Abstract
Chronic non-healing wound infections require long duration antibiotic therapy, and are associated with significant morbidity and health-care costs. Novel approaches for efficient, readily-translatable targeted and localised antimicrobial delivery are needed. The objectives of this study were to 1) develop low temperature-sensitive liposomes (LTSLs) containing an antimicrobial agent (ciprofloxacin) for induced release at mild hyperthermia (∼42 °C), 2) characterise in vitro ciprofloxacin release, and efficacy against Staphylococcus aureus plankton and biofilms, and 3) determine the feasibility of localised ciprofloxacin delivery in combination with MR-HIFU hyperthermia in a rat model. LTSLs were loaded actively with ciprofloxacin and their efficacy was determined using a disc diffusion method, MBEC biofilm device, and scanning electron microscopy (SEM). Ciprofloxacin release from LTSLs was assessed in a physiological buffer by fluorescence spectroscopy, and in vivo in a rat model using MR-HIFU. Results indicated that < 5% ciprofloxacin was released from the LTSL at body temperature (37 °C), while >95% was released at 42 °C. Precise hyperthermia exposures in the thigh of rats using MR-HIFU during intravenous (i.v.) administration of the LTSLs resulted in a four fold greater local concentration of ciprofloxacin compared to controls (free ciprofloxacin + MR-HIFU or LTSL alone). The biodistribution of ciprofloxacin in unheated tissues was fairly similar between treatment groups. Triggered release at 42 °C from LTSL achieved significantly greater S. aureus killing and induced membrane deformation and changes in biofilm matrix compared to free ciprofloxacin or LTSL at 37 °C. This technique has potential as a method to deliver high concentration antimicrobials to chronic wounds.
Introduction
Chronic non-healing wound infections have been documented for many decades, and can be associated with significant morbidity and costly complications. The most common forms of chronic wounds are associated with peripheral vascular diseases, bone infection, venous stasis, and diabetes mellitus [Citation1]. Despite significant progress, successful therapeutic outcomes remain a challenge due to intrinsic virulence of infecting organisms [Citation1], substantial colonisation without inflammation [Citation2], biofilm formation [Citation3], and inability of topical or systemically administered antimicrobials to reach the site of infection and clear bacteria [Citation4]. This results in both systemic and local infection to deeper tissues (e.g. bones) [Citation5], thereby requiring long treatment (generally >6 weeks), resection of tissues, and emergence of drug resistance [Citation6–8]. Although healing with antimicrobials alone is challenging but possible, therapeutic failure and adverse drug reactions due to prolonged use and relapse are still common [Citation9]. Overcoming this limitation and capturing the full therapeutic potential of antimicrobial treatment of non-healing wounds require improved ability to control and selectively release drugs within infected wounds at high concentration and the ability to non-invasively control drug release and bactericidal effects on demand without the need of surgical manipulation and debridement.
In previous studies it has been shown in rat models that local treatment of contaminated wounds with stealth liposome-encapsulated antimicrobials may offer advantages over free antibiotics, including an increase in efficacy, ease of administration, and safety [Citation10,Citation11]. Such sustained release is highly promising, but stealth liposomes are designed to release drug slowly in wounds, and their ability to achieve drug delivery in a bolus fashion at the infected site is limited. This may impact bacterial clearance in wounds that are infested with biofilms because their susceptibility towards antibiotics and biocides are 100- to 1000-fold less than equivalent populations of planktonic bacteria, and require large dosage for efficient bacterial clearance [Citation12]. In contrast to stealth nanocarriers which release their drug over weeks, in this study we developed antimicrobial-loaded low temperature-sensitive liposomes (LTSLs), which contain a lysolecithin lipid that allows for rapid release of encapsulated content upon being heated to mild hyperthermic temperatures (40–42 °C) [Citation13]. The motivation for this idea stems from previous work by Yatvin et al., where an inhibition in the synthesis of protein by Escherichia coli B/r was noted between 42–46 °C upon treatment with temperature-sensitive liposomes composed of dipalmitoylphosphatidylcholine (DPPC) and distearoyl phosphatidylcholine (DSPC) (3:1 molar ratio) in vitro [Citation14]. Based on this, we hypothesise that utilising LTSLs will permit ‘on-demand’ controlled bolus release of antimicrobial delivery by simply increasing the temperature for a brief time around the wound after the LTSL were applied either locally or systemically. Additionally, as demonstrated by us and others, we also hypothesise that mild hyperthermia during LTSL delivery will decrease resistance in vascular beds, relieve interstitial pressure, increase vascular permeability, and improve drug penetration; all of which can establish a high intravascular drug concentration leading to the improved drug coverage in heated regions of the wound [Citation15–19]. More specifically, as shown by de Smet et al., hyperthermia delivery using magnetic resonance imaging (MRI)-guided high-intensity focused ultrasound (MR-HIFU) can increase LTSL drug delivery beyond the amount observed directly after intravascular release triggered by hyperthermia in poorly perfused regions, or regions with few blood vessels [Citation20]. Thus, this approach could be highly relevant for biofilm therapy as they are poorly perfused, and demonstrate poor antimicrobial penetration [Citation3,Citation21].
As an initial step we characterised the efficacy of LTSLs in combination with mild hyperthermia in an in vitro Staphylococcus aureus model. S. aureus is the most common Gram positive aerophilic cocci bacterial isolate from chronic wounds [Citation22], and expresses a number of potential virulence factors and surface proteins which promote its adherence to the damaged tissue, and formation of biofilm that limits drug delivery [Citation23]. As a model antimicrobial agent, we chose ciprofloxacin. Ciprofloxacin is a second generation fluoroquinolone antimicrobial drug with known bactericidal action against gram positive and negative bacteria such as Escherichia coli, Haemophilus influenzae, Klebsiella pneumoniae, Legionella pneumophila, Moraxella catarrhalis, Staphylococcus aureus, Streptococcus pneumoniae, and Staphylococcus epidermidis [Citation24]. Finally, we tested the ability of LTSLs to achieve bolus antimicrobial drug delivery in a rat model in combination with mild hyperthermia generated using MR-HIFU. Data from our study suggest that the formulation approach presented in this study has the potential to meet our long-term objective of developing a clinically relevant drug delivery strategy for management of chronic wound infections.
