potential.
Superparamagnetic iron oxide (SPIO) particles are widely used in applications such as contrast-enhanced magnetic resonance imaging (MRI) [Citation18], drug or gene delivery [Citation19–21], cell or protein separation [Citation22,Citation23], magnetic hyperthermia [Citation24], and other applications in the medical field. There are various methods to synthesise SPIO, including co-precipitation, thermal decomposition, and solvothermal reduction [Citation25–27]. SPIO nanoparticles are often modified with various organic materials, including polyethylene glycol (PEG), dextran, and polyethylenimine (PEI), to prolong their blood half-life and increase their stability in aqueous solutions [Citation28–30]. Clustered magnetic nanoparticles were also shown to have a much better heating effect than individual magnetic nanoparticles [Citation31]. Despite these modifications, the stability of iron oxides in solution remains of great concern and limits their applicability for the diagnosis and effective treatment of cancer. Polyacrylic acid (PAA), a hydrophilic polymer that contains carboxyl groups capable of binding to SPIO nanoparticles, allows stable dispersion of the particles in water [Citation32]. Moreover, the functional carboxyl groups of PAA might increase the potential targeting capacity of the magnetic particles. Therefore, PAA is an excellent surfactant candidate for the modification of SPIO nanoparticles.
To the best of our knowledge, the heating efficacy of PAA-coated magnetic nanoparticle clusters (MNCs) under an AMF and their radiosensitisation efficacy and putative radiosensitisation mechanism have not been studied to date. In this study, we used MNCs coated with PAA to explore their efficacy under an AMF and radiosensitisation, and we explored their putative mode of action. Our results may offer a prospect for the effective treatment of malignant tumours by MNCs under an AMF combined with radiotherapy.
Materials and methods
Reagents
PAA was purchased from Acros Organics (Geel, Antwerp, Belgium). Ferric chloride hexahydrate (FeCl3·6H2O, 97%), ethylene glycol and urea were purchased from Sinopharm Chemical Reagent (Beijing, China). Nanosized Fe3O4 powder was obtained from Aladdin Reagents (Shanghai, China). Deionised (DI) water was used for all experimental procedures, including preparation of solutions. All reagents were of analytical grade and were used without further purification.
Cell culture
Human NPC cell line (CNE-2), human lung cancer cell (NCI-H460), and human bronchial epithelial cell lines (BEAS-2B) were obtained from the Cell Bank of Xiangya School of Medicine, Central South University and cultured in Roswell Park Memorial Institute 1640 medium (RPMI-1640) and BEAS-2B cells were cultured in Dulbecco's modified Eagle medium (DMEM), supplemented with 10% fetal bovine serum (HyClone, Logan, UT), 100 U/mL penicillin, and 100 μg/mL streptomycin. The cells were cultured in a humidified incubator at 37°C with 5% CO2.
Synthesis and characterisation of PAA-coated magnetic nanoparticle clusters
The MNCs were prepared by the solvothermal reduction method as previously described [Citation33]. The size distribution of dispersed MNCs was analysed by dynamic light scattering (Malvern, Worcestershire, UK). Transmission electron microscopy (TEM) was used to analyse the size and morphology of the MNCs; images were acquired with an H-7000 FA electron microscope (Hitachi, Japan). X-ray diffraction (XRD) using an X-ray diffractometer (PANalytical, Almelo, Netherlands) was used to investigate the crystalline structure and phase purity of the MNCs. Fourier transform infrared (FTIR) spectroscopy (VERTEX70, Bruker, Ettlingen, Germany) was used to assess the adsorption of PAA on the MNC surface. The magnetic properties of the MNCs were identified using a vibrating sample magnetometer (VSM) (HH-15, Nanjing, China). Thermogravimetric analysis (TGA) was carried out using the Pyris1 TGA instrument (PerkinElmer, Waltham, MA) by heating the MNCs from 100°C to 800°C, at a heating rate of 10°C/min.
In vitro measurement of thermal characteristics of MNC fluids
MNC fluids with nanoparticle concentrations of 200, 400, 800, 1000, and 2000 μg/mL and Fe3O4 fluid (400 μg/mL) were prepared. After sonication, MNC fluids, Fe3O4 fluid, and DI water were exposed to an alternating magnetic field (P = 12 kW; f = 30–80 kHz; I = 30 A; H0 = 500 A m−1) in a high frequency induction heater (GY-25AB, Dongguan, China) for 60 min. The temperature of the MNC fluids was measured with a digital infrared thermometer (CEM DT-811, Hua Shengchang, China) at 5-min intervals, and temperature profiles were recorded.
Confocal laser scanning microscopy and transmission electron microscopy (TEM)
For confocal laser scanning microscopy (CLSM), CNE-2 and NCI-H460 cells were seeded into 24-well plates at the rate of 2.5 × 104 cells/well and incubated with Rhodamin123 (Rh123)-conjugated MNCs as previously described [Citation34]. After 6 h of incubation with MNCs, the cells were washed twice with phosphate-buffered saline (PBS) and subsequently fixed with 4% paraformaldehyde. The cells were counterstained with Hoechst 33342 fluorescent nucleic acid stain for 10 min, washed three times with PBS, and mounted with mounting medium (Beyotime, Jiangsu, China). The cells were subsequently examined by confocal laser scanning microscopy.
For TEM, CNE-2 and BEAS-2B cells were incubated with MNCs for 24 h and washed with PBS. The cells were digested with 0.25% trypsin and centrifuged at 1000 rpm for 5 min. The cells were fixed in 2.5% glutaraldehyde, washed three times with 0.1 M dimethyl sodium arsenate, and post-fixed in 0.1% osmic acid for 2 h. Afterwards, the cells were washed with DI water and dehydrated in a graded series of ethanol (30%, 50%, 70%) at 4°C for 10 min each, and a graded series of acetone (80%, 90%, 95%, 100%) for 10 min each. The cells were infiltrated with an epoxy resin-containing solution for 4 h and transferred to undiluted epoxy resin and polymerised at 35°C, 45°C, and 60°C, for 24 h at each temperature. Ultrathin sections were cut using an ultra-microtome and were stained with aqueous solutions of uranyl acetate and lead citrate. Sections were washed with 1% NaOH and DI water, and examined with the H-7000FA microscope.
