Abstract
Purpose Patchouli alcohol (PA) is used to treat gastrointestinal dysfunction. The purpose of this study was to ascertain the function of PA in the regulated process of oxidative stress in rat intestinal epithelial cells (IEC-6). Materials and methods Oxidative stress was stimulated by exposing IEC-6 cells to heat shock (42 °C for 3 h). IEC-6 cells in treatment groups were pretreated with various concentrations of PA (10, 40, and 80 ng/mL) for 3 h before heat shock. Results Heat shock caused damage to the morphology of IEC-6 cells, and increased reactive oxygen species (ROS) level and malondialdehyde (MDA) content. Moreover, mRNA and protein expression by target genes related to oxidative stress in heat shock were also altered. Specifically, the mRNA expression by HSP70, HSP90, GSH-px, NRF2 nd HO-1were all increased, and Nrf2 and Keap1 protein expression were increased after heat shock. However, pretreatment with PA weakened the level of damage to the cellular morphology, and decreased the MDA content caused by heat shock, indicating PA had cytoprotective activities. Pretreatment with PA at high dose significantly increased generation of intracellular ROS. Compared with the heat shock group alone, PA pretreatment significantly decreased the mRNA expression by HSP70, HSP90, SOD, CAT, GSH-px, KEAP1 and HO-1. Furthermore, the high dose of PA significantly increased Nrf2 protein expression, while both the intermediate and high dose of PA significantly increased HO-1 protein expression. Conclusion Heat-shock-induced oxidative stress in IEC-6 cells, and PA could alleviate the Nrf2-Keap1 cellular oxidative stress responses.
Introduction
As a critical component of the gut barrier, intestinal epithelial cells prevent the transfer of harmful microorganisms, antigens and toxins from the gut lumen into the circulation [Citation1,Citation2]. Recent research has found that hyperthermia can compromise the gut barrier, but studies have mostly focused on tissue damage and repair, apoptosis and immune function [Citation3–6]. For example, heat stress was reported to cause damage and apoptosis in IEC-6 cells [Citation4,Citation5], which resembled the effects of oxidative stress [Citation7]. Similarly, mouse testes were reported to have suffered oxidative damage after whole body heat stress, and a separate study found that hyperthermia exposure caused injury to the intestinal lining that was attributed to oxidative stress [Citation7,Citation8]. Therefore, to follow up on our previous study [Citation4], we investigated the effects of a heat-induced oxidative stress model by treating IEC-6 cells at 42 °C for 3 h.
Oxidative stress is induced by many factors, such as xenobiotics, drugs and heavy metals [Citation9]. Oxidative stress produced by reactive oxygen species (ROS) can cause DNA damage, cell cycle dysfunction, apoptosis, or even death [Citation9–11]. The intestinal epithelium is prone to oxidative damage induced by luminal oxidants [Citation2]. The intestinal redox status maintains the mucosal integrity, supports luminal nutrient absorption, and governs the regenerative potential of the mucosa, the disruption of which results in mucosal oxidative stress [Citation2,Citation12]. ROS and oxidative stress play a central role in causing excessive inflammation, tissue damage and sepsis [Citation13]. The balance between the level of ROS formation and anti-oxidants is crucial for cell survival and growth [Citation10,Citation14,Citation15].
The nuclear factor (erythroid-derived 2)-related factor 2 (Nrf2)-Kelch like-ECH-associated protein (Keap1) pathway is a cellular sensor of oxidative stress, and is critical for cellular protection and cell survival [Citation9]. Upon exposure to oxidative stress, Nrf2 disassociates from the repressor protein Keap1, and translocates to the nucleus where it binds to antioxidant-responsive elements (AREs) located in the promoter region of cytoprotective genes, such as detoxifying enzymes and antioxidant proteins, and induces their transcription [Citation16]. Malondialdehyde (MDA) can serve as a marker of oxidative stress, since the amount of MDA reflects the degree of lipid peroxidation [Citation17,Citation18]. In contrast, superoxide dismutase (SOD), catalase (CAT) and glutathione peroxidase (GSH-px) are three important antioxidants. SOD is a major scavenger of O2•− and it converts ROS produced in the body to hydrogen peroxide (H2O2), which is decomposed to water and oxygen by CAT [Citation14,Citation19]. GSH-px is the major H2O2 reducing intestinal selenoprotein [Citation2]. Previously, it has been reported that whole-body heat shock triggered oxidative stress in mice testes and increased GSH-px levels, as well as CAT and SOD activities [Citation8].
Pogostemonis herba, a common Chinese herb, has long been used to remove dampness, relieve sunstroke and regulate gastrointestinal functions in China [Citation20,Citation21]. Patchouli alcohol (PA), a tricyclic sesquiterpene and mainly derived from Pogostemonis herba, has been reported to display anti-inflammatory, antiviral, selective antibacterial activity against Helicobacter pylori, and significant gastro-protective effects against gastric ulceration [Citation21–25]. However, the molecular mechanism for how PA confers a cytoprotective effect by regulating the oxidative stress response remains unclear. The purpose of this study was to investigate the antioxidant and Nrf2-Keap1 pathway response of PA in rat intestinal epithelial cells, and ascertain its function in the regulated process of heat shock-induced oxidative stress.