Materials and methods
Chemicals
1,2-dipalmitoyl-sn-glycerol-3-phosphocholine (DPPC), 1-stearoyl-2-hydroxyl-sn-glycero-3-phosphocholine (MSPC), N-(Carbonyl-methoxypolyethyleneglylcol-2000)-1,2-distearoyl-sn-glycerol-3-phosphoethanolamine sodium salt (DSPE-MPEG 2000) were obtained from Corden Pharma (Boulder, CO, USA). Ciprofloxacin HCl was obtained from LC laboratory (St Louis, MO, USA). Trypticase soy powder and broth were obtained from Becton Dickinson (Sparks, MD). Staphylococcus aureus (ATCC 29213) was obtained from the Oklahoma Animal Disease Diagnostic Laboratory (Stillwater, OK).
Synthesis of ciprofloxacin-loaded LTSL
LTSLs were prepared by hydration of a phospholipid film followed by extrusion through a polycarbonate membrane filter [Citation25–27]. Briefly, the phospholipids [DPPC:MSPC:DSPE-PEG] were dissolved in a minimum volume of chloroform at a molar ratio of 85.3:9.7:5.0. The organic solvent was evaporated to dryness in a rotary evaporator and the resulting thin film of phospholipids was hydrated using 300 mM ammonium sulphate at pH 2.5. The hydrated lipids were extruded five times through two stacked polycarbonate filters of 100 nm pore size to yield bland liposomes (LTSLs). Encapsulation of ciprofloxacin into LTSLs (2 mg of ciprofloxacin per 100 mg of lipids) was carried out actively using a pH gradient method described by Mayer et al. [Citation28]. Unencapsulated ciprofloxacin in the LTSL solution was removed using a PD-10 size exclusion column (GE Healthcare Bio-Sciences, Pittsburgh, USA).
LTSL size and ciprofloxacin loading analysis
LTSL were characterised for size using a dynamic light scattering (DLS) instrument (Zetapals, Brookhaven Instruments, Holtsville, NY). Briefly, 10–20 µL of LTSLs were added to 2 mL of water in a cuvette, and DLS measurements were taken (n = 3). Encapsulation efficiency was measured using a total phosphorus assay as described previously by Oh et al. [Citation29], by computing the ratio of drug:phosphorus in pre- and column-purified samples as given below.
Thermoscan of ciprofloxacin release from LTSLs
Stability at body temperature (37 °C) was assessed by measuring release of encapsulated ciprofloxacin from LTSLs as a function of temperature (25–42 °C) in vitro in phosphate buffered saline (PBS). For ciprofloxacin release study, samples (50 mg lipids/1 mg ciprofloxacin) were diluted 50-fold in phosphate buffered saline (PBS), and placed in a quartz cuvette equipped with a stopper and magnetic stirrer. A Cary Eclipse Fluorescence Spectrophotometer (Agilent Technologies, Santa Clara, CA) equipped with Multicell Holder and Temperature Controller was used to measure ciprofloxacin release from the LTSLs. Ciprofloxacin release was assessed by excitation at 320 nm and fluorescence emission monitored at 420 nm every 1 °C from 25–42 °C. Each temperature point was held for 3 min to ensure the temperature had fully equilibrated. From 39–42 °C fluorescence readings were taken every 0.2 °C and each point was equilibrated for 1 min.
Ciprofloxacin release kinetics
To measure ciprofloxacin release as a function of time at a constant temperature, kinetics experiments were performed. For fluorescence measurement, at the same volumes as described as described in 2.4, the samples were equilibrated to the desired temperature (25, 30, 35 and 37–42 °C) for 15 min. Baseline fluorescence measurements for each sample were taken at 25 °C and complete release was determined by heating the sample for 5 min at 45 °C. Drug release based on fluorescence quantification at a given time (t) under constant temperature exposure was determined using the equation below:
where Io represented the initial fluorescence intensity of LTSL suspension at 25 °C, and It is its intensity at time (t) at a predetermined temperature. Im represented the fluorescence intensity of completely released ciprofloxacin at 45°C. Data was obtained as percentage release of encapsulated ciprofloxacin at a given temperature.
In vitro efficacy assessment
S. aureus culture
S. aureus was grown overnight in trypticase soy broth (TSB), centrifuged, washed in PBS and serially diluted to achieve a final suspension containing 1.0 × 108 colony forming unit/mL.
Disc diffusion method
500 µL of LTSL were heated to 37 and 42 °C in separate 1.5 mL tubes under continuous monitoring of a thermocouple. Once the desired temperature was reached, LTSLs were stabilised for 10 min and then placed in a dialysis bag (molecular weight cut-off 3000 kDa), which was then submerged in a conical flask that contained 50 mL of sterile PBS maintained at room temperature. At a 24-h interval, 1 mL of dialysate containing the released ciprofloxacin was collected. Then the antimicrobial activity of released drug was evaluated using a disc diffusion method. Briefly, a Mueller-Hinton (MH) agar plate was inoculated with S. aureus (1 × 108 CFU/mL). Next, sterile paper discs of 5 mm in diameter containing 10 µL of dialysate were laid on the inoculated test organism. The Petri dishes were incubated at 37 °C for 24 h and antimicrobial activity of LTSL was determined by measuring the zone inhibition around the disc. The mean and standard deviation reported for each temperature point was tested in triplicate (n = 9).
Efficacy against biofilms
The efficacy of LTSL against biofilms was determined using a well-established MBEC 96-well plate system (Innovotech, Edmonton, Alberta, Canada) that allows for high throughput screening of test agents [Citation30]. Briefly, MBEC wells were filled with 150 µL of S. aureus culture (1.0 × 107 CFU mL-1), and then were incubated for 5 days in a humidified gyro-rotary shaker rotating at 150 rpm at 37 °C to establish a biofilm on the pegs located on the lid of the device. Biofilm growth was confirmed by scanning electron microscopy (see below). Upon confirmation, the pegs containing biofilm were transferred to a new plate containing 200 µL of free ciprofloxacin or LTSL (3–350 µM ciprofloxacin). To compare response with (40–42 °C) and without (37 °C) mild hyperthermia, one set of LTSL-treated cells was heated to 42 °C for 24 h in the incubator. The lid containing the pegs was then transferred to a 96-well plate containing 200 µL of PBS, and the biofilm was sonicated for 15 min to release the bacteria in PBS. Aliquots (50 μL) of the bacterial suspensions were serially diluted in 450 μL of PBS to a maximum of 105-fold dilution. Subsequently, the dilutions were read using a spectrophotometer at optical density of 650 nm. Wells with visual turbidity >0.1 were interpreted to have positive bacterial growth. Data were normalised to untreated control, and mean percent bacterial killing was computed (n = 4–6).