MTT assay to assess the effect of MNCs on cell proliferation
Cancer (CNE-2 and NCI-H460) and normal (BEAS-2B) cells in the logarithmic growth phase were digested, diluted to single-cell solutions by using fresh complete culture medium, and were seeded into 96-well plates at a density of 5 × 103 cells/well. After 24 h of incubation, various concentrations of MNCs were added to each well. The cells were cultured in an incubator containing 5% CO2 at 37°C for 24, 48, and 72 h. After incubation, the cells were washed three times with fresh PBS, 20 μL of MTT (BioSharp, Hefei, China) was added to each well, and the cells were further cultured for 4 h. The culture medium was replaced with 150 μL of DMSO (Sigma-Aldrich, St Louis, MO) to dissolve intracellular Formosan, and the plates were gently shaken in the dark for 10 min. The optical density (OD) in each well was measured at a wavelength of 570 nm and a reference wavelength of 630 nm on a microplate reader (Multiskan MK3-353, Thermoscientific, Waltham, MA). The cell viability was calculated as follows: cell viability (%) = (OD of the experimental group/OD of the control group) × 100%.
Flow cytometry
CNE-2 and NCI-H460 cells were seeded into 6-well plates and grouped according to the various treatments employed, as follows: Ctrl, control; NP, MNCs alone; NP + R, cells incubated with MNCs exposed to irradiation; NP + AMF, cells incubated with MNCs subjected to AMF; R + NP + AMF, cells incubated with MNCs irradiated and subsequently exposed to AMF. MNCs with final concentration of 400 μg/mL were added to the groups NP, NP + AMF, and R + NP + AMF after 1 day of incubation. Group NP + AMF was subjected to an AMF for 60 min by using a mid-frequency heating coil plate (P = 12 kW; f = 30–80 kHz; coil number = 7 cm; turn number = 7). Group R was irradiated with a 4-Gy dose of 6 MV X-ray unit (PRIMUS.K, Siemens, Munich, Germany) and group NP + R was incubated with MNCs and then irradiated. Group R + NP + AMF was first irradiated and then subjected to an AMF.
The cells were further cultured at 37°C in an incubator with 5% CO2 for 24 h. For the analysis of apoptosis, cells were collected and stained using the Annexin V-EGFP Apoptosis Detection Kit (BIO-BOX Biotech, Nanjing, China) according to the manufacturer's instructions. To assess the DNA content and cell cycle distribution of the cells, a PI/RNAse staining solution was added to the harvested cells. Within 1 h, cells were analysed on a flow cytometer (BD FACS Vantage SE, BD Biosciences, San Jose, CA), and data were analysed with ModFit LT software. The experiments were repeated three times.
Clone formation assay for radiosensitivity
CNE-2 and NCI-H460 cells were seeded in triplicate into 6-well plates and allowed to adhere overnight. MNCs (400 μg/mL, for R + NP + AMF and NP + R treatment) or complete culture medium (for R treatment) was added to the wells, and the cells were irradiated with of doses 0, 2, 4, 6, and 8 Gy. The cells of the R + NP + AMF group were subjected to an AMF for 60 min after irradiation and the cells of the NP + R group were incubated with MNCs and then irradiated with the above-mentioned doses. The cells were further cultured for approximately 14 days to form distinct cell clusters. A cluster comprising more than 50 cells as evaluated using a light microscope, was regarded a clone. The cells were stained with methyl violet dye. Plating efficiency (PE) and survival fraction (SF) were calculated as follows: PE = (number of clusters/number of seeded cells) × 100%, SF = {PE (R + NP + AMF)/PE (R)} × 100%. The data were analysed with Graphpad Prism 5.0 software (San Diego, CA).
Analysis of γ-H2AX foci experiment
CNE-2 and NCI-H460 cells were seeded into each well of 24-well plates, and were grouped according to the various treatments they were subjected to as follows: R, radiation alone; NP + R, MNCs with radiation; and R + NP + AMF, MNCs were irradiated and subsequently exposed to an AMF. After the cells had adhered overnight, MNCs (400 μg/mL) were added to the NP + R and R + NP + AMF group, and complete culture medium was added to the R group. The cells were exposed to 2-Gy X-rays under a linear accelerator. The R + NP + AMF group was heated for 60 min after irradiation. Cells were collected at various time points after radiation (2, 4, 8, 12, and 24 h) and were immediately fixed with 4% paraformaldehyde for 15 min. Then, 0.2% Triton X-100 was added at 4°C for 15 min, after which 5% bovine serum albumin (BSA) was added at 37°C for 30 min. The cells were incubated with monoclonal primary antibody against γ-H2AX (Abcam, Cambridge, UK) at 4°C overnight, and subsequently with DyLight 549-labelled goat anti-mouse IgG (EarthOx, San Francisco, CA) secondary antibody at 37°C for 50 min. The cells were counterstained with Hoechst33342 fluorescent dye for 10 min and mounted with mounting medium. Random fields containing more than 100 cells were examined with the confocal laser-scanning microscope.
Western blotting
The cells were lysed on ice for 30 min using lysis buffer and then centrifuged at 12,000 rpm at 4°C. Proteins were resolved on a 12% SDS-PAGE gel, transferred to polyvinylidene difluoride membranes and blocked with 5% BSA. Membranes were incubated with monoclonal rabbit anti-human primary antibodies against Hsp70 and caspase-3 (Epitomics, Burlingame, CA) at 4°C overnight, followed by rinsing with Tris-buffered saline with Tween-20 three times for 30 min. The cells were then incubated with horseradish peroxidase-conjugated secondary antibody at room temperature for 1 h. The immunoreactive protein bands were visualised using an enhanced chemiluminescence Western blotting system (Google Biotechnology, Wuhan, China) and band intensities were quantified with Image J software.