Materials and methods
Cell culture
Rat IEC-6 cells (CRL 21592, Peking Union Medical College, Beijing, China) were cultured in Dulbecco’s modified Eagle’s medium (DMEM) containing 10% (v/v) fetal bovine serum (FBS, 10099-141, Grand Island, NY, USA), 2 mg/L insulin, 50 IU/mL penicillin and 50 mg/mL streptomycin and incubated at 37 °C under 5% (v/v) CO2. The medium was replaced 48 h following initial cell plating.
Cell viability assay
PA (C15H26O, purity 99.9%, National Institutes for Food and Drug Control, Beijing) was diluted in DMEM (1 mg/mL). To measure cell viability, equivalent numbers of IEC-6 cells were plated on 96-well plates (3603, Corning, NY) and cultured in DMEM containing 10% FBS. After cells were grown to 90% confluence, cells were washed twice with phosphate buffered saline (PBS) and serum starved for 2 h, and then cells were treated with various concentrations of PA (10, 20, 40, 80, 100 ng/mL) for 3 h, 6 h, 12 h and 24 h. 10 μL of 3-(4,5-dimethylthiazol-2-yl)-2,5-diphenyltetrazolium bromide (MTT) (5 mg/mL, Amresco, Solon, OH) was added to each well and incubated at 37 °C for 4 h. Subsequently the media was aspirated from the wells and the formazan product was dissolved using dimethyl sulfoxide (Amresco). The plate was analysed using a microplate reader (Bio-Rad Laboratories, Foster City, CA) at a fixed absorption wavelength of 490 nm and a reference wavelength of 630 nm.
Morphological examination
Control cells were kept strictly at 37 °C under 5% CO2, while cells of the heat shock group were exposed to 42 °C and 5% CO2 for 3 h. In the PA pretreatment groups, once IEC-6 cells reached 90% confluence they were washed twice with PBS, and then pretreated with PA (10, 40, 80 ng/mL) at 37 °C, 5% CO2 for 3 h before being exposed to heat shock (42 °C, 5% CO2) for 3 h. Changes in cell morphology following all treatments were observed using a phase-contrast inverted biological microscope (IX71/IX2, Olympus, Tokyo, Japan).
ROS and MDA determination
Intracellular ROS in IEC-6 cells was determined with ROS detection reagents (carboxy-H2DCFDA, catalogue no. 400, Invitrogen, Carlsbad, CA) according to the manufacturer’s instructions. Briefly, IEC-6 cells were seeded on 6-well cell culture plates. Cells from the heat shock group, with or without PA pretreatment (10, 40, 80 ng/mL), were exposed to 42 °C for 3 h. Cells were removed from growth media via centrifugation and 1 mL of ROS indicator media dilute solution (carboxy-H2DCFDA, 1:20 000 diluted) was added to each well, then incubated at 37 °C for 10 min. After treatment, fluorescence-intensity-loaded control and heat-shocked IEC-6 cells were measured with flow cytometry (BD Biosciences, San Jose, CA). The concentration of MDA in the supernatant of IEC-6 cells was determined using detection kits (A003, Nanjing Jiancheng Bioengineering Institute, Nanjing, China).
Quantitative RT-PCR analysis
Total RNA was isolated from IEC-6 cells using a phenol and guanidine isothiocyanate-based TRIzol reagent (Invitrogen) according to the manufacturer’s instructions. The RNA quality assessment and reverse transcription (RT) were performed as previously described [Citation6]. The polymerase chain reaction (PCR) system (20 μL in total) contained 10 μL of SYBR Green PCR mix (Stratagene, La Jolla, CA), 0.3 μL of reference dye, 1 μL of each primer (both 10 μmol/L), 1 μL of cDNA template, and 6.7 μL of diethyl-pyro-carbonate-treated water. The cycling conditions were as follows: 95 °C for 3 min, followed by 40 cycles of 95 °C for 10 s, 60 °C for 20 s, and 72 °C for 60 s. Quantitative polymerase chain reaction (PCR) analysis was carried out using the DNA Engine Mx3000P® fluorescence detection system (Agilent, Santa Clara, CA) against a double-stranded DNA-specific fluorescent dye (Stratagene, La Jolla, CA) according to optimised PCR protocols. Expression levels were determined using the relative threshold cycle (CT) method as described by the manufacturer (Stratagene). Reverse transcription-generated cDNA encoding β-actin, HSP70, HSP90, SOD, CAT, GSH-px, NRF2, KEAP1 and HO-1 were amplified by real-time PCR using selective primers (). β-actin was amplified in parallel with the target genes and used as a normalisation control.
Table 1. Primers used for real-time PCR.
