Abstract
Purpose: The aim of this study was to evaluate the effect of different radio-frequency ablation (RFA) thermal doses on coagulation and heat shock protein (HSP) response with and without adjuvant nanotherapies.
Materials and methods: First, Fischer rats were assigned to nine different thermal doses of hepatic RFA (50–90 °C, 2–20 min, three per group) or no treatment (n = 3). Next, five of these RF thermal doses were combined with liposomal-doxorubicin (Lipo-Dox, 1 mg intravenously) in R3230 breast tumours, or no tumour treatment (five per group). Finally, RFA/Lipo-Dox was given without and with an Hsp70 inhibitor, micellar quercetin (Mic-Qu, 0.3 mg intravenously) for two different RFA doses with similar coagulation but differing peri-ablational Hsp70 (RFA/Lipo-Dox at 70 °C × 5 min and 90 °C × 2 min, single tumours, five per group). All animals were sacrificed 24 h post-RFA and gross tissue coagulation and Hsp70 (maximum rim thickness and % cell positivity) were correlated to thermal dose including cumulative equivalent minutes at 43 °C (CEM43).
Results: Incremental increases in thermal dose (CEM43) correlated to increasing liver tissue coagulation (R2 = 0.7), but not with peri-ablational Hsp70 expression (R2 = 0.14). Similarly, increasing thermal dose correlated to increasing R3230 tumour coagulation for RF alone and RFA/Lipo-Dox (R2 = 0.7 for both). The addition of Lipo-Dox better correlated to increasing Hsp70 expression compared to RFA alone (RFA: R2 = 0.4, RFA/Lipo-Dox: R2 = 0.7). Finally, addition of Mic-Qu to two thermal doses combined with Lipo-Dox resulted in greater tumour coagulation (p < 0.0003) for RFA at 90 °C × 2 min (i.e. greater baseline Hsp70 expression) than an RFA dose that produced similar coagulation but less HSP expression (p < 0.0004).
Conclusion: Adjuvant intravenous Lipo-Dox increases peri-ablational Hsp70 expression in a thermally dependent manner. Such expression can be exploited to produce greater tumour destruction when adding a second adjuvant nanodrug (Mic-Qu) to suppress peri-ablational HSP expression.
Introduction
Currently, radio-frequency ablation (RFA) is used to treat a wide range of focal tumours in various organs [Citation1–5], based upon its inherent minimally invasive nature, low morbidity rate, economic feasibility and applicability in a wider cohort of non-surgical patients [Citation6,Citation7]. However, while some long-term studies have demonstrated comparable outcomes to surgery [Citation8,Citation9], there are many reports of incomplete treatment, especially for larger tumours (>3 cm) [Citation10,Citation11], and higher rates of local recurrence even for smaller tumours in some studies [Citation12]. Residual viable tumour may be due in part to various (and as of yet, incompletely characterised) heating–tissue interactions that are occurring in the peri-ablational rim of tumour/tissue that is exposed to non-lethal hyperthermia.
One strategy to address these critical limitations of RFA efficacy has been to study the tissue changes that occur in the peri-ablational rim, and based upon this understanding, combine RFA with adjuvant therapies targeting specific processes, maximising RF-induced tumour injury. For example, elucidation of hyperthermia-induced microvascular changes and increased vessel permeability in the peri-ablational rim [Citation13] has led directly to the successful combination of RFA with Lipo-Dox to increase targeted local drug delivery to tumours incompletely treated with ablation [Citation14]. Characterisation studies of this combination RF/Doxil paradigm demonstrated increases in tumour coagulation, animal end-point survival, and intratumoural drug accumulation [Citation15–17]. Similarly, characterisation of apoptotic changes in the peri-ablational rim, and reactive and protective heat shock protein expression (Hsp70) have led to studies targeting these pathways as well as to increased RF-induced tumour injury and animal end-point survival [Citation18,Citation19].
Whereas most of the studies combining RF with nanodrugs have used a single standardised RF application, it has been clearly demonstrated that variation in the amount of tissue heating (i.e. thermal dose) affects ablation zone size in normal liver [Citation20,Citation21]. Indeed, preliminary results from the recently concluded HEAT trial using hepatic RFA combined with thermosensitive liposomes for large hepatocellular carcinoma suggests that longer heating times may support higher local drug delivery [Citation22]. Thus, here, we study how varying RFA parameters (i.e. thermal dose) affects both coagulation and the peri-ablational tissue response to non-lethal heating (i.e. heat shock protein expression) in a small animal tumour model. We then study how varying thermal dose affects coagulation and tissue responses when combined with adjuvant nanoparticle chemotherapies. Accordingly, the purpose of this study is to determine the influence of variable thermal doses on coagulation and expression of Hsp70 in the peri-ablational rim, and to characterise the modulatory effect of adjuvant intravenous nanodrugs (Lipo-Dox and Mic-Qu) on Hsp70 expression elicited by variable thermal doses.
Materials and methods
Experimental overview
Approval of the Institutional Animal Care and Use Committee was obtained prior to the start of this study. The study was performed in three phases to systematically investigate the potential effects of variable thermal doses of RFA with and without adjuvant nanodrugs on tissue coagulation and Hsp70 expression (). In the sections below, ‘thermal dose’ refers to the dose achieved at the tip of the RF electrode as 1) a function of ablation time (minutes) and electrode tip temperature (°C), and 2) the calculated corresponding cumulative equivalent minutes (CEM43), as described by Sapareto and Dewey [Citation23]. Also, references to ‘doxorubicin’ and ‘quercetin’ imply liposomal and micellar encapsulations respectively, as these were the sole methods of drug administration throughout the study.