In vivo experiments in nude mice with lung cancer
Four- to five-week-old female BALB/c nude mice were obtained from HFK bioscience (Beijing, China). The experiment was approved by the Experimental Animal Ethics Committee of Tongji Medical College, Huazhong University of Science and Technology, and was performed in accordance with institutional guidelines. The mice were maintained in super pathogen-free conditions with 12-h light/dark cycles. NCI-H460 tumour cells in the exponential growth phase were harvested and resuspended in 200 μL of serum-free RPMI-1640 medium. The mice were injected subcutaneously at the left posterior limb rump with 5 × 106 NCI-H460 tumour cells. When the tumours reached a mean volume of approximately 80–100 mm3, mice were randomly assigned into four treatment groups (four mice per group) as follows: Ctrl, control; R, radiation; NP + AMF, MNCs under an AMF; R + NP + AMF, MNCs were irradiated and subsequently exposed to an AMF. To ensure homogenous fluid dispersion in the tumour tissues, we applied a multipoint injection strategy. The mice were anaesthetised by injecting 2% sterile phenobarbital sodium into the enterocoelia. Tumours were injected with 200 μL of PBS (Ctrl and R group) or 200 μL MNCs (4 mg/mL; NP + AMF and R + NP + AMF group). The mice of the R and R + NP + AMF groups were subjected to a dose of 10 Gy of 6 MV X-rays under a linear accelerator. The mice of NP + AMF and R + NP + AMF groups were exposed to an AMF for 1 h. The tumour diameters were measured every 2 days using a digital caliper and were recorded for 28 days following treatment. The tumour volume (TV) was calculated by the following formula: TV = (length × width2)/2. The growth inhibition rate was calculated as (1 − mean TV of the experimental group / mean TV of the control group) × 100%.
Histological analysis of PAA-Fe3O4 distribution in the tumour
PAA-Fe3O4 fluid (4 mg/mL) was injected into the transplantable tumour of BALB/c mice using a multipoint injection strategy. Seven days after injection, the mice were deeply anaesthetised and the tumour was resected. The resected transplantable tumour tissue was post-fixed with 4% paraformaldehyde for 24 h. The sample tissue was stained with Pearl's Prussian blue solution and haematoxylin and eosin (H&E), following the manufacturer's instructions.
Statistical analysis
Data are shown as the mean ± standard deviation and were analysed with GraphPad Prism 5.0 software. Student's t-test was used for comparing the means of groups; p < 0.05 was considered to be statistically significant.
Results
Structural and magnetic characteristics of the PAA-coated MNCs
The MNCs were nearly spherical and their diameters were indicated to be approximately 70 nm detected by dynamic light scattering and approximately 50 nm by TEM ( and ). The XRD pattern showed characteristic peaks at 2θ values (220), (311), (400), (422), and (511), indicating that the MNCs had a cubic inverse spinel structure. The grain size of the MNCs was calculated from the XRD data using the Scherrer equation: D = Kλ/(β·cosθ), where D is the grain size of the MNCs, K is the Scherrer constant (0.9), λ is the X-ray wavelength (0.154178 nm), β stands for the full width at half maximum (in radians) of the diffraction peaks, and θ is the diffraction angle of the XRD patterns [Citation35]. The mean grain size of the MNCs obtained using this equation was 7.33 nm.
Figure 1. Characteristics of the PAA-coated MNCs. (A) Histogram of diameter distribution as measured by dynamic light scattering. (B) TEM micrographs of the MNCs. (C) XRD pattern of the MNCs. (D) FTIR spectrum of the MNCs. (E) Magnetisation curve of the MNCs. MNCs were tested using a vibrating sample magnetometer at room temperature (T = 300 K). (F) TGA curve of the MNCs.
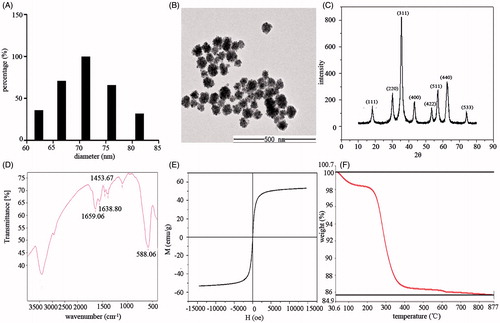
Further characterisation of the MNCs was performed by analysing their FTIR spectra (). The peak at 588.06 cm−1 is attributed to the Fe–O vibration of Fe3O4. Peaks at 1453.67, 1638.80, and 1659.06 cm−1 are peaks characteristic of the asymmetric and symmetric stretching vibrations of the COO− groups chelated to the particle surface, which confirmed that PAA was covalently linked to the surface of the MNCs.
To investigate the magnetic property of MNCs, the magnetisation curve at room temperature was recorded by VSM. The magnetisation curve showed characteristics that indicated a superparamagnetic behaviour of the MNCs: a negligible coercivity and remanence, and a saturation magnetisation value of 53 emu/g, demonstrating a strong relative magnetisation saturation value ().
The TGA curve of the MNCs is shown in . The weight loss in the temperature region ranging from 100°C to 877°C was approximately 15%. The weight loss in the regions 200–390°C and 390–800°C was due to dehydration of the carboxylic group of PAA and the decomposition of carbon chains, respectively, which demonstrated that PAA was successfully chelated onto the surface of the MNCs.
In vitro thermal characteristics of the PAA-coated MNCs
MNCs were dispersed in the DI water at concentrations ranging from 200 μg/mL to 2 mg/mL, commercial Fe3O4 fluid (400 μg/mL), and DI water were exposed to an AMF for 60 min. As shown in , the MNC fluids of all used started heating up within 5 min. Furthermore, the temperature curves were MNC concentration-dependent, and the temperature stabilised after 40–45 min of exposure to the AMF. The Fe3O4 fluid (400 μg/mL) had a lower temperature compared to the MNCs at the same concentration. In addition, the temperature of DI water under AMF for 60 min stabilised at 28°C, indicating that AMF alone cannot raise the temperature of a watery fluid without magnetic nanoparticles. All MNC fluids with a concentration above 200 μg/mL reached a temperature above 42°C, which is appropriate for effective hyperthermia cancer treatment.
Cellular uptake and biocompatibility of MNCs
To investigate whether MNCs can be endocytosed by cancer and normal cells, we explored their intracellular distribution. Cells were incubated with MNCs for 6 and 24 h, respectively. CLSM clearly revealed the cytoplasmic distribution of the MNCs (). Similarly, TEM indicated that MNCs were distributed throughout the cytoplasm and around the nuclei envelope in both tumour and BEAS-2B cells ( and ). These results demonstrated that MNCs can be endocytosed by cells.