Western blot analysis
Proteins from IEC-6 cells were extracted using a total protein extraction kit (BioChain Institute, Hayward, CA) and quantified using a BCA protein assay kit (Pierce Biotechnology, Rockford, IL). Proteins (10–20 μg/sample) were separated by SDS-PAGE (Invitrogen), transferred to nitrocellulose membranes (88585, Pierce), and then hybridised with the specific antibodies. The following antibodies used were as follows: Nrf2 (ab137550, Abcam, Tokyo, Japan), Keap1 (4617S, Cell Signaling Technology, Danvers, MA), HO-1 (BS6626, Bioworld, St Louis Park, MN) and β-actin (5057, Cell Signaling Technology). Blots were normalised by using β-actin to correct for differences in loading of the proteins. Proteins were detected using an Odyssey Infrared Imaging System (LI-COR Biosciences, Lincoln, NE). Densitometric values of immunoblot signals were obtained from three separate experiments using Image J software (National Institutes of Health, Bethesda, MD).
Statistical analysis
All data were obtained from at least three independent experiments performed in triplicate and the results were presented as the mean ± SEM, with a value of p < 0.05 considered statistically significant. Statistical analyses were carried out with SPSS 12.0 software (IBM, Armonk, NY) and graphs were created with Origin 6.0 (OriginLab, Northampton, MA).
Results
The cytotoxicity of PA on IEC-6 cells
To select a proper concentration of PA for further research with the IEC-6 cells, cells were exposed to various concentrations of PA and cell viability was determined using the MTT assay (). The viability of IEC-6 cells did not change after pretreatment with PA at concentrations of 10, 40 and 80 ng/mL respectively, indicating PA had no cytotoxic effect on IEC-6 cells below 80 ng/mL. However, after being stimulated with 100 ng/mL of PA for 6 h, cell viability significantly decreased (p < 0.01). Accordingly, concentrations of 10 (low dose), 40 (middle dose), and 80 ng/mL (high dose) of PA were chosen for further research on the cytoprotective effects of PA against oxidative stress.
Figure 1. The cytotoxicity of PA on IEC-6 cells. PA had no cytotoxic effect on IEC-6 cells in concentrations from 0 to 80 ng/mL. After stimulation with 100 ng/mL of PA for 6, 12 and 24 h, cell viability was significantly inhibited. Data are mean ± SEM, n = 6 per treatment (*p < 0.05 vs. control group; **p < 0.01 vs. control group).
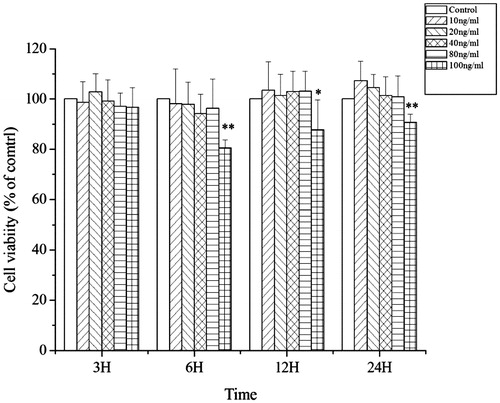
Morphology of IEC-6 cells
Under normal conditions, the morphology of IEC-6 cells was compact and regular (). Upon heat shock (42 °C for 3 h), the morphology of IEC-6 cells markedly changed (, red arrows indicate cells with altered morphology). Pretreatment of IEC-6 cells with PA at 10 ng/mL (), 40 ng/mL () and 80 ng/mL () for 3 h before they were placed at 42 °C improved cell morphology, with the cells appearing more compact and ordered. Thus, PA protected the cellular morphology and arrangement of IEC-6 cells exposed to heat shock, presumably by dampening the amount of damage caused by heat shock.
Figure 2. Morphology of IEC-6 cells. In the normal condition, the distribution of IEC-6 cells was compact and regular (A). Heat shock markedly altered IEC-6 cellular morphology; red arrows represent changes in cell shape (B). PA regulated cellular morphology and the arrangements of IEC-6 cells returned to normal. (A) control cells without any processing; (B)heat shock; (C) pretreatment with 10 ng/mL PA + heat shock; (D) pretreatment with 40 ng/mL PA + heat shock; (E) pretreatment with 80 ng/mL PA + heat shock). Scale bar: 50 μm.
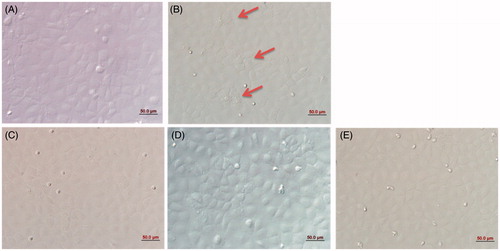
The mRNA expression of heat shock proteins (HSPs) in IEC-6 cells
Compared with the control group, the mRNA expression by HSP70 (p < 0.01) and HSP90 (p < 0.01) in the heat-shock group was significantly increased to 425.3-fold and 11.2-fold, respectively (), which revealed IEC-6 cells were stressed. Compared with the heat shock group, pretreatment with PA at 10 ng/mL (p < 0.05), 40 ng/mL (p < 0.01) and 80 ng/mL (p < 0.01) significantly decreased the mRNA expression by HSP70 to 0.74-fold, 0.42-fold and 0.40-fold, respectively (). Furthermore, pretreatment with PA at 40 ng/mL (p < 0.01) and 80 ng/mL (p < 0.05) significantly decreased the mRNA expression by HSP90 to 0.60-fold and 0.61-fold, respectively ().