Scanning electron microscopy of S. aureus biofilms
Scanning electron microscopy (SEM) of biofilms was conducted following LTSL treatment as described above. Briefly, each peg was collected separately, fixed with 10% formaldehyde, washed with sodium cacodylate buffer, incubated for 1 h in 1% osmium tetraoxide in cacodylate buffer, serially dehydrated in increasing concentrations of ethanol (50, 70, 90, 95 and 100%), and dried in hexamethyldisilazane. Individual samples (biofilm alone, LTSL (37 and 42 °C) were mounted on microscopy stubs with tape and then coated with gold–palladium, and viewed under the SEM.
In vivo drug delivery study design: animal and experimental set-up
A pilot animal study evaluating the feasibility of achieving local tissue delivery of ciprofloxacin using LTSLs and mild hyperthermia was conducted. All animal procedures were approved and carried out under the guidelines of the University of Texas Southwestern Medical Center Institutional Animal Care and Use Committee. Sprague Dawley Rats (male, 500–600 g) were randomly assigned to two treatment groups (three rats per group): 1) i.v. administration of free ciprofloxacin (10 mg/kg) + MR-HIFU hyperthermia, and 2) i.v. administration of LTSL (10 mg/kg) + MR-HIFU hyperthermia. All animals were anaesthetised with a mixture of 2–3% isoflurane and 1–2 L/min of 100% oxygen. A pulse oximeter was attached to the animal’s paw to monitor heart rate and oxygen saturation, and a fibre-optic temperature probe was inserted into the rectum to monitor and record core temperature (T1, Neoptix, Quebec, Canada). Fur covering the animal’s thigh was removed using an electric trimmer and depilatory cream (Veet, Parsippany, NJ, USA) to enable transmission of ultrasound into the thigh muscle. Overview of the experimental set-up is shown in . The animal was placed in the decubitus position on the platform of a small animal MR-HIFU system (RK100, FUS Instruments, Toronto, Canada, ) on top of a warming tray that maintained its core temperature at 37 °C. The target region in the superficial thigh muscle was positioned over a window in the warming tray with a thin film, and ultrasound gel was applied between the skin of the thigh and the film for acoustic coupling. A circulating warming blanket was placed on top of the rat to assist in maintaining body temperature throughout the heating experiment. Further technical details regarding the construction, characterisation, and use of the small animal system for hyperthermia exposures is given in Senavirathna et al. [Citation26].
Figure 1. Experimental setup for the in vivo drug delivery experiments. (a) The animal was placed on its side on the MR-HIFU platform with one thigh in the path of a focused ultrasound beam. (b) A transverse MR image through the thigh shows the location of heating in the dashed circle. (c) A sagittal MR image along the path of the ultrasound beam shows the location of heating in a perpendicular plane. The relationship between both images is illustrated with the white dashed lines.
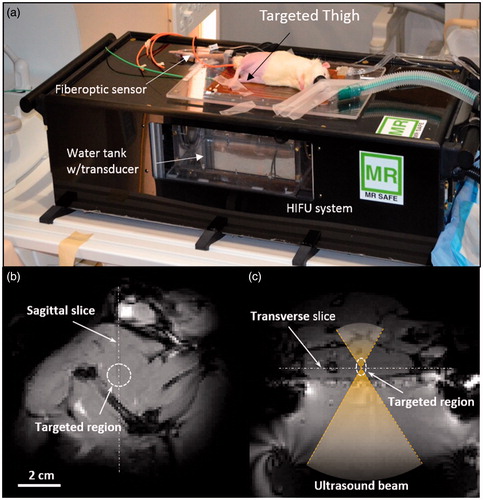
Image-guided MR-guided HIFU treatment
Once the animal was appropriately positioned on the MR-HIFU system the thigh muscle in one leg was heated using a focused ultrasound transducer equipped with a 3D-printed sector vortex lens, driven at 3.39 MHz and a maximum electric power of 7 W. The target temperature within a region of the thigh measuring approximately 10 mm in diameter was 42 °C and the duration of hyperthermia was 30 min. T1-weighted survey scans (GRE sequence, TE = 5.1 ms, TR = 30 ms, 128 × 128 matrix, FOV = 12.8 × 12.8 cm, voxel size = 1.0 × 1.0 × 1.5 mm) were acquired for treatment planning and localisation of the ultrasound beam (). A preliminary test shot was performed to confirm proper localisation of heating, and to ensure the ultrasound beam was not hitting any bones that might impact the ability to achieve uniform heating. After confirmation, ultrasound energy was delivered during continuous gradient echo imaging and calculation of the temperature distribution in the muscle using the PRFS method for a period of 30 min of heating and an additional 10 min to monitor cooling. Control of heating was achieved by monitoring the temperature within a circular region of interest (ROI) (2.5-mm radius) on the transverse temperature maps and using these measurements as input to a feedback control algorithm to adjust the electrical power delivered to the transducer.
Sonication, drug administration and tissue harvesting
Once the average temperature in the circular region of interest reached 42 °C, LTSL was administered i.v. (10 mg/kg through the tail vein) at a rate of 0.02 mL/s using an automatic syringe pump (Medrad Spectris Solaris EP, Bayer HealthCare, Whippany, NJ) attached to the MR scanner. The duration of the infusion was approximately 5 min for all animals. Approximately 20 min after treatment (10 min continuous temperature monitoring to observe the tissue cooling and ∼10 min post-imaging), the rat was removed from the bore of the magnet and transferred to a procedural room, where it was exsanguinated using cardiac perfusion under deep anaesthesia in order to remove unabsorbed drugs in the vasculature. The heated and contralateral unheated muscles were harvested, along with tissue samples from liver, spleen, lung, heart, and kidney. Excised tissues were weighed, and snap frozen over liquid nitrogen for subsequent ciprofloxacin analysis.