Figure 3. (A) CLSM image of CNE-2 cells (upper panels) and NCI-H460 cells (lower panels) incubated with MNCs for 6 h. MNCs were labelled by Rh-123 (red fluorescent signal) and nuclei were counterstained by Hoechst 33342 dye (blue fluorescent signal, scale bar = 10 µm) (B) TEM images of CNE-2 cells incubated with MNCs (100 μg/mL) for 24 h. (C) TEM images of BEAS-2B cells incubated with MNCs (100 μg/mL) for 24 h. (D) CNE-2 cell viability analysis of cells at various time points (24, 48, and 72 h) after incubation of cells with MNCs at various concentrations (400, 800, 1200, and 1600 μg/mL). Error bars represent standard deviations (n = 3). (E) NCI-H460 cell viability analysis of cells at various time points (24, 48, and 72 h) after incubation of cells with MNCs at various concentrations (400, 800, 1200, and 1600 μg/mL). Error bars represent standard deviations (n = 3). (F) BEAS-2B cell viability analysis of cells at various time points (24, 48, and 72 h) after incubation of cells with MNCs at various concentrations (400, 800, 1200, and 1600 μg/mL). Error bars represent standard deviations (n = 3).
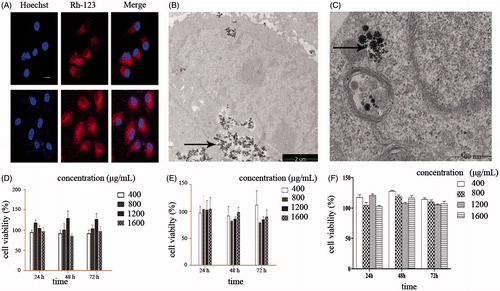
Cancer cells (CNE-2 and NCI-H460) and normal cells (BEAS-2B) were incubated with MNC fluids with various concentrations ranging from 400 μg/mL to 1600 μg/mL for specific periods of time (24, 48, and 72 h). Cell viability was assessed by the MTT assay. As shown in , and ), the cell viability for all three incubation periods and all MNC fluid concentrations was above 75% in all the cell lines, indicating that the MNCs did not induce cytotoxicity in cancer and normal cells and thus are biocompatible in all concentrations used. A concentration of 400 μg/mL was used in further experiments.
Cell cycle and apoptosis analysis
The CNE-2 and NCI-H460 cells were subjected to different treatments and subsequently stained with Annexin V-EGFP and PI; the apoptosis rate for both cell lines was analysed by flow cytometry (). For CNE-2 cells, the total apoptotic rate in the R, NP + R, NP + AMF, and R + NP + AMF groups was 12.91%, 13.85%, 19.04% and 28.94% respectively, compared to 3.43% and 6.01% of the Ctrl group and the NP group. The R + NP + AMF group exhibited the highest apoptotic rate (28.94%). Similar results were observed for the NCI-H460 cells. Flow cytometry was used to measure the cellular DNA content and for cell cycle analysis for each group. The NP + AMF and R + NP + AMF groups were dominated by S phase cells (p < 0.05), while the cells of the R group were predominantly in G2/M phase ().
Figure 4. Apoptosis rate (including early apoptosis and late apoptosis) assessed at 24 h after various treatments. After staining with Annexin V-EGFP and PI, the cells were counted by flow cytometry. Cells were grouped in Ctrl, NP, R, NP + AMF, and R + NP + AMF according to the different treatments. Ctrl, control; NP, MNCs alone; R, radiation; NP + R, cells incubated with MNCs were exposed to irradiation; NP + AMF, cells incubated with MNCs were subjected to AMF; R + NP + AMF, cells incubated with MNCs were irradiated and subsequently subjected to AMF.
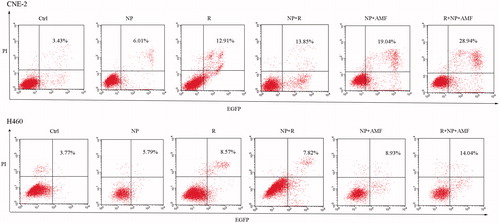
Figure 5. Cell cycle analysis of CNE-2 and NCI-H460 cells after different treatments. (A) DNA content of CNE-2 cells (upper panels) and NCI-H460 cells (lower panels) measured by flow cytometry after 24 h of treatment. (B) Bar graphs showing the percentages for cells in the S phase (upper panels) and G2/M phase (lower panels). Experiments were repeated three times. *p < 0.05.
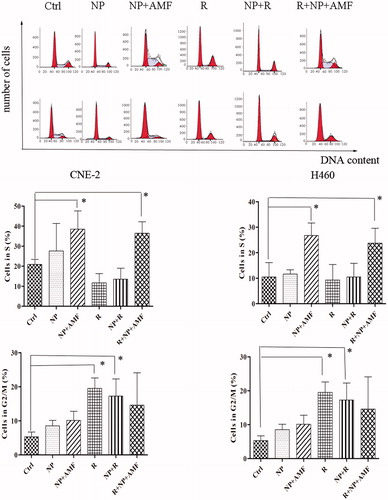
In vitro effect of MNCs under an AMF on radiosensitivity and DNA repair
A clone formation assay was performed to investigate the radiosensitisation effect of MNCs under an AMF on CNE-2 and NCI-H460 cells. The cell survival curves, generated according to the single-hit multi target model, are shown in , and the main parameters of cell survival curves are given in . With increasing radiation doses (0, 2, 4, 6, and 8 Gy), the SF of all cells reduced, with the SF of the R + NP + AMF group being lower than that of the R and NP + R groups. In addition, the survival curve parameters of the R + NP + AMF group were smaller than those of the R group, and the sensitivity enhancement ratio (SER) was 1.35 for CNE-2 and 1.44 for H460. These data indicate that MNCs under an AMF can radiosensitise both CNE-2 cells and NCI-H460 cells.