Figure 1. Flow chart representing the study design. Briefly, 105 rats were randomised into three experiments. Phase I examines the effect of variable thermal doses on liver coagulation and Hsp70 expression. Phase II examines the effect of variable thermal doses on tumour coagulation and Hsp70 expression, without and with the use of adjuvant intravenous liposomal doxorubicin. Finally, phase III studies the modulatory effect of micellar quercetin when added to combination therapies of RFA and adjuvant intravenous liposomal doxorubicin. gp/gps, group/groups; IHC, immunohistochemistry; IV, intravenous; Lipo-Dox, liposomal doxorubicin, Mic-qu, micellar quercetin, RFA radio-frequency ablation,
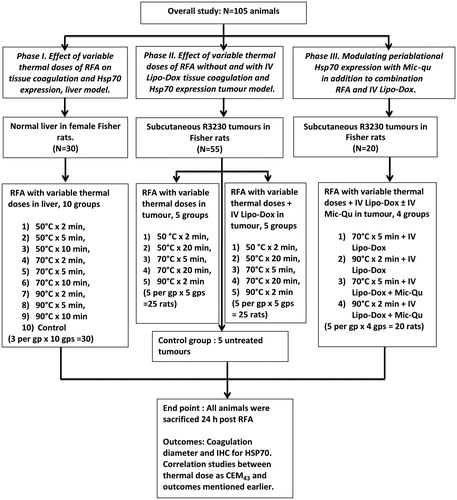
Phase I: effect of variable thermal doses of RFA on tissue coagulation and Hsp70 expression in an animal liver model
Thirty Fisher 344 female rats (without tumours) were randomised into nine treatment groups for RFA of normal liver with variable thermal doses (50 °C × 2 min, 50 °C × 5 min, 50 °C × 10 min, 70 °C × 2 min, 70 °C × 5 min, 70 °C × 10 min, 90 °C × 2 min, 90 °C × 5 min, 90 °C × 10 min, three per group, total 27) or no treatment (control livers, n = 3). Animals were sacrificed and tissues harvested 24 h post-treatment. Outcome measures included gross pathological assessment for coagulation size and immunohistochemistry for Hsp70 (a marker of sub-lethal thermal injury [Citation24]), plotted to thermal dose (CEM43).
Phase II: effect of variable thermal doses of RFA without and with adjuvant intravenous Lipo-Dox on tissue coagulation and Hsp70 expression in a small animal tumour model
Here, single subcutaneous R3230 breast adenocarcinoma tumours (total 55 tumours in Fischer 344 rats) were randomised into treatment arms of RFA without and with adjuvant intravenous Lipo-Dox (1 mg, 0.5 mL intravenous injection administered 15 min post-RFA) with five variable thermal doses (50 °C × 2 min, 50 °C × 20 min, 70 °C × 5 min, 70 °C × 20 min, 90 °C × 2 min, five per group, n = 50) or no treatment (control tumours, n = 5). Animals were sacrificed and tissues harvested 24 h post-treatment with outcome measures similar to those described for phase I.
Phase III: modulating peri-ablational Hsp70 expression with adjuvant Mic-Qu in addition to combination RFA and intravenous Lipo-Dox
Animals with single subcutaneous R3230 breast adenocarcinoma tumours were randomised into four treatment groups (five in each, n = 20). Groups 1 and 2 received intravenous Lipo-Dox with tumour RFA using two different thermal doses (70 °C × 5 min and 90 °C × 2 min, respectively). Groups 3 and 4 received both intravenous Lipo-Dox and intravenous Mic-Qu (0.3 mg, 0.5 mL intravenous injection administered 15 min post-RFA) combined with tumour RFA using the same two thermal doses (70 °C × 5 min and 90 °C × 2 min). Animals were sacrificed and tumours harvested 24 h post-treatment. Outcome measures were similar to those described earlier.
Animal models
For all experiments and procedures, anaesthesia was induced with intraperitoneal injection of a mixture of ketamine (50 mg/kg Ketaject, Phoenix Pharmaceutical, St Joseph, MO, USA) and xylazine (5 mg/kg, Bayer, Shawnee Mission, KS). Animals were sacrificed with an overdose (1.5 mL/kg) of a mixture of ketamine and xylazine.
Experiments were performed upon two animal models. The first model was normal liver of Fisher 344 female rats (150 ± 20 g, 14–16 weeks old, Charles River, Wilmington, MA). After administering anaesthesia, the rat’s abdomen was prepped with Betadine and alcohol, and shaved. A mini-laparotomy was achieved by a 1.5-cm subcostal incision exposing the right lobe of the liver. After RFA, the abdomen was closed in layers using interrupted sutures.
The second model was a well-characterised established R3230 mammary adenocarcinoma cell line used in multiple prior studies [Citation19,Citation25]. Female Fisher 344 rats (150 ± 20 g, 14–16 weeks old, Charles River) were used in this study. Tumour implantation, evaluation, and preparation techniques were performed as previously described [Citation19,Citation25]. Briefly, one tumour was implanted into each rat by slowly injecting 0.3–0.4 mL of tumour suspension into the mammary fat pad of each rat via an 18-gauge needle. Solid non-necrotic tumours 1.3–1.6 cm were used for this study, randomised to different treatment arms.
RF application
Conventional monopolar RFA was applied by using a 500-kHz RFA generator (model CC-1, Radionics, Burlington, MA), as has been previously described [Citation19,Citation20]. Initially, the 1-cm tip of a 21-gauge electrically insulated electrode (SMK electrode, Radionics) was placed at the centre of the tumour. For livers, after exposure and visualisation of the right lobe, the probe was inserted into the liver with its long axis parallel to the long axis of the lobe. Complete insertion into the liver was confirmed visually before performing RFA. For each thermal dose, RF was applied for a predetermined number of minutes with the generator output titrated to maintain a designated tip temperature. To complete the RF circuit, the rat’s back was shaved and water-soluble ultrasound gel was applied to ensure proper conduction with the metallic grounding pad (Radionics).
Preparation and administration of adjuvant intravenous liposomal agents
For liposomal doxorubicin, a commercially-available preparation (Doxil, 2 mg/mL, ALZA Pharmaceuticals, Palo Alto, CA) was used. Quercetin-loaded micelles were prepared by the lipid film hydration method. Briefly, 0.6 mg of quercetin (1 mg/mL solution in methanol) was added to polyethylene glycol phosphatidyl ethanolamine (PEG2000-PE) solution in chloroform, and a lipid film was formed in a round-bottomed flask by solvent removal on a rotary evaporator. The lipid film was then rehydrated with 1 mL of phosphate-buffered saline, pH 7.4, to obtain final lipid concentration of 5 mM. The sample was vortexed for 15 min at room temperature and the unincorporated quercetin was removed by filtration of the micelle suspension with 0.2 μm membrane filters. The micellar loading efficiency of quercetin was 100% (as noted above, 0.6 mg of quercetin was loaded in each mL). The micelle size was 17.0 ± 2.1 nm and zeta-potential was −21.7 ± 4.3 mV.