Analysis of ciprofloxacin in tissues
Tissue samples (100 mg) were homogenised in 1.2 mL extraction medium (2% aqueous acetic acid/acetonitrile, 1:1 v/v) using a bead-vial homogeniser (Mini-Beadbeater-16, BiospecBartlesville, OK) at 3450 oscillations/min for 3 min in 2 mL polypropylene screw-cap micro vials (Biospec) using zirconia beads (1 mm diameter, Biospec). Homogenised tissue samples were kept at −78 °C before and after use. The sample lysate was transferred to 1.5 mL tubes and centrifuged to pellet cell debris at 14,000 g for 15 min. The supernatant was transferred to another 1.5 mL tube and centrifuged an additional 15 min. For ciprofloxacin detection, 500 μL of clarified supernatant was added to a 700 μL quartz cuvette, and fluorescence (ex: 320 nm, em: 420 nm) was measured using a SpectraMax M2 spectrophotometer (Molecular devices, Sunnyvale, CA).
Statistical analysis
Treatment groups (free ciprofloxacin, free ciprofloxacin(42 °C), LTSL, and LTSL(42 °C) were compared for differences in mean ciprofloxacin concentration using analysis of variance (ANOVA) followed by Tukey’s multiple comparison post hoc test. All analyses were performed using GraphPad Prism 6.0 (San Diego, CA). All p-values were two-sided, and a p-value less than 0.05 indicated statistical significance. Values are reported as mean ± SEM.
Results
LTSL size and encapsulation efficiency
The hydrodynamic diameter of LTSLs measured by DLS was 153 ± 1.1 nm (n = 3) with a polydispersity index of 0.122 (±0.028). Active loading of ciprofloxacin by transmembrane pH gradient yielded an encapsulation efficiency of >95% in LTSLs.
Thermoscan of ciprofloxacin release from LTSLs
Percentage ciprofloxacin release from LTSL in PBS by its fluorescence dequenching was minimal (<5%) at 25–39 °C (); was followed by a more gradual release at 40 °C (∼20%), and was rapid and complete (>95%) near the temperature giving maximum release rate (∼41–42 °C). In 10% FBS, ciprofloxacin release was <1% at 37 °C, 10–30% between 38–39.5 °C, and approximately 80% at 40 °C. Further, >90% ciprofloxacin release was noted at 41 °C or greater.
Figure 2. (a, b) Thermoscan assay in physiological buffer between 25–42 °C. Release of ciprofloxacin from LTSL was relatively greater in serum than in PBS. (c, d) Ciprofloxacin release kinetics; (c) Less than 20--30% ciprofloxacin release was noted from 25--39 °C upon incubation for 15 min in PBS; (d) In contrast, 30--60% release was noted in serum from 25--39 °C upon incubation for 15 min in FBS.
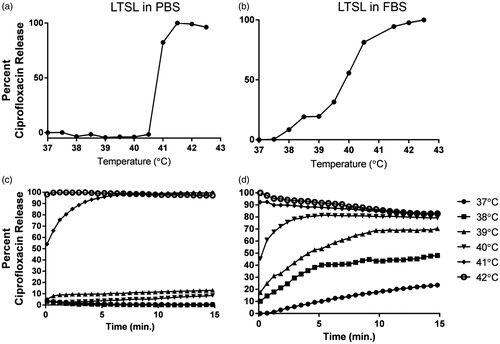
Ciprofloxacin release kinetics
When incubated for 15 min each at various temperature points (25–42 °C) in PBS, LTSL exhibited similar kinetics (). In general, between 25–38 °C release was <10%, and ∼20–30% release was seen at 39 °C, and >95% release was achieved by 42 °C (within a few seconds). Compared to PBS, release was more prominent in FBS with 20–25% of the drug releasing at 37 °C (), and by 39–40 °C, about 70–80% of ciprofloxacin release was observed. Additionally, >90% release at 40 °C or greater was observed in serum.
Efficacy of ciprofloxacin
Disc diffusion test
The antibacterial activity of LTSLs (±heat) evaluated against S. aureus inhibited bacterial growth (). In general, dialysate from LTSL plus heat resulted in a significantly larger zone of inhibition (15 ± 0.3 mm) compared to body temperature (11.5 ± 0.35 mm; p < 0.05 unpaired t-test, )
Impact of hyperthermia on bacterial growth and biofilm production
LTSL plus hyperthermia resulted in significant inhibition of S. aureus biofilms compared to LTSL alone (). At 42 °C, 60–70% S. aureus was killed upon free ciprofloxacin, or LTSL treatment (2.7–88 µM ciprofloxacin). LTSL alone had relatively lower killing rates (∼10%) compared to free ciprofloxacin (37 and 42 °C) or LTSL (∼42 °C). At saturated antimicrobial concentrations (175–350 µM), the survival rates between free ciprofloxacin, LTSL, and LTSL plus hyperthermia was similar (∼20–30%).
Figure 4. S. aureus survival following treatment of 5-day-old biofilms with free ciprofloxacin and LTSL at 37 and 42 °C (2.7–350 µM). At lower concentrations (2.7–44 µM), significant killing of S. aureus was noted for Cipro-LTSL and free ciprofloxacin at 42 °C compared to body temperature (p < 0.05, Tukey’s multiple comparison). At the super-saturated concentrations (>50 µM), similar survival was noted for all the groups.
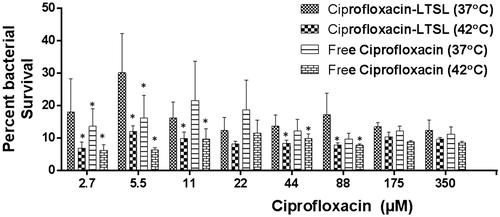
Scanning electron microscopy of biofilm
SEM confirmed biofilm growth on the peg device with the extracellular polymeric substance, or matrix, encompassing the staphylococci bacteria (). The matrix of LTSL treated biofilms at 37 °C was rough and the bacterial membrane appeared uniformly spherical (). In contrast, S. aureus biofilms that were exposed to 42 °C in combination with LTSL had areas of the extra polymeric substance (matrix) that appeared very smooth, while some areas around the edges of the biofilm growth appear very granular and rough (). Additionally, the bacteria cocci appeared deformed or possibly damaged, as they exhibited a less than uniformly spherical shape.