Figure 6. (A) In vitro cell survival curves derived from a colony formation assay of irradiated CNE-2 and NCI-H460 cells. The cells were exposed to an alternating magnetic field (AMF) for 1 h and radiated with different doses of X-rays. Data are shown as the mean ± SD from three independent experiments. (B) Micrographs of γ-H2AX foci at various time points after radiation. CNE-2 cells and NCI-H460 cells were irradiated (2 Gy) and then immunostained with anti-γ-H2AX primary antibody and DyLight 549-labelled secondary antibody. Hoechst 33342 was used to counterstain the nuclei. (C) Quantification of the γ-H2AX foci in each cell. More than 100 cells were counted for each time point. Error bars represent standard deviations, and * represents a significant difference compared with the R group (p < 0.05). (D) Tumour size at different time points after radiation and/or MNCs under an AMF indicating antitumour activity of the different treatments in NCI-H460 mouse xenograft models. (E) Prussian blue staining of PAA-Fe3O4 in resected tumour site.
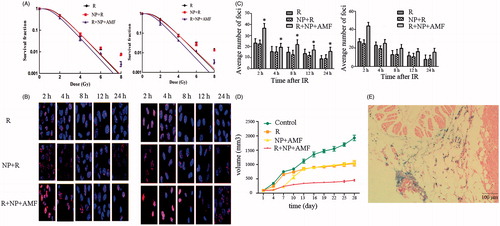
Table 1. The main radiosensitisation parameters of two cell lines after irradiation according to SF = 1 − (1 − e−D/D0)N.
To explore the effects of MNCs under an AMF on DSBs induced by ionising radiation we monitored the number of γ-H2AX foci in CNE-2 and NCI-H460 cells at different time points after treatment with 2 Gy of irradiation. As depicted in and ), average numbers of foci decreased as time prolonged after irradiation. In addition, the average number of γ-H2AX foci in the R + NP + AMF group was significantly higher than that in the R group at each time point. The number of γ-H2AX foci in the R group was similar to that in the NP + R group at each time point (p > 0.05). These results suggest that MNCs under an AMF has a radiosensitisation effect and effectively inhibits cellular DNA repair mechanisms.
The anti-tumour and radiosensitisation effect under an AMF in vivo
We used BALB/c nude mice to assess the effect of the different treatments on tumour growth in vivo. The results are shown in . Between day 4 and day 10 after treatment, tumour growth was significantly reduced in the H group compared to the R group (p < 0.05). However, after 13 days the two groups showed no significant differences (p > 0.05), with both groups exhibiting a similar level of tumour growth inhibition rate. The R and NP + AMF group inhibited tumour growth 46% and 49%, respectively, while combined treatment with R + NP + AMF inhibited tumour growth 80%. MNCs under an AMF combined with radiation thus had a better therapeutic effect in vivo than either one of the two treatments alone, suggesting a complementary and/or synergistic effect of both treatments.
Histological studies
The tumour section was observed under a light microscope. H&E and Prussian blue staining showed that many blue spots (i.e. Fe3+) could be observed in the xenografted tumour region (), indicating that PAA-Fe3O4 nanoparticles were distributed in the tumour site. Furthermore, our results suggested that PAA-Fe3O4 can be retained in the tumour for at least 7 days.
Western blot analysis
To investigate the putative mechanism of MNCs under an AMF radiosensitisation, we examined the expression of Hsp70 and caspase-3 proteins in the different treatment groups by using Western blot analysis. As shown in , the expression of both caspase-3 and Hsp70 was significantly higher in the R, NP + AMF, and R + NP + AMF groups than in the Ctrl group, indicating that all three treatments induced expression of these proteins (p < 0.05).
Discussion
Magnetic hyperthermia, a therapy in which magnetic particles or MNCs are used for localised heating of tumours, offers a promising approach to cancer treatment and has gained increasing interest in recent decades because it overcomes various limitations of classic hyperthermia such as low heat penetration in tumour tissues and cytotoxicity in healthy tissues by excessive heating. Surface modification of the nanoparticles with PAA is often used to increase particle dispersion and stability in suspension. In this study we examined the heating properties and effect of PAA-coated MNCs under an AMF, and the effect of MNCs under an AMF combined with radiotherapy on tumour cell growth in vitro and in vivo.
As a hydrophilic polymer, PAA allows magnetic nanoparticles to perfectly disperse in water. Therefore, various studies assessed the usability of PAA-coated nanoparticles for hyperthermia. Surendra et al. investigated the hyperthermia effect of different sizes of PAA-coated CoFe2O4 particles, and found that at a concentration of 1.25 g/L, the temperature of PAA-CoFe2O4 could reach 48°C, 58°C, and 68°C with increases in the particle sizes of 6 nm, 10 nm, and 14 nm under 10 min of AMF [Citation36]. In addition, Piñeiro-Redondo studied the specific absorption rate of PAA-coated magnetic nanoparticles of an average particle size of ∼10 nm with changing concentration of magnetic nanoparticles or viscosity of the solvent. The results revealed that the specific absorption rate (SAR) decreased with increasing particle concentration and solvent viscosity [Citation37].
We showed that MNC fluids of various concentrations heated up within 5 min and that the heating rate (200 μg/mL) was 1.4°C/min, indicating that the PAA-coated MNCs effectively generate heat. More importantly, they showed a potent heating efficacy under an AMF in relative low concentrations, while the temperature of Fe3O4 obtained from Aladdin Reagents was much lower at the same concentrations, which is in agreement with previous findings that clustered magnetic nanoparticles have a much higher SAR than individual magnetic nanoparticles [Citation38–39]. CLSM and TEM analyses clearly showed that the MNCs were endocytosed by the cancer cells, indicating that local heating of tumours can be achieved. A previous study showed that magnetic nanoparticles can be passed to daughter cells during cell mitosis, allowing the MNCs to spread throughout the tumour tissue, which increases the efficacy and endurance of hyperthermia [Citation40].