Adjuvant Lipo-Dox and Mic-Qu were both administered via tail vein intravenous injection 15 min post-RFA. This timing of administration was selected based upon prior studies combining RFA with nanodrugs demonstrating maximum tumour coagulation and intratumoural drug accumulation with this regimen [Citation18,Citation19]. When two agents (i.e. Lipo-Dox and Mic-Qu) were administered at the same time point, both agents were loaded in the same syringe to ensure simultaneous administration and minimise potential variability from varying temporal administration. Agents were stored at 4 °C in light-proof containers and loaded exactly at time of administration to avoid precipitation.
Pathological evaluation
Animals were sacrificed and tissue harvested. Livers and tumours were sectioned perpendicularly to the direction of electrode insertion. The relevant portion of the liver or one half of the tumour containing the central section of tumour was fixed in 10% formalin overnight at 4 °C, embedded in paraffin, and sectioned at a thickness of 5 μm. Tissues were stained with haematoxylin and eosin for histopathological assessment. The second half of the tumour was used to determine gross RFA-induced tissue coagulation using cell viability staining (10–15 min incubation in 1 mg 2,3,5 tetrazolium chloride in 50 mL PBS, Sigma, Chicago, IL) [Citation14].
The area of white coagulation was identified and measured using manual calipers (maximum dimension and shorter perpendicular dimension).
Immunohistochemical staining and microscopy
A Hsp70 mouse antibody (Cell Signaling Technology, Danvers, MA) was used to identify Hsp70 expression using previously described techniques [Citation18,Citation19]. Briefly, 5 μm thick paraffin sections were freshly cut; slides were left to bake at 60 °C overnight in a dedicated deparaffinising oven, after which heat mediated antigen retrieval was performed using citrate buffer in a pressure cooker. After application of a hydrogen peroxidase block, the primary antibody was applied for 1 h at room temperature followed by application of the secondary antibody. Finally, slides were developed using diaminobenzidine and a coverslip applied for imaging.
Specimen slides were imaged and analysed using a Micromaster I microscope (Fisher Scientific, Pittsburgh, PA) and Micron Imaging Software (Westover Scientific, Mill Creek, WA). Images were taken at 10× and 40× magnification and HSP expression was evaluated for mean peri-ablational rim thickness, and percentage cell positivity, respectively. In each case, five random high-power fields were analysed for a minimum of three specimens for each parameter and scored in a blinded fashion to remove observer bias. Mean rim thickness was determined using the mean of three representative values per specimen. As an additional control to ensure uniformity of staining, whenever direct comparisons were made, immunohistochemistry was repeated with all relevant comparison slides stained at the same time.
CEM43 calculation
To standardise and quantify administered thermal doses, we used the model reported by Sapareto and Dewey, and adopted by others [Citation23,Citation26,Citation27], which translates the time–temperature history of the RF probe tip into a numerical value of CEM43. The following equation was used:
where CEM43 represents the total amount of equivalent minutes at 43 °C, ti denotes the time interval, R is related to the temperature dependence of the rate of cell death, which has a value of 0.5 when Ti is more than 43 °C and Ti is the average temperature during ti.
Statistical analysis
The Microsoft Excel 2007 software package (Redmond, WA) was used for statistical analysis. All data were provided as mean plus or minus SD. Immunohistochemistry results were compared using analysis of variance (ANOVA). Additional post-hoc analysis was performed with paired, two-tailed Student’s t-test, if and only if, the analysis of variance achieved statistical significance. A p-value of less than 0.05 was considered significant.
Results
Phase I: effect of variable RFA thermal doses on tissue coagulation and Hsp70 expression in normal liver
In normal liver, increasing the administered thermal dose (CEM43) demonstrated a positive correlation with achieved coagulation diameter (R2 = 0.7, ). Thus, higher thermal doses induced significantly larger coagulation zones when compared to lower doses (P < 0.05). This was evident when maximising either tip temperature or time of ablation (, ). For example, liver RFA at 50 °C × 2 min resulted in no visible coagulation compared to a mean coagulation of 6.0 ± 0.7 mm or 1.9 ± 1.6 mm, when maximising temperature to 90 °C or time of application to 10 min, respectively (P < 0.05 and P < 0.02).
Figure 2. Correlation between varied RF thermal doses (CEM43 °C or tip temperature) and, RF-induced coagulation diameters and peri-ablational Hsp70 expression in normal liver. (A) There is a positive correlation (R2 = 0.7) between the log of thermal dose applied during RFA (CEM43 °C) and coagulation diameter in normal liver. (B) Increasing thermal doses (tip temperature and/or duration of application) induced significantly more coagulation when compared to their lower counter parts, p < 0.05. For example at any constant application duration, incremental increase of temperature from 50° to 90 °C resulted in significant in coagulation, p < 0.05. Similarly incremental increase in time from 2 to 10 min at any constant tip temperatures resulted in significant gains in coagulation, p < 0.02. (C) In contrast to coagulation zone, the log of CEM43 °C as a measure of thermal dose had only a weak correlation (to RF-induced peri-ablational Hsp70 expression in normal rat liver (R2 = 0.14).
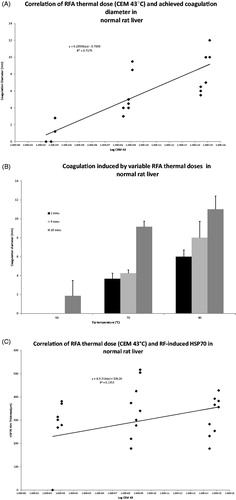
Table 1. RFA diameters for varied application times and tip temperatures. At constant RF application periods, incremental increase of temperature from 50–90 °C resulted in significant coagulation gains, p < 0.05. Similarly, incremental increase in time at constant tip temperatures resulted in significant gains in coagulation, p < 0.02.
In normal liver, Hsp70 expression did not correlate well with thermal dose (when plotting increasing logarithm of CEM43 values against respective Hsp70 rim thicknesses (R2 = 0.14) (). Significant increases in Hsp70 expression were detected when increasing RF application time for any given temperature (p < 0.0008), as opposed to increasing tip temperature, which had no appreciable effect (p = 0.14). For example, incrementally increasing RF time from 2 to 10 min at constant temperatures (50 °C, 70 °C and 90 °C) resulted in CEM43 values that correlated with Hsp70 rim thicknesses (R2 = 0.87, 0.5, 0.6, respectively). However, CEM43 for increasing tip temperatures (i.e. 50 °C to 90 °C) at constant application duration (2, 5 or 10 min) had a much weaker (if any) correlation to Hsp70 rim thicknesses (R2 = 0.5, 0.09, 0.06, respectively, , ). Thus, increasing liver RFA time at 50 °C or 90 °C from 2 min to 10 min increased peri-ablational Hsp70 rim thickness from 0 ± 0 μm to 230.9 ± 52.5 μm versus 344.1 ± 56.3 μm to 389.1 ± 56.3 μm, respectively (p < 0.0001 and p < 0.02). Conversely, increasing tip temperature from 50 °C to 90 °C for RFA durations of 5 and 10 min did not increase peri-ablational Hsp70 rim thickness (p = 0.4, ). Finally, Hsp70 percentage cell positivity was not significantly increased when applying increasing thermal doses of RFA (p = 0.07, ).