Figure 5. (a) Scanning electron micrograph of a Staphylococcus biofilm on the PEG of the MBEC biofilm device (arrow). (b) Biofilms treated at 37 °C with LTSL shows the presence of extracellular matrix substances, surrounding and encasing spherical S. aureus (arrow). (c) Biofilms treated at 42 °C with LTSL suggest a loss in the granularity of the matrix (arrow), and deformation of bacterial membrane.
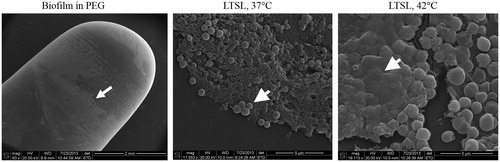
Image-guided hyperthermia
shows MR temperature maps in two perpendicular planes acquired at different time points after the sonication was initiated in the animal. A uniform heating pattern was achieved, and the size of the 42 °C isotherm, as indicated by the black contour, overlapped well with the desired treatment region. The mean temperature achieved within the heated ROI was 42.0° ± 0.2 °C, with a 90th percentile (T10) and 10th percentile (T90) of 43.2° ± 0.3 °C and 41.0° ± 0.3 °C respectively (), demonstrating precise temperature control. The rats’ body temperature was maintained at 37.3° ± 0.8 °C across all treatments indicating that the warming tray was working effectively. It took less than 3 min to achieve the target temperature range of 42 °C within the thigh, after which the temperature was maintained by the feedback controller. In order to trigger the rapid release of the liposomes without causing undesired heating within the surrounding tissue, a temperature range between 41–45 °C was required to achieve in this study. The ‘time in range’ which was defined as the period when all pixels within the heated ROI were between 41–45 °C, was estimated to be 31.4 min on average.
Figure 6. Temperature maps and measurements during treatment. (a) Temperature maps in two perpendicular planes at different time points (0, 10, 20, 30 and 40 min). The black contour is the 42° isotherm which corresponds well to the desired treatment region. (b) Mean, T90, and T10 temperatures within the target ROI. A tight temperature range was achieved and the body temperature curve confirmed the effectiveness of the warming tray.
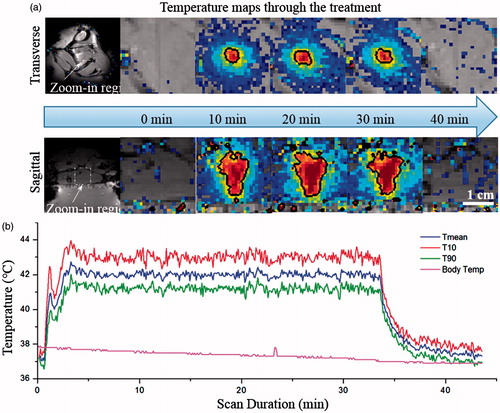
Ciprofloxacin biodistribution and targeted drug delivery
Ciprofloxacin biodistribution was determined in liver, kidney, spleen and heart tissues adjoining and contra-lateral to heated muscle in all treatment groups (n = 3; ). Ciprofloxacin was similar in the liver for various treatment groups (p > 0.05, Tukey). Treatment with free ciprofloxacin + MR-HIFU resulted in significantly higher accumulation (twofold) (p < 0.05, Tukey) in kidney and lung compared to LTSL + MR-HIFU. In contrast, ciprofloxacin delivery to spleen was twofold compared to free ciprofloxacin (p < 0.05, Tukey).
Figure 7. Biodistribution of ciprofloxacin in rats following treatment with ciprofloxacin + MR-HIFU or LTSL + MR-HIFU at a dose of 10 mg/kg administered i.v. Data are shown as mean ciprofloxacin concentration in the indicated tissues with standard error of mean (n = 3). *Free ciprofloxacin versus LTSL, p < 0.05, Tukey’s multiple comparison).
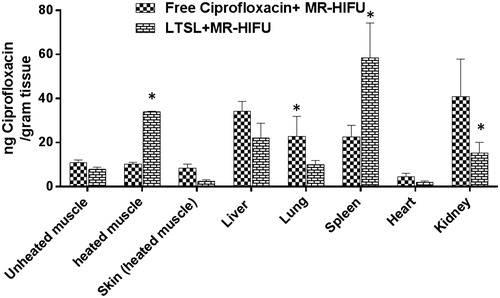
The heated muscle ciprofloxacin concentrations were 34 ± 0.3 and 8 ± 1 μg ciprofloxacin/g tissue for LTSL + MR-HIFU, and free ciprofloxacin, respectively (). LTSL + MR-HIFU resulted in a 4-fold greater tissue drug delivery compared to free ciprofloxacin (p < 0.05, Tukey) and 3-fold greater delivery compared to contralateral unheated muscle alone (p < 0.05, Tukey) (). Importantly, the level of free ciprofloxacin measured in both heated and unheated muscle was comparable, indicating no additional benefit derived from the heating alone. In terms of specificity of drug delivery to the heated muscle compared to adjacent skin (muscle:adjoining skin), the relative ciprofloxacin concentrations were 0.9 -, and 14.5-fold greater for the ciprofloxacin, and LTSL + MR-HIFU groups respectively (). The specificity of LTSL + MR-HIFU was significantly greater than the other treatments (p < 0.05, Tukey).
Figure 8. Specificity of drug delivery shown by relative ciprofloxacin concentration in rat superficial/deep thigh muscle as compared to adjacent muscle following treatment either with free ciprofloxacin or LTSL + MR-HIFU at a dose of 10 mg/kg ciprofloxacin. Data are shown as ratio of muscle (ciprofloxacin):skin (ciprofloxacin) with standard error of mean (n = 3). **p < 0.05.