Figure 3. mRNA expression by HSP70 and HSP90 in IEC-6 cells. Compared with the control group, the mRNA expression by HSP70 and HSP90 in the heat shock group was significantly increased. Compared with the heat shock group, PA significantly decreased the mRNA expression by HSP70 (A), PA pretreatment at middle dose (40 ng/mL) and high dose (80 ng/mL) significantly decreased the mRNA expression by HSP90 (B). Control, control cells without any processing; HS, heat shock; Low, pretreatment with 10 ng/mL PA + heat shock; Middle, pretreatment with 40 ng/mL PA + heat shock; High, pretreatment with 80 ng/mL PA + heat shock. Data are mean ± SEM, n = 3 per treatment (**p < 0.01 vs. control group; #p < 0.05 vs. heat shock group; ##p < 0.01 vs. heat shock group).
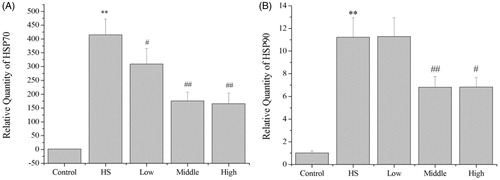
Effects of PA on ROS, MDA, and oxidative stress response
Generation of intracellular ROS was detected by flow cytometry after carboxy-H2DCFDA treatment, and data showed the fluorescence intensity of IEC-6 cells (). Compared with the control group, the fluorescence intensity in the heat-shock group was increased to 2.1-fold, indicating heat shock significantly increased (p < 0.05) the intracellular ROS (). Compared with the heat shock group, pretreatment with PA at 80 ng/mL significantly increased the fluorescence intensity to 1.64-fold, indicating pretreatment with PA at 80 ng/mL significantly increased (p < 0.05) the intracellular ROS (). Compared with the control group, heat shock significantly (p < 0.05) increased the MDA content in the supernatant of IEC-6 cells to 1.79-fold (). Compared with the heat shock group, pretreatment with PA at 10 ng/mL (p < 0.01), 40 ng/mL (p < 0.01) and 80 ng/mL (p < 0.01) significantly decreased the MDA content to 0.52-fold, 0.49-fold and 0.56-fold, respectively ().
Figure 4. Effects of PA on the generation of intracellular ROS and MDA content in response to heat shock. Generation of intracellular ROS was detected by flow cytometry (A). Compared with the control group, heat shock significantly increased the intracellular ROS. Compared with the heat shock group, pretreatment with PA at 80 ng/mL significantly increased the intracellular ROS (B). Compared with the control group, heat shock significantly increased the MDA content in the supernatant of IEC-6 cells. Compared with the heat shock group, the MDA content in the low (10 ng/mL), middle (40 ng/mL) and high dose (80 ng/mL) of PA pretreatment groups was significantly decreased (C). Control, control cells without any processing; HS, heat shock; Low, pretreatment with 10 ng/mL PA + heat shock; Middle, pretreatment with 40 ng/mL PA + heat shock; High, pretreatment with 80 ng/mL PA + heat shock. Data are mean ± SEM, n = 3 per treatment (*p < 0.05 vs. control group; **p < 0.01 vs. control group; ##p < 0.01 vs. heat shock group).
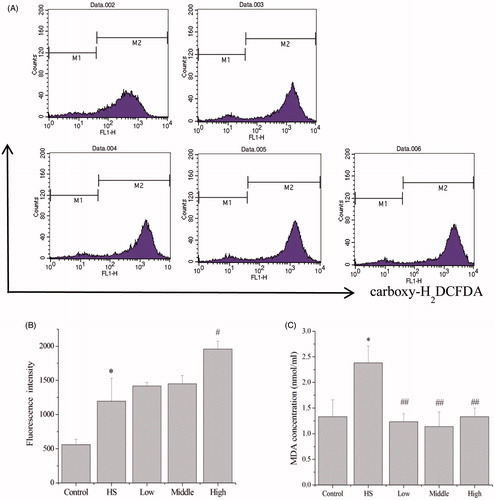
In response to heat shock there was a significant increase (p < 0.01) in the mRNA expression by GSH-px mRNA, but there was no change in the mRNA expression by SOD or CAT. Compared with the heat shock group, pretreatment with PA at 10 ng/mL (p < 0.05), 40 ng/mL (p < 0.01) and 80 ng/mL (p < 0.01) significantly decreased the mRNA expression by SOD to 0.74-fold, 0.59-fold and 0.56-fold, respectively (). Pretreatment with PA at 10 ng/mL (p < 0.01), 40 ng/mL (p < 0.01) and 80 ng/mL (p < 0.05) significantly decreased the mRNA expression by CAT to 0.63-fold, 0.63-fold and 0.68-fold, respectively (). Pretreatment with PA at 10 ng/mL (p < 0.05), 40 ng/mL (p < 0.05) and 80 ng/mL (p < 0.05) significantly decreased the mRNA expression by GSH-px to 0.46-fold, 0.44-fold and 0.44-fold, respectively ().