Figure 3. Comparison of RF-induced peri-ablational Hsp70 in normal rat liver for varied RF application. Magnified images (10×) of liver peri-ablational rims stained for Hsp70 expression after 2 min (A), 5 min, (B), and 10 min (C) for a standardised RF tip temperature (90 °C) demonstrates a significant increase in rim thickness when increasing duration of RF application (p < 0.003). By contrast, incremental increase of tip temperature at any constant application period did not result in significant increases in Hsp70 expression (p < 0.4).
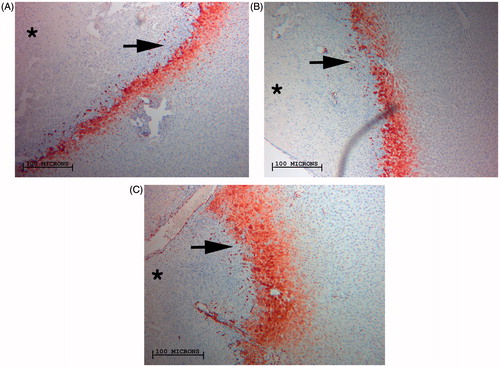
Table 2. Hsp70 expression in the peri-ablational rim for varied RF application protocols. Hsp70 rim thickness demonstrated a positive correlative relation with increased RF duration of application at any set temperature (50 °C, 70 °C and 90 °C R2 = 0.87, 0.5, 0.6, respectively). However, increased tip temperature achieved no tangible gains in Hsp70 rim thickness.
Phase II: effect of variable RFA thermal doses without and with adjuvant intravenous Lipo-Dox on tissue coagulation and Hsp70 expression in small animal tumours
For RFA alone of R3230 tumours, a positive correlation (R2 = 0.7) was observed between thermal dose (CEM43) and coagulation (). Maximising tip temperature and/or increasing duration of application resulted in significantly larger coagulation zones when compared to corresponding lower thermal dose, p < 0.02 (). For example, tumour tissue ablated with a dose of 50 °C × 2 min resulted in 3.2 ± 0.7 mm of coagulation. At this temperature, coagulation zones increased to 5.2 ± 0.5 mm when duration was increased to 20 min and 12.4 ± 0.4 mm when temperature was increased to 90 °C, respectively (p < 0.0001). For RFA alone in R3230 tumours, peri-ablational Hsp70 rim thickness correlated with administered thermal dose CEM43 values to R2 = 0.4 (). Hsp70 rim thickness increased significantly when either tip temperature or application duration was increased. For example, increasing RFA from 50 °C × 2 min to 90 °C × 2 min increased Hsp70 rim thickness (from 691.3 ± 140.1 μm to 1030.5 ± 176.9 μm, p < 0.02, and ). Similarly, increased RF application time at higher tip temperatures (≥ 70 °C) also increased tumour peri-ablational Hsp70 rim thickness, as observed when increasing the application time at 70 °C from 5 min to 20 min (HSP rim thickness 687.5 ± 104.5 μm versus 1300.4 ± 123.3 μm, P < 0.001, and , ). By contrast, increasing RFA application time at lower tip temperatures that produced limited ablation (i.e. 50 °C) did not result in significant Hsp70 increases. Finally, similar to liver RFA (phase I), Hsp70 percentage cell positivity did not demonstrate any significant increase for variable thermal doses of RFA (p = 0.09).
Figure 4. Correlation between varied RF thermal doses (CEM43 °C) and RF induced coagulation diameters and peri-ablational Hsp70 expression in R3230 adenocarcinoma without and with adjuvant liposomal doxorubicin. (A) Increasing thermal dose, when presented as log of CEM43 °C, demonstrates a strong positive correlation with achieved RF induced coagulation in R3230 adenocarcinoma, R2 = 0.7. (B) Graphical representation of increasing thermal dose, presented as CEM43 °C log, demonstrating weak correlation with respective RF-induced Hsp70 rim thicknesses (R2 = 0.4). (C) After addition of adjuvant liposomal doxorubicin to RFA, the achieved coagulation zones in R3230 tumours continue to correlate positively with thermal doses, when plotted as log CEM43 °C values (R2 = 0.7). (D) Following the addition of adjuvant intravenous liposomal doxorubicin, overall correlation of RF-induced Hsp70 and thermal dose as calculated CEM43 °C values improves from 0.4 to 0.7.
Figure 5. Comparison of RFA-induced peri-ablational Hsp70 for varied RF applications: four images of R3230 tumour peri-ablational rims. Images demonstrate significant increase of Hsp70 expression when RF tip temperature is maximised from 50 °C (A) to 90 °C (B) for a constant period of application, 2 min. Significant Hsp70 gains are when RF application duration is maximised from 5 min (C) to 20 min (D) at a set tip temperature, 70 °C.
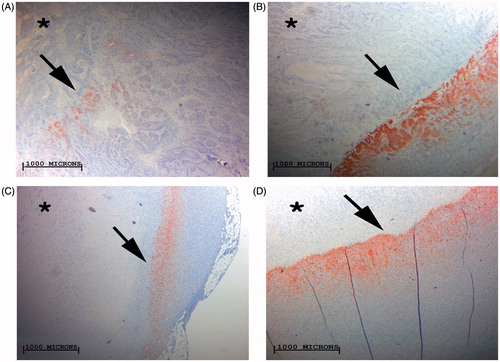
Table 3. RF coagulation zone induced diameters in R3230 tumours are dependent on administered thermal dose whether combined with adjuvant intravenous liposomal doxorubicin or not. Maximising duration of RF application from 50 °C × 2 min to 50 °C × 20 min or from 70 °C × 5 min to 70 °C × 20 min whether RF alone or combined with doxorubicin has significantly increased coagulation gains, p < 0.05 for all comparisons. Similarly, maximising tip temperature to 90 °C has resulted in significant coagulation gains whether RF is combined with doxorubicin or not, p < 0.001.