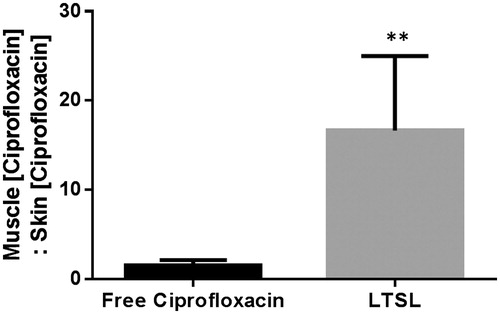
Table 1. Analysis of the muscle (±MR-HIFU) and organ ciprofloxacin at 4 h post-treatment.
Discussion
The objective of this study was to investigate the potential combination of ciprofloxacin-LTSLs with hyperthermia in vitro and in a rat model for achieving localised, triggered antimicrobial delivery potential therapy of chronic tissue infections. This study was a requisite first step towards translation of this image-guided focal drug delivery paradigm to a clinically relevant chronic wound-infected animal model.
In previous studies, LTSLs containing anticancer drugs (e.g. doxorubicin and gemcitabine) have been combined with an MR-HIFU system in a normal rabbit thigh and VX2 rabbit tumour model [Citation15] as well as TSLs and a clinical MR-HIFU system in a rat tumour model [Citation31]. Results from these studies [Citation15,Citation31] have demonstrated the ability to use MR-HIFU to enhance local drug delivery. However, the application of LTSLs for localised antimicrobial delivery has yet to be demonstrated. In this study we designed ciprofloxacin LTSLs using a remote loading procedure described by Oh et al. [Citation29]. This method of ciprofloxacin loading resulted in greater than 95% encapsulation of added drug with excellent thermal stability (<5% drug release between 25–39 °C in physiological medium, ). Because ciprofloxacin is a highly charged molecule, the range of lipid compositions that can encapsulate this molecule and maintain their nanoscale size is limited [Citation29]. Our size data suggest that the LTSL lipid composition adopted in this study provided the crucial properties of stable ciprofloxacin encapsulation needed, and prevented charge-induced irreversible aggregation of drug and lipids in physiological media (PBS) under the relevant conditions and temperatures. However, because LTSLs are also highly sensitive to serum proteins, the ciprofloxacin release in serum rich medium was relatively greater (∼30%) than PBS () [Citation32,Citation33]. This is in agreement with previous studies where the presence of albumin increased the membrane permeability of the LTSL bilayer [Citation32,Citation34]. Therefore, it is essential that ciprofloxacin-LTSL based formulations are fine-tuned further to improve in vivo stability. Studies are currently underway to study the impact of various lipid components on the release of antibiotics of various chemistries in serum containing medium.
For an optimal outcome it is critical that the impact of mild hyperthermia on the antimicrobial function of ciprofloxacin be determined. Many previous studies have theoretically established that the combination of hyperthermia and chemotherapy promote additional synergistic killing of infected cells [Citation35]. For instance, Mackowiak et al. [Citation36] demonstrated a progressive increase in antimicrobial activities of many drug molecules in broth medium and serum obtained from patients against both gram negative and positive bacteria as temperature was increased within the physiological range (37–43 °C). Similarly, Hajdu et al. reported that incubation with antibiotics (daptomycin, vancomycin, tigecycline, phosphomycin, and cefamandole) at 40 or 45 °C achieved significant reductions in the bacterial growth in S. aureus biofilm compared to body temperature [Citation37]. Although the mechanisms are not known, it is plausible that higher physiological temperatures induce changes in the biofilm matrix, and alter bacterial structure and ribosomal subunit functions, which in turn produce bactericidal response. As an initial step we characterised the structural integrity of biofilm upon addition of hyperthermia by SEM (). A decrease in granularity and roughness of the matrix and deformation of the bacterial membrane with hyperthermia was noted (). Additionally, marked reduction in bacterial viability at 42 °C in a dose-dependent manner following LTSL was observed compared to LTSLs at 37 °C ( and ). Most likely, alteration in the biofilm by hyperthermia enhances antimicrobial diffusion from the interstices and channels of the matrix, and disrupt the inner electrostatic equilibrium of the bacterial membrane, thereby destroying the bacterial membrane and microcolonies that were not dense or robust enough [Citation38].
Our ultimate long-term goals are to apply LTSL in combination with local mild heating for treatment of chronic wounds and biofilm-infested bone infection that currently require long duration antibiotic therapy, and are limited by modest drug delivery to the infection site [Citation39]. In previous studies, we have shown that MR-HIFU therapy can generate controlled heating within tissue and bone using active temperature feedback [Citation40,Citation41]. Therefore, the application of MR-HIFU for LTSL therapy of chronic wounds could be a clinically feasible combination of technologies especially for S. aureus osteomyelitis that remains an orthopaedic challenge because of bacterial adherence to bone extracellular matrix through the membrane adhesive proteins and glycoproteins via receptors to fibronectin and other structural proteins on the bone surface [Citation42,Citation43]. Currently, access and sensitivity of these pathogens to high dose antimicrobial dose is clinically challenging, and we speculate that a localised LTSL plus MR-HIFU combination would significantly improve the targeting of these pathogens. As an initial step we determined the targeted release of ciprofloxacin from LTSL in heated muscle tissues with a small animal preclinical MR-HIFU hyperthermia system. Biodistribution data suggest that drug delivery with LTSL + MR-HIFU hyperthermia is highly target-specific, as evidenced by a fourfold higher ciprofloxacin concentration in heated muscle compared to contralateral unheated muscle (). Additionally, the ciprofloxacin concentration in normal tissues was relatively similar and in some cases lower for LTSL + MR-HIFU compared to an equivalent concentration of free ciprofloxacin, thereby indicating that localised hyperthermia may significantly enhance localised delivery and safety of antimicrobial molecules. This is promising and is in line with several studies reported previously where significantly greater delivery of an anticancer agent (doxorubicin) was reported in combination with MR-HIFU [Citation16,Citation41,Citation44–46]. The likely mechanisms are attributed to increased tissue perfusion and the permeability of blood vessels to LTSLs that are mediated by stable and long duration hyperthermic temperature administration by MR-HIFU [Citation45,Citation47,Citation48]. However, our in vivo study has several limitations. First, the efficiency of LTSL drug delivery in infected bone tissue was not investigated. Second, only healthy muscle that does not necessarily simulate the dynamic complexity of a chronically infected wound tissue was examined. Nevertheless, this initial study addressed the feasibility of localised antimicrobial delivery in vitro and in vivo. We believe that the data presented in this study will help guide the development of targeted antimicrobial delivery, thereby addressing the important public health issue of chronic infections.