The safety of biomaterials that are eligible for application in human beings is of utmost concern. A great number of studies have proven that iron oxide is non-toxic to human and mouse cells, and it has been approved for use in humans by the FDA. In our study, the MTT assay was performed to analyse the cytotoxicity of PAA-coated MNCs. Our results revealed that within the range of 400–1600 μg/mL, the MNCs showed no significant toxicity to human cells. In CNE-2 cells, nanoparticles at a concentration of 800 μg/mL exhibited the highest cell viability at 24 h, while cell viability reached approximately 130% at a concentration of 1200 μg/mL at both 48 and 72 h. In NCI-H460 cells, cell viability increased with increasing nanoparticle concentrations. Further analysis of the effect of different concentrations of MNCs on cell viability is required. The cell viability was all beyond 75% at 24, 48, and 72 h after treatment (grade 0–1 according to cytotoxicity gradation), which meets the requirements of the standard of a safe and biocompatible material [Citation41].
PAA-coated MNCs could be endocytosed by both normal and cancer cells, and were located in the cytoplasm. In in vivo studies, the MNCs were retained in the tumour tissue after 7 days of nanoparticle injection into the xenograft. Nanoparticles that have reached the perivascular space are not cleared and they accumulate in the tumour tissue, which is called the “enhanced permeation and retention” effect. This is the basis of passive targeting [Citation42]. However, further studies of active specific targeting for cancer cells should be conducted.
It has been reported that magnetic particles can radiosensitise cells at both kilovoltage and megavoltage levels because the nanopartcicles have lower X-ray absorption. Khoei investigated the radiosensitisation effect of amino-group dextran coating iron oxide nanoparticles at megavoltage, demonstrating that its dose enhancer factor (DEF) is about 1.2 with the dose range of 1–6 Gy [Citation43]. Klein et al. [Citation54] found that superparamagnetic iron oxide nanoparticles could be a radiosensitiser via enhanced reactive oxygen species formation. In our study, PAA-coated Fe3O4 similarly exhibited very slight radiosensitisation effects with a sensitivity enhancement ratio of 1.04 for CNE-2 and of 1.06 for NCI-H460, while MNCs under an AMF showed a more potent radiosensitisation effect, thus reducing the required radiation dose and resulting in a better therapeutic effect.
Magnetic hyperthermia combined with radiotherapy has been shown to be highly effective for the treatment of cancer. In an in vivo study, Hoopes et al. studied the safety and efficacy of iron oxide nanoparticle hyperthermia and external beam radiation in a murine breast cancer model, demonstrating that iron oxide hyperthermia is more effective than non-nanoparticle tumour heating techniques [Citation44]. In clinical trials, Klaus et al. used magnetic hyperthermia combined with radiotherapy to treat glioblastoma multiforme patients, and found that the combination therapy more effectively shrank tumours than either one of the therapies alone [Citation45]. Using flow cytometry, we found that MNCs under an AMF displayed a slightly better killing effect than radiotherapy alone (at a dose of 4 Gy), while the group exposed to a combination of both treatments showed the highest apoptosis rate, which is consistent with the results reported by Lin et al. [Citation46]. The SER for CNE-2 and NCI-H460 cells was 1.35 and 1.44, respectively, confirming their radiosensitisation effect.
Exposure to radiation can cause lethal DSBs in cells which arise from single-strand breaks or base damage [Citation47]. The phosphorylated histone H2AX protein (γ-H2AX), which is expressed after the induction of DNA DSBs, with an expression peak at 30 min after DSBs induction, is often used as a sensitive biomarker for the assessment of radiosensitivity [Citation48]. In our research, average foci numbers of the R + NP + AMF group significantly exceeded those of the R group (p < 0.05). This indicated that MNCs under an AMF, but not MNCs alone, efficiently radiosensitised cells, suggesting that MNCs under an AMF may inhibit the repair of DSBs. The results of the cell cycle analysis after different treatments showed that the cell cycles were arrested at the S phase after 24 h of MNCs under an AMF or the combination of both treatments. Radiation alone prolonged the G2/M phase, while cells treated with MNCs alone had cell cycle distribution similar to the control group. To date, it is not known how exactly hyperthermia affects the cell cycle distribution. However, our results confirmed that cells in the S phase are more sensitive to hyperthermia.
Heat shock proteins (HSPs) are highly conserved molecular chaperones that catalyse protein folding and assist in the synthesis, processing, and transportation of proteins. When cells are being exposed to mild stress, such as heat, irradiation, and reactive oxide species, heat shock proteins will be induced in order to reduce the cellular damage [Citation49–51]. It has been demonstrated that HSP is a conserved protein that is expressed in physical conditions. In tumour cells, the expression of HSPs is also up-regulated. Hyperthermia is known to induce expression of HSPs such as Hsp27, Hsp70, and Hsp90 that participate in the destruction of tumour cells through complex, yet not fully revealed mechanisms. Ito et al. observed that hyperthermia promoted an anti-tumour immune response by the activation of Hsp70 [Citation52]. In addition, hyperthermia can induce apoptosis by repressing the anti-apoptosis factors Bcl-2, Bcl-xL, and Mcl-1, which activates downstream cytochrome c, and eventually upregulates caspase-3 [Citation53]. Therefore, we examined caspase-3 and Hsp70 expression after different treatments. Our finding that both proteins were upregulated by MNCs under an AMF, radiation, and the combination of both, is in agreement with previous studies, and suggests that MNCs under an AMF radiosensitise cells by inducing apoptosis.
Conclusions
The PAA-coated MNCs used in this study were synthesised using the solvothermal reduction method; these were highly dispersible in an aqueous suspension. We showed that the MNC fluids were biocompatible and that MNCs under an AMF were able to radiosensitise CNE-2 and NCI-H460 human cancer in vitro at relatively low concentrations of 400 μg/mL. Furthermore, we demonstrated that MNCs under an AMF combined with radiotherapy effectively inhibited tumour growth in vivo. We hypothesised that the putative mechanism of radiosensitisation by the MNCs involves the inhibition of the DNA repair mechanism and the induction of apoptosis by up-regulating caspase-3 expression. Finally, this study revealed the potent hyperthermia efficiency of PAA-coated MNCs under an AMF, and provided an experimental basis for the use of both hyperthermia and radiotherapy to strengthen their therapeutic effect.
Acknowledgements
We would like to thank Weihong Chen, Jinghua Ren, and Honglin Jin of the Cancer Centre, Union Hospital, Huazhong University of Science and Technology, for advice in the experiments.
Declaration of interest
This work was supported by a grant from the National Natural Science Foundation of China (Grant No. 81172121). There are no conflicts of interest. The authors alone are responsible for the content and the writing of the paper.