Comparison of tissues
Tissue-specific differences were also observed when comparing RFA of normal liver alone (from phase I) to R3230 tumour. For the same increase in thermal dose, RFA of the R3230 tumour model led to greater increases in coagulation diameter compared to RFA of normal liver. For example, for RFA of 90 °C × 2 min, significantly greater coagulation was observed in R3230 tumour tissue compared to normal liver tissue (12.3 ± 1.4 mm and 6.0 ± 0.7 mm, respectively, p < 0.003). Similarly, greater peri-ablational Hsp70 expression was observed in tumour tissue compared to normal liver tissue for identical RFA thermal doses. For example, no Hsp70 up-regulation was observed after RFA of 50 °C × 2 min for normal liver, compared to an Hsp70 rim thickness of 691.3 ± 140.1 μm for the R3230 tumour at the same thermal dose. Similarly, peri-ablational Hsp70 rim thickness was significantly increased for RFA of 90 °C × 2 min in R3230 tumour tissue compared to normal liver (399.2 ± 36.8 μm versus 231.1 ± 46.9 μm, p < 0.0002).
Combination of therapies
Overall, combining Lip-Dox with RFA in R3230 tumours resulted in significantly larger ablation zones and Hsp70 expression when compared to RF alone groups, as previously reported [Citation10]. CEM43 values for combined RFA/Lipo-Dox again correlated with tumour coagulation (R2 = 0.7, ). Overall, improved correlation between peri-ablational Hsp70 rim thickness and administered thermal dose was noted with the addition of adjuvant intravenous Lipo-Dox (R2 = 0.7, ). However, for different thermal doses, variable, and non-concordant gains in Hsp70 rim thickness and/or coagulation were observed on the addition of Lipo-Dox (). Accordingly, this led to the identification of two distinct thermal doses 70 °C × 5 min and 90 °C × 2 min, for which combined RFA/Lipo-Dox resulted in equal tumour coagulation (mean diameters 12.3 ± 0.4 mm and 12.3 ± 1.2 mm, respectively, p = 1.0), but significantly different peri-ablational Hsp70 rim thicknesses (mean 1189.1 ± 147.6 μm and 1467.2 ± 317.0 μm, respectively, p < 0.03). These two thermal dose and combination RFA/Lipo-Dox groups were selected for use in phase 3.
Phase III: modulating peri-ablational Hsp70 expression with adjuvant micellar quercetin in addition to combination RFA and intravenous Lipo-Dox
The control group of RFA/Lipo-Dox alone treated with either 70 °C × 5 min and 90 °C × 2 min (i.e. groups 1 and 2) again resulted in equal tumour coagulation with significantly different mean Hsp70 rim thicknesses (644.8 ± 39.8 μm and 1030.1 ± 192.0 μm, respectively, p < 0.01). The addition of a second nanodrug, micellar quercetin (RFA/Lipo-Dox/Mic-Qu) resulted in significant increases in the coagulation zone at both thermal doses of 70 °C × 5 min and 90 °C × 2 min. However, when Mic-Qu was added to RFA/Lipo-Dox at 90 °C × 2 min (i.e. the thermal dose with originally the widest peri-ablational Hsp70 rim), tumour coagulation was increased compared to when Mic-Qu was added to the RFA/Lipo-Dox 70 °C × 5 min arm that had a narrower baseline Hsp70 rim thickness (15.3 ± 1.0 mm versus 10.5 ± 0.5 mm coagulation diameter, respectively, p < 0.001, ). Additionally, complete ablation was observed in 2/5 of the tumours (16 mm diameter) treated with RFA/Lipo-Dox/Mic-Qu at 90 °C × 2 min, with no viable margins to evaluate Hsp70 rim expression (compared to no complete ablation observed for any of the other groups in phase III). Furthermore, a significantly narrower peri-ablational Hsp70 rim was observed for the remaining three RFA/Lipo-Dox/Mic-Qu tumours treated at 90 °C × 2 min (672.3 ± 61.9 μm) as compared to the five RFA/Lipo-Dox at 90 °C × 2 min (1030.1 ± 192.0 μm, p < 0.04), corresponding to the increased tumour coagulation.
Figure 6. Comparison of RFA coagulation diameter and peri-ablational Hsp70 expression for varied RF applications combined with liposomal doxorubicin and/or micellar quercetin. Significantly greater coagulation gains are achieved in 90 °C × 2 min treatment group, 15.3 ± 1.0 mm, when combined with doxorubicin and micellar quercetin as compared to 70 °C × 5 min, 10.5 ± 0.5 mm, when combined with doxorubicin and micellar quercetin. RF + D, radio-frequency ablation plus liposomal doxorubicin; DMicQ, liposomal doxorubicin plus micellar quercetin.
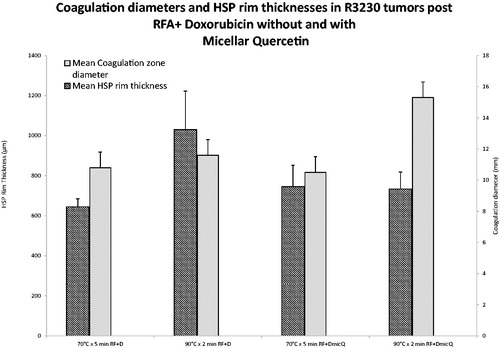
Discussion
Traditionally, efforts at improving high-temperature thermal ablation have focused on creating an optimal zone of cytotoxic tissue injury that encompasses the target tumour and an additional 5–10 mm of normal tissue as an ablative margin. For pathology, tissue changes from high-temperature ablation have been grossly divided into a central ‘white’ zone of immediately coagulated tissue and a peri-ablational ‘red zone’ of reactive inflammation [Citation28]. Currently, all ablation application algorithms have largely prioritised obtaining the most completely uniform ‘white zone’ in a clinically efficient manner (i.e. ablating the desired target volume in the shortest amount of time) or achieving a specific ablation geometry (e.g. spherical ablation volume) [Citation29–31]. However, there is now greater appreciation of the various secondary reactions that occur in the peri-ablational rim exposed to non-lethal hyperthermic injury, with increases in protective heat shock protein expression, microvascular permeability, growth factor production, and reactive oxygen species reported [Citation32]. Whereas several studies have reported that combination therapies can be effective in increasing focal ablation coagulation by targeting many of these factors, investigation of the potential role for modifying actual ablation parameters to improve the efficacy of combined ablation–drug therapies by tailoring thermal dose to the targeting of specific tissue responses in the peri-ablational rim has thus far been limited.