Conclusion
Building on prior success combining LTSLs and MR-HIFU for a targeted drug delivery system [Citation16,Citation46,Citation49,Citation50], we report on the development of an antimicrobial-loaded LTSL. Our data suggest that the approach presented in this work confers LTSLs with the crucial properties needed of triggered delivery in vitro and in vivo, and proof that LTSL encapsulation does not influence bacterial bioactivity until release is triggered. At higher temperatures (42 °C), extended treatment can impact the biofilm matrix and deform the bacterial membrane, resulting in lower survival rates, suggesting potential synergy between antimicrobial agents and hyperthermia.
Acknowledgements
We thank Terry Colberg and Lisa Whitworth from Oklahoma State University Microscopy Laboratory for assistance in preparing biofilm samples and collecting scanning electron microscope images.
Declaration of interest
Research reported in this publication was supported by the Center for Veterinary Health Sciences seed support (AR) and the Cancer Prevention and Research Initiative of Texas, grant number R1308 (RC). The content is solely the responsibility of the authors and does not necessarily represent the official views of the Oklahoma State University. Salary support for R.S. was provided by Philips Research; however, the company did not provide any financial support or input on the development of the LTSL formulation described in this manuscript.
References
- Siddiqui AR, Bernstein JM. Chronic wound infection: Facts and controversies. Clin Dermatol 2010;28:519–26
- Sibbald RG, Woo K, Ayello EA. Increased bacterial burden and infection: The story of NERDS and STONES. Adv Skin Wound Care 2006;19:447–61; quiz 61–3
- Kasimanickam RK, Ranjan A, Asokan GV, Kasimanickam VR, Kastelic JP. Prevention and treatment of biofilms by hybrid- and nanotechnologies. Int J Nanomed 2013;8:2809–19
- Lipsky BA, Hoey C. Topical antimicrobial therapy for treating chronic wounds. Clin Infect Dis 2009;49:1541–9
- Marriott R, Rubayi S. Successful truncated osteomyelitis treatment for chronic osteomyelitis secondary to pressure ulcers in spinal cord injury patients. Ann Plast Surg 2008;61:425–9
- Werdin F, Tennenhaus M, Schaller HE, Rennekampff HO. Evidence-based management strategies for treatment of chronic wounds. Eplasty 2009;9:e19
- Sun X, Jiang K, Chen J, Wu L, Lu H, Wang A, et al. A systematic review of maggot debridement therapy for chronically infected wounds and ulcers. Int J Infect Disease 2014;25:32–7
- Amalsadvala T, Swaim SF. Management of hard-to-heal wounds. Vet Clin North Am Small Anim Pract 2006;36:693–711
- Howell-Jones RS, Wilson MJ, Hill KE, Howard AJ, Price PE, Thomas DW. A review of the microbiology, antibiotic usage and resistance in chronic skin wounds. J Antimicrob Chemother 2005;55:143–9
- Price CI, Horton JW, Baxter CR. Liposome encapsulation: A method for enhancing the effectiveness of local antibiotics. Surgery 1994;115:480–7
- Price CI, Horton JW, Baxter CR. Topical liposomal delivery of antibiotics in soft tissue infection. J Surg Res 1990;49:174–8
- Aka ST, Haji SH. Sub-MIC of antibiotics induced biofilm formation of Pseudomonas aeruginosa in the presence of chlorhexidine. Braz J Microbiol 2015;46:149–54
- Kong G, Anyarambhatla G, Petros WP, Braun RD, Colvin OM, Needham D, et al. Efficacy of liposomes and hyperthermia in a human tumor xenograft model: Importance of triggered drug release. Cancer Res 2000;60:6950–7
- Yatvin MB, Weinstein JN, Dennis WH, Blumenthal R. Design of liposomes for enhanced local release of drugs by hyperthermia. Science 1978;202(4374):1290–3
- Staruch R, Chopra R, Hynynen K. Localised drug release using MRI-controlled focused ultrasound hyperthermia. Int J Hyperthermia 2010;27:156–71
- Ranjan A, Jacobs GC, Woods DL, Negussie AH, Partanen A, Yarmolenko PS, et al. Image-guided drug delivery with magnetic resonance guided high intensity focused ultrasound and temperature sensitive liposomes in a rabbit Vx2 tumor model. J Control Release 2012;158:487–94
- Staruch RM, Ganguly M, Tannock IF, Hynynen K, Chopra R. Enhanced drug delivery in rabbit VX2 tumours using thermosensitive liposomes and MRI-controlled focused ultrasound hyperthermia. Int J Hyperthermia 2012;28:776–87
- Manzoor AA, Lindner LH, Landon CD, Park JY, Simnick AJ, Dreher MR, et al. Overcoming limitations in nanoparticle drug delivery: Triggered, intravascular release to improve drug penetration into tumors. Cancer Res 2012;72:5566–75
- Fernando R, Downs J, Maples D, Ranjan A. MRI-guided monitoring of thermal dose and targeted drug delivery for cancer therapy. Pharm Res 2013;30:2709–17
- de Smet M, Hijnen NM, Langereis S, Elevelt A, Heijman E, Dubois L, et al. Magnetic resonance guided high-intensity focused ultrasound mediated hyperthermia improves the intratumoral distribution of temperature-sensitive liposomal doxorubicin. Invest Radiol 2013;48:395–405
- Douglas LJ. Penetration of antifungal agents through Candida biofilms. Methods Mol Biol 2009;499:37–44
- Serra R, Grande R, Butrico L, Rossi A, Settimio UF, Caroleo B, et al. Chronic wound infections: The role of Pseudomonas aeruginosa and Staphylococcus aureus. Expert Rev Anti-InfectTher 2015;13:605–13