References
- Hildebrandt B, Wust P, Ahlers O, Dieing A, Sreenivasa G, Kerner T, et al. The cellular and molecular basis of hyperthermia. Crit Rev Oncol Hematol 2002;43:33–56
- Emami B, Song CW. Physiological mechanisms in hyperthermia: A review. Int J Radiat Oncol Biol Phys 1984;10:289–95
- Nikfarjam M, Muralidharan V, Christophi C. Mechanisms of focal heat destruction of liver tumors. J Surg Res 2005;127:208–23
- Tasci TO, Vargel I, Arat A, Guzel E, Korkusuz P, Atalar E. Focused RF hyperthermia using magnetic fluids. Med Phys 2009;36:1906–12
- Le Renard PE, Jordan O, Faes A, Petri-Fink A, Hofmann H, Rüfenacht D, et al. The in vivo performance of magnetic particle-loaded injectable, in situ gelling, carriers for the delivery of local hyperthermia. Biomaterials 2010;31:691–705
- Kang M, Liu WQ, Qin YT, Wei ZX, Wang RS. Long-term efficacy of microwave hyperthermia combined with chemoradiotherapy in treatment of nasopharyngeal carcinoma with cervical lymph node metastases. Asian Pac J Cancer Prev 2013;14:7395–400
- Tamarov KP, Osminkina LA, Zimovyev SV, Maximova KA, Kargina JV, Gongalsky MB, et al. Radio frequency radiation-induced hyperthermia using Si nanoparticle-based sensitizers for mild cancer therapy. Sci Rep 2014;4:7034
- Jordan A, Wust P, Fähling H, John W, Hinz A, Felix R. Inductive heating of ferrimagnetic particles and magnetic fluids: Physical evaluation of their potential for hyperthermia. Int J Hyperthermia 1993;9:51–68
- Jordan A. Thermotherapy and nanomedicine: Between vision and reality. In: Baronzio GF, Hager ED, editors. Hyperthermia in Cancer Treatment: A Primer. New York: Springer; 2006. pp 60–63
- Kampinga HH. Cell biological effects of hyperthermia alone or combined with radiation or drugs: A short introduction to newcomers in the field. Int J Hyperthermia 2006;22:191–6
- Maier-Hauff K, Rothe R, Scholz R, Gneveckow U, Wust P, Thiesen B, et al. Intracranial thermotherapy using magnetic nanoparticles combined with external beam radiotherapy: Results of a feasibility study on patients with glioblastoma multiforme. J Neurooncol 2007;81:53–60
- Diagaradijane P, Shetty A, Wang J, Elliot A, Schwartz J, Shentu S, et al. Gold nanoshell mediated hyperthermia enhances the efficacy of radiation therapy. Proc SPIE 2008; 6865:68650N
- Attaluri A1, Kandala SK, Wabler M, Zhou H, Cornejo C, Armour M, et al. Magnetic nanoparticle hyperthermia enhances radiation therapy: A study in mouse models of human prostate cancer. Int J Hyperthermia 2015;26:1–16
- Franckena M. Review of radiotherapy and hyperthermia in primary cervical cancer. Int J Hyperthermia 2012;28:543–8
- Lutgens L, van der Zee J, Pijls-Johannesma M, De Haas-Kock DF, Buijsen J, Mastrigt GA, et al. Combined use of hyperthermia and radiation therapy for treating locally advanced cervical carcinoma. Cochrane Database Syst Rev 2010;1:CD006377
- Suit HD, Gerweck LE. Potential for hyperthermia and radiation therapy. Cancer Res 1979;39:2290–98
- Mladenov E, Magin S, Soni A, Iliakis G. DNA double-strand break repair as determinant of cellular radiosensitivity to killing and target in radiation therapy. Front Oncol 2013;3:113
- Matsumura Y, Maeda H. A new concept for macromolecular therapeutics in cancer chemotherapy: Mechanism of tumoritropic accumulation of proteins and the antitumor agent smancs. Cancer Res 1986;46:6387–92
- Grumezescu AM, Gestal MC, Holban AM, Grumezescu V, Vasile BS, Mogoantă L, et al. Biocompatible Fe3O4 increases the efficacy of amoxicillin delivery against Gram-positive and Gram-negative bacteria. Molecules 2014;19:5013–27
- Tseng TC, Hsu SH. Substrate-mediated nanoparticle/gene delivery to MSC spheroids and their applications in peripheral nerve regeneration. Biomaterials 2014;35:2630–41
- Sriraman SK, Aryasomayajula B, Torchilin VP. Barriers to drug delivery in solid tumors. Tissue barriers 2014;2:e29528
- Lu W, Ling M, Jia M, Huang P, Li C, Yan B. Facile synthesis and characterization of polyethylenimine-coated Fe3O4 superparamagnetic nanoparticles for cancer cell separation. Mol Med Rep 2014;9:1080–4
- Zhang Y, Li D, Yu M, Ma W, Guo J, Wang C. Fe3O4/PVIM-Ni2+ magnetic composite microspheres for highly specific separation of histidine-richproteins. ACS Appl Mater Interfaces 2014;6:8836–44
- Kobayashi T. Cancer hyperthermia using magnetic nanoparticles. Biotechnol J 2011;6:1342–7
- Gass J, Poddar P, Almand J, Srinath S, Srikanth H, et al. Superparamagnetic polymer nanocomposites with uniform Fe3O4 nanoparticle dispersions. Adv Funct Mater 2006;16:71–75
- Yu WW, Falkner JC, Yavuz CT, Colvin VL. Synthesis of monodisperse iron oxide nanocrystals by thermal decomposition of iron carboxylate salts. Chem Commun 2004;2306–7
- Xuan S, Wang YXJ, Yu JC, Leung KCF, et al. Tuning the grain size and particle size of superparamagnetic Fe3O4 microparticles. Chem Mater 2009;21:5079–87
- Mukhopadhyay A, Joshi N, Chattopadhyay K, De G. A facile synthesis of PEG-coated magnetite (Fe3O4) nanoparticles and their prevention of the reduction of cytochrome c. ACS Appl Mater Interfaces 2012;4:142–9