In this study we demonstrate that for various equivalent ablation zone sizes, the thickness of the peri-ablational inflammation in normal liver (represented by Hsp70 expression) varies based upon ablation parameters, and is greater for lower-temperature longer duration ablations compared to higher temperature shorter duration ablations. Interestingly, we further note that increasing the thickness of the peri-ablational rim correlated more closely with longer ablation times rather than higher tip temperatures. Regardless, our findings clearly suggest that ablation algorithms can be potentially designed and tailored in a manner to either maximise or minimise the extent of peri-ablational inflammation beyond the central coagulation zone. This is likely to have substantial clinical relevance, particularly with the increasing recognition that secondary peri-ablational tissue changes may be synergistically modulated with drugs to augment the pure ‘thermal toxicity’ of high temperature ablation. For example, when increased peri-ablational nanodrug delivery is desired, longer heating times produce greater inflammation and microvascular change for a given amount of tumour coagulation which may enable optimisation of combination ablation/drug strategies that take advantage of a larger peri-ablational rim to maximise drug delivery [Citation33]. This notion is supported from the findings observed in a recently reported multi-centre clinical trial combining hepatic RFA with adjuvant intravenous thermosensitive Lipo-Dox in the treatment of hepatocellular carcinoma [Citation6]. While no initial treatment benefit was observed for combined ablation/Lipo-Dox was observed, a sub-group analysis suggested that longer heating times led to improved treatment response [Citation6]. Thus, in some instances tailoring the ablation algorithm to increase the volume of tissue exposed to hyperthermia may be required to maximise the efficacy of adjuvant drug therapies (similar to sensitisation strategies reported for combined radiation and chemotherapy protocols [Citation34–36]), particularly when the adjuvant drugs are targeting tissues that are partly injured from non-lethal hyperthermia. By contrast, several recent studies have demonstrated that peri-ablational inflammation after RFA of normal liver can incite unwanted stimulatory effects on separate tumour tissue within the liver or in distant sites [Citation37,Citation38], suggesting that in some instances, use of ablation protocols that minimise peri-ablational reactions for the same-sized coagulation zone will be advantageous.
The results of our study are concordant with multiple prior studies reporting that adjuvant single-dose long-circulating Lipo-Dox can increase local RFA-induced tumour coagulation. Beyond this, our study demonstrates that adjuvant Lipo-Dox also increases peri-ablational processes including Hsp70 expression at all thermal doses compared to RFA alone. We further observed that despite often resulting in similarly-sized ablation zones, ablation parameters of different thermal dose combined with Lipo-Dox resulted in variable peri-ablational heat shock protein expression. This demonstrates that although a wide range of adjuvant Lipo-Dox increases local tumour destruction over RFA alone, some combinations can simultaneously stimulate relatively higher levels of specific reactions such as Hsp70 in tissues beyond the expanded zone of white coagulation. This forms the basis for developing a multi-mechanism approach, where even larger ablation zones can be achieved with multi-drug regimens, by first stimulating specific reactions with one drug and then secondarily targeting them with a second agent. Such tandem drug approaches targeting multiple mechanisms are commonly used in systemic chemotherapy paradigms, but not yet commonly developed in interventional oncology practice. In our study, two different ablation settings with the same Lipo-Dox dose induced the same coagulation, but with variable Hsp70 expression. Here, this simulated that both of those ablation regimens would have achieved the same clinical ablation end point after ablation plus one adjuvant drug, but for which a second drug can be beneficial. Adjuvant Lipo-Dox likely induces both increased local cytotoxicity in cells partially injured in the peri-ablational rim, and increased protective Hsp70 expression in surviving cells, which is then blocked by Mic-Qu, resulting in even greater overall tumour destruction for ablation arms with the greatest Hsp70. Thus, even for combination therapy paradigms, multiple factors such as ablation parameters, adjuvant drugs, and additional peri-ablational reactions, all need to be carefully balanced to achieve the most efficacious ablation treatment and corresponding clinical outcomes.
We also observed tissue-specific differences between normal liver and subcutaneous tumour in both coagulation as well as peri-ablational rim thickness for specified thermal doses with RFA alone. Differences in tissue sensitivity to conventional hyperthermia have been well-described [Citation39], along with differences in tissue-specific heating during ablation due to variations in electrical conductivity, tissue perfusion, and heat transmission [Citation40]. Additionally, variable and potentially greater tumour sensitivity to hyperthermic injury compared to normal tissues has also been reported [Citation41], and heightened sensitivity to hyperthermia was observed in the tumour model we used, as evidenced by greater peri-ablational HSP expression for similar RFA parameters. Furthermore, as any successful clinical tumour ablation is dependent on ablating an additional rim of normal tissue, tailoring the ablation protocol to maximise the peri-ablational secondary response in normal liver rather than tumour may be even more important to achieving peak drug delivery in the peri-ablational rim [Citation42].
Finally, we further demonstrate that while CEM43 was a good predictor of ablation size in normal liver and R3230 tumour models, it did not confer better predictability of peri-ablational hyperthermia responses in either normal or tumour tissue. Thus, HSP expression likely follows other non-exponential kinetics, and suggests that classic thermal dose equations alone may have limited predictive value for clinical use in developing optimised ablation protocols for adjuvant targeted drug therapies. Given the differences in tissue heat transmission, tissue heterogeneity with regard to biophysical limitations to heating (such as tissue perfusion), and variability in tissue-specific susceptibility to heating, it remains a challenge to apply commonly used metrics of thermal dose from conventional hyperthermia studies to point-source high-temperature ablation systems. Indeed, it must be noted that our use of CEM43 for focal ablative heating scenarios is one that most likely corresponds to a CEM43 T10 value (representing the thermal dose in the warmest 10% of the volume), whereas conventional hyperthermia strategies have largely been predicated upon achieving the target temperatures in 50% or 90% of the heated volume (i.e. T50 and T90 values which are thought to have better correlation to tumour control when using hyperthermia alone [Citation43–44]. Regardless, for the very specific and very local temperature profile in the case of RFA, CEM43T10 is very likely to correlate very strongly with either CEM43T50 or CEM43T90.