- Fazli M, Bjarnsholt T, Kirketerp-Moller K, Jorgensen B, Andersen AS, Krogfelt KA, et al. Nonrandom distribution of Pseudomonas aeruginosa and Staphylococcus aureus in chronic wounds. J Clin Microbiol 2009;47:4084–9
- Phillips HL, Nolen TM, Hutchison J. Efficacy of ciprofloxacin in the treatment of various bacterial infections. Alabama Med 1989;58:28–32
- Maples D, McLean K, Sahoo K, Newhardt R, Venkatesan P, Wood B, et al. Synthesis and characterisation of ultrasound imageable heat-sensitive liposomes for HIFU therapy. Int J Hyperthermia 2015;31:674–85
- Senavirathna LK, Fernando R, Maples D, Zheng Y, Polf JC, Ranjan A. Localised hyperthermia in rodent models using an MRI-compatible high-intensity focused ultrasound system. Int J Hyperthermia 2015;31(8):687–91
- Fernando R, Maples D, Senavirathna LK, Zheng Y, Polf JC, Benton ER, et al. Hyperthermia sensitization and proton beam triggered liposomal drug release for targeted tumor therapy. Pharm Res 2014;31:3120–6
- Mayer L, Bally M, Cullis P. Uptake of Adriamycin into large unilamellar vesicles in response to a pH gradient. Biochim Biophys Acta 1986;857:123–6
- Oh YK, Nix DE, Straubinger RM. Formulation and efficacy of liposome-encapsulated antibiotics for therapy of intracellular Mycobacterium avium infection. Antimicrobial agents and chemotherapy. 1995;39:2104–11
- Parker AE, Walker DK, Goeres DM, Allan N, Olson ME, Omar A. Ruggedness and reproducibility of the MBEC biofilm disinfectant efficacy test. J Microbiol Methods. 2014;102:55–64
- de Smet M, Heijman E, Langereis S, Hijnen NM, Grull H. Magnetic resonance imaging of high intensity focused ultrasound mediated drug delivery from temperature-sensitive liposomes: An in vivo proof-of-concept study. J Control Release 2011;150:102–10
- Hossann M, Syunyaeva Z, Schmidt R, Zengerle A, Eibl H, Issels RD, et al. Proteins and cholesterol lipid vesicles are mediators of drug release from thermosensitive liposomes. J Control Release 2012;162:400–6
- Hossann M, Wang T, Wiggenhorn M, Schmidt R, Zengerle A, Winter G, et al. Size of thermosensitive liposomes influences content release. J Control Release 2010;147:436–43
- Hossann M, Wiggenhorn M, Schwerdt A, Wachholz K, Teichert N, Eibl H, et al. In vitro stability and content release properties of phosphatidylglyceroglycerol containing thermosensitive liposomes. Biochim Biophys Acta 2007;1768:2491–9
- Mackowiak PA, Marling-Cason M, Cohen RL. Effects of temperature on antimicrobial susceptibility of bacteria. J Infect Dis 1982;145:550–3
- Mackowiak PA, Marling-Cason M. Hyperthermic enhancement of serum antimicrobial activity: Mechanism by which fever might exert a beneficial effect on the outcome of gram-negative sepsis. Infect Immun 1983;39:38–42
- Hajdu S, Holinka J, Reichmann S, Hirschl AM, Graninger W, Presterl E. Increased temperature enhances the antimicrobial effects of daptomycin, vancomycin, tigecycline, fosfomycin, and cefamandole on staphylococcal biofilms. Antimicrob Agents Chemother 2010;54:4078–84
- Dong D, Thomas N, Thierry B, Vreugde S, Prestidge CA, Wormald PJ. Distribution and inhibition of liposomes on Staphylococcus aureus and Pseudomonas aeruginosa biofilm. PloS One 2015;10:e0131806
- Nason R, Chole RA. Bacterial biofilms may explain chronicity in osteoradionecrosis of the temporal bone. Otol Neurotol 2007;28:1026–8
- Ramsay E, Mougenot C, Kazem M, Laetsch TW, Chopra R. Temperature-dependent MR signals in cortical bone: Potential for monitoring temperature changes during high-intensity focused ultrasound treatment in bone. Magn Reson Med 2014;74:1095–102
- Staruch R, Chopra R, Hynynen K. Hyperthermia in bone generated with MR imaging-controlled focused ultrasound: Control strategies and drug delivery. Radiology 2012;263:117–27
- Jorge LS, Chueire AG, Rossit AR. Osteomyelitis: A current challenge. Braz J Infect Dis 2010;14:310–15
- Dinh T, Snyder G, Veves A. Current techniques to detect foot infection in the diabetic patient. Int J Low Extrem Wounds 2010;9:24–30
- Deckers R, Moonen CT. Ultrasound triggered, image guided, local drug delivery. J Control Release 2003;148:25–33
- Gasselhuber A, Dreher MR, Partanen A, Yarmolenko PS, Woods D, Wood BJ, et al. Targeted drug delivery by high intensity focused ultrasound mediated hyperthermia combined with temperature-sensitive liposomes: Computational modelling and preliminary in vivo validation. Int J Hyperthermia 2012;28:337–48
- Lindner LH, Eichhorn ME, Eibl H, Teichert N, Schmitt-Sody M, Issels RD, et al. Novel temperature-sensitive liposomes with prolonged circulation time. Clin Cancer Res 2004;10:2168–78
- Hijnen N, Langereis S, Grull H. Magnetic resonance guided high-intensity focused ultrasound for image-guided temperature-induced drug delivery. Adv Drug Del Rev 2014;72:65–81
- Grull H, Langereis S. Hyperthermia-triggered drug delivery from temperature-sensitive liposomes using MRI-guided high intensity focused ultrasound. J Control Release 2012;161:317–27
- Needham D, Anyarambhatla G, Kong G, Dewhirst MW. A new temperature-sensitive liposome for use with mild hyperthermia: Characterization and testing in a human tumor xenograft model. Cancer Res 2000;60:1197–201
- Koning GA, Eggermont AM, Lindner LH, ten Hagen TL. Hyperthermia and thermosensitive liposomes for improved delivery of chemotherapeutic drugs to solid tumors. Pharm Res 2010;27:1750–4