Figure 5. Effects of PA on oxidative stress response. Heat shock did not change the mRNA expression by SOD or CAT, but significantly increased GSH-px mRNA expression. Compared with the heat shock group, PA significantly decreased mRNA expression by SOD, CAT and GSH-px (A–C). Control, control cells without any processing; HS, heat shock; Low, pretreatment with 10 ng/mL PA + heat shock; Middle, pretreatment with 40 ng/mL PA + heat shock; High, pretreatment with 80 ng/mL PA + heat shock. Data are mean ± SEM, n = 3 per treatment (**p < 0.01 vs. control group; #p < 0.05 vs. heat shock group; ##p < 0.01 vs. heat shock group).
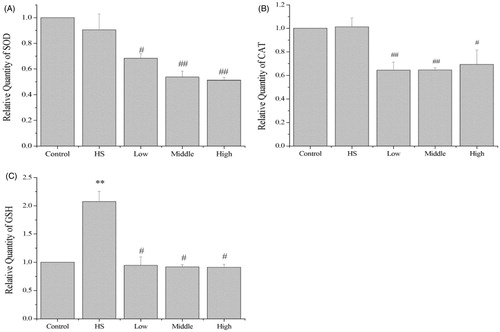
Effects of PA on the expression of Nrf2, Keap1 and HO-1
Heat shock significantly increased NRF2 (p < 0.01) and HO-1 (p < 0.01) mRNA expression in the IEC-6 cells to 103.9-fold and 68.8-fold, respectively (). However, heat shock did not change KEAP1 mRNA expression (). Relative to the heat shock group alone, pretreatment with PA at 10 ng/mL (p < 0.01), 40 ng/mL (p < 0.05) and 80 ng/mL (p < 0.01) significantly decreased the mRNA expression by KEAP1 to 0.43-fold, 0.56-fold and 0.55-fold, respectively (), pretreatment with PA at 10 ng/mL (p < 0.01), 40 ng/mL (p < 0.05) and 80 ng/mL (p < 0.01) significantly decreased the mRNA expression by HO-1 mRNA expression to 0.72-fold, 0.60-fold and 0.61-fold, respectively ().
Figure 6. Effects of PA on the mRNA expression by NRF2, KEAP1 and HO-1. Heat shock significantly increased NRF2 and HO-1 mRNA expression in the IEC-6 cells (A and C). However, heat shock did not change KEAP1 mRNA expression (B). Compared with the heat shock group, PA significantly decreased KEAP1 and HO-1 mRNA expression (B and C). Control, control cells without any processing; HS, heat shock; Low, pretreatment with 10 ng/mL PA + heat shock; Middle pretreatment with 40 ng/mL PA + heat shock; High, pretreatment with 80 ng/mL PA + heat shock. Data are mean ± SEM, n = 3 per treatment (**p < 0.01 vs. control group; #p < 0.05 vs. heat shock group; ##p < 0.01 vs. heat shock group).
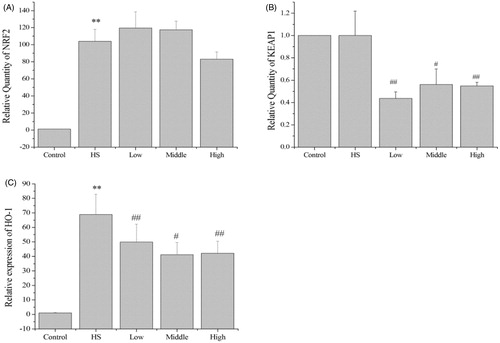
In agreement with the changes in mRNA levels, heat shock significantly increased Nrf2 protein expression to 2.1-fold (p < 0.05, ). Compared with the heat shock group, pretreatment with PA at 80 ng/mL significantly increased Nrf2 protein expression to 1.9-fold (p < 0.05, ), while pretreatment with PA at 40 ng/mL (p < 0.05) and 80 ng/mL (p < 0.05) significantly increased HO-1 protein expression to 1.6-fold and 1.5-fold, respectively (). But PA did not significantly change the protein expression of Keap1.
Figure 7. Effects of PA on the protein expression of Nrf2, Keap1 and HO-1. Heat shock significantly increased Nrf2 and Keap1 protein expression (A–C). Compared with the heat shock group, PA at high dose significantly increased (p < 0.05) Nrf2 protein expression (A, B), PA at middle and high doses significantly increased (p < 0.05) HO-1 protein expression (A, D), but PA did not significantly change the protein expression of Keap1 (A, C). Control, control cells without any processing; HS, heat shock; Low, pretreatment with 10 ng/mL PA + heat shock; Middle, pretreatment with 40 ng/mL PA + heat shock; High, pretreatment with 80 ng/mL PA + heat shock. Data are mean ± SEM, n = 3 per treatment (*p < 0.05 vs. control group; #p < 0.05 vs. heat shock group).