There are several limitations with the current study. Only a single tumour model was used, and though this model has been used in multiple studies combining RFA and adjuvant liposomal chemotherapy, careful extrapolation of findings in this model to other models and human tumours is warranted. Additionally, here the relationship between thermal dose and early ablation outcome measures (i.e. coagulation and Hsp70 expression) were studied for all therapies. Correlation to longer-term outcomes (such as tumour growth and end-point survival) will also be ultimately required. Furthermore, in our study, thermal dose calculated using CEM43 °C was for a focal thermal point source. Unlike complete tumour thermal dose classically reported in the literature when using this model, here thermal dose represents the energy dispensed at the centre of the tumour. Given the nature of our in vivo studies and the size limitations of our animal model, acquiring such complete tumour thermal dose would be technically unfeasible and of little value to address the questions furnished in this study. Finally, although we demonstrate reasonable correlation between administered thermal dose and tissue damage, our current data might be part of a complex phenomenon with multiple peaks. Greater elaboration and study are due, lest we fail to recognise the overall phenomena.
In summary, combined drug/ablation strategies to improve ablation efficacy by targeting tissue responses in the peri-ablational rim require a more careful study of how thermal ablation dose can be manipulated to simultaneously optimise the size of the central coagulation and peripheral peri-ablational rim. For RF thermal ablation, longer heating, lower temperature protocols may permit greater zones of peri-ablational inflammation compared to higher temperature, faster heating protocols. Such approaches may be tailored to maximise the peri-ablational effects of adjuvant nanodrugs, including developing multi-drug regimens that target more than one mechanism within the peri-ablational rim that result in even larger ablation zones.
Funding information
This study was supported by grants from the National Cancer Institute, Bethesda, MD (CCNE1U54CA151881-01), the Harvard Medical Faculty Physicians Radiology Foundation, and the Israel Research Foundation/Israel Ministry of Health.
Disclosure statement
The authors report no other conflicts of interest. The authors alone are responsible for the content and writing of the paper.
References
- Kim YS, Lim HK, Rhim H, Lee MW. Ablation of hepatocellular carcinoma. Best Pract Res Clin Gastroenterol 2014;28:897–908.
- Fornage BD, Hwang RF. Current status of imaging-guided percutaneous ablation of breast cancer. Am J Roentgenol 2014;203:442–8.
- De Filippo M, Bozzetti F, Martora R, Zagaria R, Ferretti S, Macarini L, et al. Radiofrequency thermal ablation of renal tumors. Radiol Med 2014;119:499–511.
- Salapura V, Jeromel M. Minimally invasive (percutaneous) treatment of metastatic spinal and extraspinal disease – a review. Acta Clin Croat 2014;53:44–54.
- Mirza AN, Fornage BD, Sneige N, Kuerer HM, Newman LA, Ames FC, et al. Radiofrequency ablation of solid tumors. Cancer J 2001;7:95–102.
- Lin SM, Lin CJ, Lin CC, Hsu CW, Chen YC. Radiofrequency ablation improves prognosis compared with ethanol injection for hepatocellular carcinoma < or =4 cm. Gastroenterology. 2004;127(6):1714–23.
- Stang A, Fischbach R, Teichmann W, Bokemeyer C, Braumann D. A systematic review on the clinical benefit and role of radiofrequency ablation as treatment of colorectal liver metastases. Eur J Cancer 2009;45:1748–56.
- Alexander ES, Machan JT, Ng T, Breen LD, DiPetrillo TA, Dupuy DE. Cost and effectiveness of radiofrequency ablation versus limited surgical resection for stage I non-small-cell lung cancer in elderly patients: is less more? J Vasc Interv Radiol 2013;24:476–82.
- Solbiati L, Ahmed M, Cova L, Ierace T, Brioschi M, Goldberg SN. Small liver colorectal metastases treated with percutaneous radiofrequency ablation: local response rate and long-term survival with up to 10-year follow-up. Radiology 2012;265:958–68.
- Lai EC, Tang CN. Radiofrequency ablation versus hepatic resection for hepatocellular carcinoma within the Milan criteria – a comparative study. Int J Surg 2013;11:77–80.
- Kim YS, Lim HK, Rhim H, Lee MW, Choi D, Lee WJ, et al. Ten-year outcomes of percutaneous radiofrequency ablation as first-line therapy of early hepatocellular carcinoma: analysis of prognostic factors. J Hepatol 2013;58:89–97.
- Mulier S, Ni Y, Jamart J, Ruers T, Marchal G, Michel L. Local recurrence after hepatic radiofrequency coagulation: multivariate meta-analysis and review of contributing factors. Ann Surg 2005;242:158–71.
- Kruskal JB, Oliver B, Huertas JC, Goldberg SN. Dynamic intrahepatic flow and cellular alterations during radiofrequency ablation of liver tissue in mice. J Vasc Interv Radiol 2001;12:1193–201.
- Goldberg SN, Kamel IR, Kruskal JB, Reynolds K, Monsky WL, Stuart KE, et al. Radiofrequency ablation of hepatic tumors: increased tumor destruction with adjuvant liposomal doxorubicin therapy. Am J Roentgenol 2002;179:93–101.
- Solazzo SA, Ahmed M, Schor-Bardach R, Yang W, Girnun GD, Rahmanuddin S, et al. Liposomal doxorubicin increases radiofrequency ablation-induced tumor destruction by increasing cellular oxidative and nitrative stress and accelerating apoptotic pathways. Radiology 2010;255:62–74.
- Ahmed M, Liu Z, Lukyanov AN, Signoretti S, Horkan C, Monsky WL, et al. Combination radiofrequency ablation with intratumoral liposomal doxorubicin: effect on drug accumulation and coagulation in multiple tissues and tumor types in animals. Radiology 2005;235:469–77.
- Soundararajan A, Dodd GD III, Bao A, Phillips WT, McManus LM, Prihoda TJ, et al. Chemoradionuclide therapy with 186Re-labeled liposomal doxorubicin in combination with radiofrequency ablation for effective treatment of head and neck cancer in a nude rat tumor xenograft model. Radiology 2011;261:813–23.
- Yang W, Ahmed M, Tasawwar B, Levchenko T, Sawant RR, Collins M, et al. Radiofrequency ablation combined with liposomal quercetin to increase tumour destruction by modulation of heat shock protein production in a small animal model. Int J Hyperthermia 2011;27:527–38.