- Huining L, Yi Z, Dihong T, Yifeng P, Man X, Ting Y, et al. Inhibition of choriocarcinoma by Fe3O4-dextran-anti-β-human chorionic gonadotropin nanoparticles containing antisense oligodeoxynucleotide of heparanase. Int J Nanomed 2013;8:4371–8
- Cai H, An X, Cui J, Li J, Wen S, Li K, et al. Facile hydrothermal synthesis and surface functionalization of polyethylenimine-coated iron oxide nanoparticles for biomedical applications. Anal Chem 2013;85:11585–92
- Hayashi K, Nakamura M, Sakamoto W, Yogo T, Miki H, Ozaki S, et al. Superparamagnetic nanoparticle clusters for cancer theranostics combining magnetic resonance imaging and hyperthermia treatment. Theranostics 2013;3:366–76
- Xu Y, Lin Y, Zhuang L, Lin J, Lv J, Huang Q, et al. Bleomycin loaded magnetite nanoparticles functionalized by polyacrylic acid as a new antitumoral drug delivery system. Biomed Res Int 2013;2013:462589
- Xu F, Cheng C, Chen DX, Gu H. Magnetite nanocrystal clusters with ultra-high sensitivity in magnetic resonance imaging. Chem Phys Chem 2012;13:336–41
- Ke JH, Lin JJ, Carey JR, Chen JS, Chen CY, Wang LF. A specific tumor-targeting magnetofluorescent nanoprobe for dual-modality. Biomaterials 2010;31:1707–15
- Xuan S, Wang F, Lai JM, Sham KW, Wang YX, Lee SF, et al. Synthesis of biocompatible, mesoporous Fe3O4 nano/microspheres with large surface area for magnetic resonance imaging and therapeutic applications. ACS Appl Mater Interfaces 2011;3:237–40
- Surendra MK, Annapoorani S, Ansar EB, Varma PRH, Rao MSR. Magnetic hyperthermia studies on water-soluble polyacrylic acid-coated cobalt ferrite nanoparticles. J Nanopart Res 2014;16:2773
- Piñeiro-Redondo Y, Bañobre-López M, Pardiñas-Blanco I, Goya G, López-Quintela MA, Rivas R. The influence of colloidal parameters on the specific power absorption of PAA-coated magnetite nanoparticles. Nanoscale Res Lett 2011;6:383
- Jeyadevan B. Present status and prospects of magnetite nanoparticles-based hyperthermia. J Ceram Soc Jpn 2010;118:391–401
- Lartigue L, Hugounenq P, Alloyeau D, Clarke SP, Lévy M, Bacri JC, et al. Cooperative organization in iron oxide multi-core nanoparticles potentiates their efficiency as heating mediators and MRI contrast agents. ACS Nano 2012;6:10935–49
- Qiu P, Jensen C, Charity N, Towner R, Mao C. Oil phase evaporation-induced self-assembly of hydrophobic nanoparticles into spherical clusters with controlled surface chemistry in an oil-in-water dispersion and comparison of behaviors of individual and clustered iron oxide nanoparticles. J Am Chem Soc 2010;132:17724–32
- Stella B, Arpicco S, Peracchia MT, Desmaële D, Hoebeke J, Renoir M, et al. Design of folic acid-conjugated nanoparticles for drug targeting. J Pharm Sci 2000;89:1452–64
- Jain RK. Transport of molecules across tumor vasculature. Cancer Metast Rev 1987;6:559–93
- Khoei S, Mahdavi SR, Fakhimikabir H, Shakeri-Zadeh A, Hashemian A. The role of iron oxide nanoparticles in the radiosensitization of human prostate carcinoma cell line DU145 at megavoltage radiation energies. Int J Radiat Biol 2014;90:351–6
- Hoopes PJ, Strawbridge RR, Gibson UJ, Zeng Q, Pierce ZE, Savellano M, et al. Intratumoral iron oxide nanoparticle hyperthermia and radiation cancer treatment. Proc SPIE 2007;6440:64400K
- Maier-Hauff K, Rothe R, Scholz R, Gneveckow U, Wust P, Thiesen B, et al. Intracranial thermotherapy using magnetic nanoparticles combined with external beam radiotherapy: results of a feasibility study on patients with glioblastoma multiforme. J Neurooncol 2007;81:53–60
- Lin M, Zhang D, Huang J, Zhang J, Xiao W, Yu H, et al. The anti-hepatoma effect of nanosized Mn-Zn ferrite magnetic fluid hyperthermia associated with radiation in vitro and in vivo. Nanotechnology 2013;24:255101
- Jeggo PA, Geuting V, Löbrich M. The role of homologous recombination in radiation-induced double-strand break repair. Radiother Oncol 2011;101:7–12
- Rogakou EP, Boon C, Redon C, Bonner WM. Megabase chromatin domains involved in DNA double-strand breaks in vivo. J Cell Biol 1999;146:905–16
- Jäättelä M. Escaping cell death: survival proteins in cancer. Exp Cell Res 1999;248:30–43
- Mayer MP, Bukau B. Hsp70 chaperones: cellular functions and molecular mechanism. Cell Mol Life Sci 2005;62:670–84
- Dakappagari N, Neely L, Tangri S, Lundgren K, Hipolito L, Estrellado A, et al. An investigation into the potential use of serum Hsp70 as a novel tumour biomarker for Hsp90 inhibitors. Biomarkers 2010;15:31–8
- Ito A, Shinkai M, Honda H, Yoshikawa K, Saga S, Wakabayashi T, et al. Heat shock protein 70 expression induces antitumor immunity during intracellular hyperthermia using magnetite nanoparticles. Cancer Immunol Immunother 2003;52:80–8
- Cui ZG, Piao JL, RehmancMU, Ogawa R, Li P, Zhao QL, et al. Molecular mechanisms of hyperthermia-induced apoptosis enhanced by withaferin A. Eur J Pharmacol 2014;723:99–107
- Klein S, Sommer A, Distel LV, Neuhuber W, Kryschi C, et al. Superparamagnetic iron oxide nanoparticles as radiosensitizer via enhanced reactive oxygen species formation. Biochem Biophys Res Commun 2012;425:393–7