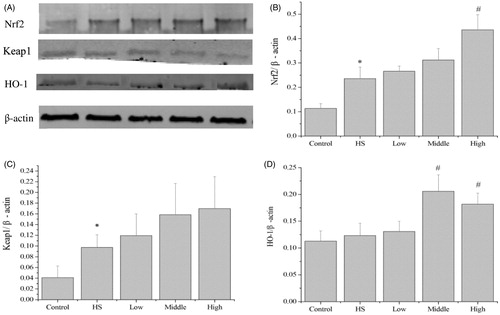
Discussion
Pogostemonis herba is widely used in the treatment of gastrointestinal dysfunction in China, and PA has free radical scavenging and anti-inflammatory properties [Citation21,Citation26]. A pharmacokinetic study about oral PA administration (10 mg/kg) in rats revealed that the maximum concentration of PA in rat plasma was 99.83 ng/mL, and the elimination half-life was 21.51 h [Citation20], which provided a meaningful basis for evaluating the clinical application of patchouli alcohol. In this study, PA was used to regulate heat-induced oxidative stress responses. After heat hock, PA significantly decreased HSP70 and HSP90 mRNA expression at 40 ng/mL, revealing PA was effective in preventing heat shock in IEC-6 cells.
Heat shock proteins (HSPs) protect intestinal mucosal against cellular stress and enhance cell survival during thermotolerance [Citation27], while transcription of HSP70 and HSP90 could serve as successful indicators of stress responses [Citation27,Citation28]. For example, Hsp70 has been reported to be important for cytoprotective functions in gastric mucosa, and its overexpression was able to protect against heat stress [Citation29]. Similarly, Hsp90, which functions as a multiprotein chaperone complex together with Hsp70, has been demonstrated to be involved in cell-cycle regulation, signal-transduction and apoptotic pathways [Citation27]. In our study, after heat shock, the increased expression by HSP70 and HSP90 indicated that IEC-6 cells were in heat shock-induced stress. Presumably, the induction of HSPs help cells survive the overheated environment. Heat shock altered the morphology of IEC-6 cells, and PA regulated the morphology of IEC-6 cells back to normal. Because HSP expression depends on the severity of lesions [Citation27], the decreased mRNA expression by HSP70 and HSP90 in PA pretreatment groups showed that PA pretreatment had weakened the heat shock responses of IEC-6 cells, but it should not disregard the cytoprotective functions of HSP70 and HSP90.
In this study the increased ROS production and MDA content revealed that IEC-6 cells were in oxidative stress after they were exposed to high temperature. Though pretreatment with PA at 80ng/mL increased ROS production, the decreased MDA content in PA groups showed that PA alleviated the oxidative stress status of IEC-6 cells. The mRNA expression by SOD, CAT and GSH-px in PA groups was decreased, revealing PA did not protect cells from oxidative stress by increasing the mRNA expression of antioxidants. PA significantly decreased the mRNA expression by SOD, which is an ROS scavenger, suggesting the ROS scavenging abilities in PA pretreatment groups were reduced. It can explain why pretreatment with PA at 80 ng/mL significantly increased the generation of intracellular ROS, and it is inferred that maybe only after ROS was generated can PA enact the protection of scavenging free radical.
The Nrf2-keap1 pathway is regarded as one of the most important signal transduction pathways to protect against oxidative stress, and it also plays a critical role in cellular protection and cell survival [Citation9]. Agonists of the pathway, such as curcumin, can effectively attenuate oxidative stress by modulating the Nrf2-Keap1 pathway [Citation30] and inducing the expression of a battery of defensive genes encoding detoxifying enzymes and antioxidant proteins. Normally, Nrf2 is retained in the cytoplasm by Keap1, but in response to oxidative stress Nrf2 is ’switched on’ and translocates to the nucleus, where it binds the ARE and activates expression of its target genes [Citation9,Citation31]. A previous study reported that heat shock induced oxidative stress in mouse testes, indicated by Nrf2 nuclear accumulation [Citation8]. HO-1, a well-characterised Nrf2 target gene, has the ability to stimulate cell growth and proliferation, and is able to protect cells from harmful oxidative stress [Citation32].
In this study, heat shock significantly increased Nrf2 expression at both the mRNA and the protein level, and increased HO-1 mRNA expression, suggesting that Nrf2 and HO-1 were critical to heat-induced oxidative stress. Compared with the heat shock group, PA increased Nrf2 protein expression in a dose-dependent manner, and significantly increased Nrf2 protein expression at the high dose. The middle and high doses of PA resulted in a significant increase in HO-1 protein expression, indicating that PA protected IEC-6 cells from oxidative stress by increasing Nrf2 and HO-1 protein expression, further demonstrating that the Nrf2-Keap1 pathway was critical for PA to regulate oxidative stress responses. Keap1 inhibits Nrf2 by retaining it in the cytoplasm, so the down-regulation of KEAP1 mRNA expression in the PA pretreatment groups may be helpful for Nrf2 to translocate to the nucleus. In addition, heat shock increased HO-1 mRNA expression, but HO-1 protein expression did not change for unknown reasons. The down-regulation of HO-1 mRNA expression in response to PA reflected the weakened oxidative stress response of IEC-6 cells; however, PA increased HO-1 protein expression in these cells, indicating that there might be a feedback mechanism to maintain homeostasis.