- Yang W, Ahmed M, Tasawwar B, Levchenko T, Sawant RR, Torchilin V, et al. Combination radiofrequency (RF) ablation and intravenous liposomal heat shock protein suppression: reduced tumor growth and increased animal endpoint survival in a small animal tumor model. J Control Release 2012;160:239–44.
- Mertyna P, Dewhirst MW, Halpern E, Goldberg W, Goldberg SN. Radiofrequency ablation: the effect of distance and baseline temperature on thermal dose required for coagulation. Int J Hyperthermia 2008;24:550–9.
- Goldberg SN, Gazelle GS, Dawson SL, Rittman WJ, Mueller PR, Rosenthal DI. Tissue ablation with radiofrequency: effect of probe size, gauge, duration, and temperature on lesion volume. Acad Radiol 1995;2:399–404.
- Poon, RTP. Phase 3, randomized, double-blind, dummy-controlled, trial of radiofrequency ablation (RFA) + lyso-thermosensitive liposomal doxorubicin (LTLD, Thermodox®) for hepatocellular carcinoma (HCC) lesions 3-7Cm. Abstract presented at the International Liver Cancer Association Seventh Annual Conference, September 2013.
- Sapareto SA, Dewey WC. Thermal dose determination in cancer therapy. Int J Radiat Oncol Biol Phys 1984;10:787–800.
- Chen T, Guo J, Han C, Yang M, Cao X. Heat shock protein 70, released from heat-stressed tumor cells, initiates antitumor immunity by inducing tumor cell chemokine production and activating dendritic cells via TLR4 pathway. J Immunol 2009;182:1449–59.
- Yuan H, Schroeder T, Bowsher JE, Hedlund LW, Wong T, Dewhirst MW. Intertumoral differences in hypoxia selectivity of the PET imaging agent 64Cu(II)-diacetyl-bis(N4-methylthiosemicarbazone). J Nucl Med 2006;47:989–98.
- van Rhoon GC, Samaras T, Yarmolenko PS, Dewhirst MW, Neufeld E, Kuster N. CEM43 degrees C thermal dose thresholds: a potential guide for magnetic resonance radiofrequency exposure levels? Eur Radiol 2013;23:2215–27.
- Chang IA. Considerations for thermal injury analysis for RF ablation devices. Open Biomed Eng J 2010;4:3–12.
- Scudamore CH, Lee SI, Patterson EJ, Buczkowski AK, July LV, Chung SW, et al. Radiofrequency ablation followed by resection of malignant liver tumors. Am J Surg 1999;177:411–17.
- Baust JG, Gage AA, Klossner D, Clarke D, Miller R, Cohen J, et al. Issues critical to the successful application of cryosurgical ablation of the prostate. Technol Cancer Res Treat 2007;6:97–109.
- Hernandez JI, Cepeda MF, Valdes F, Guerrero GD. Microwave ablation: state-of-the-art review. Onco Targets Ther 2015;8:1627–32.
- Quaranta V, Manenti G, Bolacchi F, Cossu E, Pistolese CA, Buonomo OC, et al. FEM analysis of RF breast ablation: multiprobe versus cool-tip electrode. Anticancer Res 2007;27:775–84.
- Solazzo SA, Ahmed M, Schor-Bardach R, Yang W, Girnun GD, Rahmanuddin S, et al. Liposomal doxorubicin increases radiofrequency ablation-induced tumor destruction by increasing cellular oxidative and nitrative stress and accelerating apoptotic pathways. Radiology 2010;255:62–74.
- Kruskal JB, Oliver B, Huertas JC, Goldberg SN. Dynamic intrahepatic flow and cellular alterations during radiofrequency ablation of liver tissue in mice. J Vasc Intervent Radiol 2001;12:1193–201.
- Cabibbo G, Latteri F, Antonucci M, Craxi A. Multimodal approaches to the treatment of hepatocellular carcinoma. Nature Clin Pract Gastroenterol Hepatol 2009;6:159–69.
- Hoffmann RT, Jakobs TF, Kubisch CH, Stemmler HJ, Trumm C, Tatsch K, et al. Radiofrequency ablation after selective internal radiation therapy with yttrium90 microspheres in metastatic liver disease – is it feasible? Eur J Radiol 2010;74:199–205.
- Horkan C, Dalal K, Coderre JA, Kiger JL, Dupuy DE, Signoretti S, et al. Reduced tumor growth with combined radiofrequency ablation and radiation therapy in a rat breast tumor model. Radiology 2005;235:81–8.
- Shiozawa K, Watanabe M, Takahashi M, Wakui N, Iida K, Sumino Y. Analysis of patients with rapid aggressive tumor progression of hepatocellular carcinoma after percutaneous radiofrequency ablation. Hepatogastroenterology 2009;56:1689–5.
- Nijkamp MW, Borren A, Govaert KM, Hoogwater FJ, Molenaar IQ, van Diest PJ, et al. Radiofrequency ablation of colorectal liver metastases induces an inflammatory response in distant hepatic metastases but not in local accelerated outgrowth. J Surgl Oncol 2010;101:551–6.
- Yarmolenko PS, Moon EJ, Landon C, Manzoor A, Hochman DW, Viglianti BL, et al. Thresholds for thermal damage to normal tissues: An update. Int J Hyperthermia 2011;27:320–43.
- Ahmed M, Liu Z, Afzal KS, Weeks D, Lobo SM, Kruskal JB, et al. Radiofrequency ablation: effect of surrounding tissue composition on coagulation necrosis in a canine tumor model. Radiology 2004;230:761–7.
- Brown SL, Hunt JW, Hill RP. Differential thermal sensitivity of tumour and normal tissue microvascular response during hyperthermia. Int J Hyperthermia 1992;8:501–14.
- Mulier S, Ni Y, Jamart J, Ruers T, Marchal G, Michel L. Local recurrence after hepatic radiofrequency coagulation: multivariate meta-analysis and review of contributing factors. Ann Surg 2005;242:158–71.
- Thrall DE, Rosner GL, Azuma C, Larue SM, Case BC, Samulski T, et al. Using units of CEM43 °C T90, local hyperthermia thermal dose can be delivered as prescribed. Int J Hyperthermia 2000;16:415–28.
- Yarmolenko PS, Moon EJ, Landon C, Manzoor A, Hochman DW, Viglianti BL, et al. Thresholds for thermal damage to normal tissues: an update. Int J Hyperthermia 2011;27:320–43.