Heat shock caused oxidative stress response of IEC-6 cells by increasing the generation of intracellular ROS, MDA, the mRNA expression by GSH-px, NRF2 and HO-1, and the protein expression of Nrf2 and Keap1. Pretreatment with PA at 10 ng/mL and 40 ng/mL can return the oxidative stress status of IEC-6 cells by decreasing the MDA content and up-regulating the protein expression of HO-1. Though pretreatment with PA at 80 ng/mL significantly increased the generation of intracellular ROS, which may cause more severe oxidative stress, the antioxidant responses were also enhanced by up-regulating the protein expression of Nrf2 and HO-1. Pretreatment with PA at 80 ng/mL increased the generation of intracellular ROS by decreasing the mRNA expression of antioxidants, but it still can alleviate the oxidative stress status of IEC-6 cells by activating the Nrf2-Keap1 pathway. To avoid the damage of increased ROS production caused by the high dose of PA (80 ng/mL), the proper dose of PA pretreatment is 10–40 ng/mL for IEC-6 cells.
Conclusion
Heat shock caused oxidative stress of IEC-6 cells, and PA modulated the morphology and alleviated the oxidative stress responses of IEC-6 cells. The potential mechanism for PA’s protection of IEC-6 cells was due to increased Nrf2 and HO-1 protein expression. Thus, PA reduced the oxidative stress response in IEC-6 cells by activating the Nrf2-Keap1 pathway.
Acknowledgements
We are thankful for the help from the members of the CAU-BUA TCVM teaching and research team.
Disclosure statement
This work was supported by grants from the Ministry of Agriculture, Public Service Sectors Agriculture Research Projects (No. 201403051-07), the National Natural Science Foundation of China (No. 31272478, 31502025), the National Twelve-Five Technological Supported Plan of China (No. 2011BAD34B01, 2013BAD10B04), and the Importation and Development of High-Caliber Talents Project of Beijing Municipal Institutions (CIT & TCD20130324). The authors alone are responsible for the content and production of this manuscript.
References
- Williams JM, Duckworth CA, Burkitt MD, Watson AJ, Campbell BJ, Pritchard DM. pithelial cell shedding and barrier function: A matter of life and death at the small intestinal villus tip. Vet Pathol 2015;52:445–55.
- Circu ML, Aw TY. Intestinal redox biology and oxidative stress. Semin Cell Dev Biol 2012;23:729–37.
- Liu X, Li H, Lu A, Zhong Y, Hou X, Wang N, et al. Reduction of intestinal mucosal immune function in heat-stressed rats and bacterial translocation. Int J Hyperthermia 2012;28:756–65.
- Yu J, Liu F, Yin P, Zhao H, Luan W, Hou X, et al. Involvement of oxidative stress and mitogen-activated protein kinase signaling pathways in heat stress-induced injury in the rat small intestine. Stress 2013;16:99–113.
- Niederlechner S, Baird C, Petrie B, Wischmeyer E, Wischmeyer PE. Epidermal growth factor receptor expression and signaling are essential in glutamine's cytoprotective mechanism in heat-stressed intestinal epithelial-6 cells. Am J Physiol Gastrointest Liver Physiol 2013; 304:G543–52.
- Yu J, Yin P, Yin J, Liu F, Zhu X, Cheng G, et al. Involvement of ERK1/2 signalling and growth-related molecules’ expression in response to heat stress-induced damage in rat jejunum and IEC-6 cells. Int J Hyperthermia 2010;26:538–55.
- Oliver SR, Phillips NA, Novosad VL, Bakos MP, Talbert EE, Clanton TL. Hyperthermia induces injury to the intestinal mucosa in the mouse: Evidence for an oxidative stress mechanism. Am J Physiol Regul Integr Comp Physiol 2012;302:R845–53.
- Li Y, Huang Y, Piao Y, Nagaoka K, Watanabe G, Taya K, et al. Protective effects of nuclear factor erythroid 2-related factor 2 on whole body heat stress-induced oxidative damage in the mouse testis. Reprod Biol Endocrinol 2013;11:23.
- Kaspar JW, Niture SK, Jaiswal AK. Nrf2:INrf2 (Keap1) signaling in oxidative stress. Free Radic Biol Med 2009;47:1304–9.
- Pizarro JG, Folch J, Vazquez De la Torre A, Verdaguer E, Junyent F, Jordan J, et al. Oxidative stress-induced DNA damage and cell cycle regulation in B65 dopaminergic cell line. Free Radic Res 2009;43:985–94.
- Thannickal VJ, Fanburg BL. Reactive oxygen species in cell signaling. Am J Physiol Lung Cell Mol Physiol 2000;279:L1005–28.
- Jones DP. Redefining oxidative stress. Antioxid Redox Signal 2006;8:1865–79.
- Xu X, Li H, Hou X, Li D, He S, Wan C, et al. Punicalagin induces Nrf2/HO-1 expression via upregulation of PI3K/AKT pathway and inhibits LPS-induced oxidative stress in RAW264.7 macrophages. Mediators Inflamm 2015;2015:380218.
- Fang J, Seki T, Maeda H. Therapeutic strategies by modulating oxygen stress in cancer and inflammation. Adv Drug Deliv Rev 2009;61:290–302.
- Giordano FJ. Oxygen, oxidative stress, hypoxia, and heart failure. J Clin Invest 2005;115:500–8.
- Johnson JA, Johnson DA, Kraft AD, Calkins MJ, Jakel RJ, Vargas MR, et al. The Nrf2-ARE pathway: An indicator and modulator of oxidative stress in neurodegeneration. Ann N Y Acad Sci 2008;1147:61–9.
- Davey MW, Stals E, Panis B, Keulemans J, Swennen RL. High-throughput determination of malondialdehyde in plant tissues. Anal Biochem 2005;347:201–7.
- Del Rio D, Stewart AJ, Pellegrini N. A review of recent studies on malondialdehyde as toxic molecule and biological marker of oxidative stress. Nutr Metab Cardiovasc Dis 2005;15:316–28.
- Chelikani P, Fita I, Loewen PC. Diversity of structures and properties among catalases. Cell Mol Life Sci 2004;61:192–208.
- Zhang R, Yan P, Li Y, Xiong L, Gong X, Peng C. A pharmacokinetic study of patchouli alcohol after a single oral administration of patchouli alcohol or patchouli oil in rats. Eur J Drug Metab Pharmacokinet 2015. PMID 25753831.
- Zheng YF, Xie JH, Xu YF, Liang YZ, Mo ZZ, Jiang WW, et al. Gastroprotective effect and mechanism of patchouli alcohol against ethanol, indomethacin and stress-induced ulcer in rats. Chem Biol Interact 2014;222C:27–36.
- Yu XD, Xie JH, Wang YH, Li YC, Mo ZZ, Zheng YF, et al. Selective antibacterial activity of patchouli alcohol against Helicobacter pylori based on inhibition of urease. Phytother Res 2015;29:67–72.
- Li YC, Xian YF, Ip SP, Su ZR, Su JY, He JJ, et al. Anti-inflammatory activity of patchouli alcohol isolated from Pogostemonis herba in animal models. Fitoterapia 2011;82:1295–301.
- Li YC, Peng SZ, Chen HM, Zhang FX, Xu PP, Xie JH, et al. Oral administration of patchouli alcohol isolated from Pogostemonis herba augments protection against influenza viral infection in mice. Int Immunopharmacol 2012;12:294–301.
- Xian YF, Li YC, Ip SP, Lin ZX, Lai XP, Su ZR. Anti-inflammatory effect of patchouli alcohol isolated from Pogostemonis herba in LPS-stimulated RAW264.7 macrophages. Exp Ther Med 2011;2:545–50.
- Feng XX, Yu XT, Li WJ, Kong SZ, Liu YH, Zhang X, et al. Effects of topical application of patchouli alcohol on the UV-induced skin photoaging in mice. Eur J Pharm Sci 2014;63:113–23.
- Otaka M, Odashima M, Watanabe S. Role of heat shock proteins (molecular chaperones) in intestinal mucosal protection. Biochem Biophys Res Commun 2006;348:1–5.
- Liu X, Shi Y, Hou X, Wan C, He S, Chong X, et al. Microarray analysis of intestinal immune-related gene expression in heat-stressed rats. Int J Hyperthermia 2014;30:324–27.
- Gupta S, Deepti A, Deegan S, Lisbona F, Hetz C, Samali A. HSP72 protects cells from ER stress-induced apoptosis via enhancement of IRE1 alpha-XBP1 signaling through a physical interaction. PLoS Biology 2010;8:e1000410.
- Soetikno V, Sari FR, Lakshmanan AP, Arumugam S, Harima M, Suzuki K, et al. Curcumin alleviates oxidative stress, inflammation, and renal fibrosis in remnant kidney through the Nrf2-keap1 pathway. Mol Nutr Food Res 2013;57:1649–59.
- Bryan HK, Olayanju A, Goldring CE, Park BK. The Nrf2 cell defence pathway: Keap1-dependent and -independent mechanisms of regulation. Biochem Pharmacol 2013;85:705–17.
- Alam J, Stewart D, Touchard C, Boinapally S, Choi AM, Cook JL. Nrf2, a Cap’n’Collar transcription factor, regulates induction of the heme oxygenase-1 gene. J Biol Chem 1999;274:26071–8.