Abstract
Objective. The advantages of highly localised, conformal treatments achievable with stereotactic radiotherapy (SRT) are increasingly being extended to extracranial sites as stereotactic body radiotherapy with advancements in imaging and beam collimation. One of the challenges in stereotactic treatment lies in the significant complexities associated with small field dosimetry and dose calculation. This review provides a comprehensive overview of the complexities associated with stereotactic radiotherapy and the potential for detriment. Methods. This study is based on a comprehensive review of literature accessible via PubMed and other sources, covering stereotactic radiotherapy, small-field dosimetry and dose calculation. Findings. Several key issues were identified in the literature. They pertain to dose prescription, dose measurement and dose calculation within and beyond the treatment field. Field-edge regions and penumbrae occupy a significant portion of the total field size. Spectral and dosimetric characteristics are difficult to determine and are compounded by effects of tissue inhomogeneity. Measurement of small-fields is made difficult by detector volume averaging and energy response. Available dosimeters are compared, and emphasis is given to gel dosimetry which offers the greatest potential for three-dimensional small-field dosimetry. The limitations of treatment planning system algorithms as applied to small-fields (particularly in the presence of heterogeneities) is explained, and a review of Monte Carlo dose calculation is provided, including simplified treatment planning implementations. Not incorporated into treatment planning, there is evidence that far from the primary field, doses to patients (and corresponding risks of radiocarcinogenesis) from leakage/scatter in SRT are similar to large fields. Conclusions. Improved knowledge of dosimetric issues is essential to the accurate measurement and calculation of dose as well as the interpretation and assessment of planned and delivered treatments. This review highlights such issues and the potential benefit that may be gained from Monte Carlo dose calculation and verification via three-dimensional dosimetric methods (such as gel dosimetry) being introduced into routine clinical practice.
The objective of the present work is to give an overview of stereotactic radiotherapy and radiosurgery and to describe, in detail, the difficulties associated with the small-fields employed in stereotactic techniques in terms of dose measurement and calculation. Stereotactic radiotherapy and radiosurgery are increasingly common treatment modalities. highlights this trend – with one paper relating to stereotactic radiotherapy published in the early sixties compared to over 1700 papers published in the last four years. The emphasis of this paper is to meet the objective of giving the reader an understanding of the complexities associated with the use of small fields in terms of dose measurement and calculation. Uncertainties in small-field measurements and calculations can lead to incorrect prediction of patient doses, which may lead to complications. The need for accurate radiation delivery and confident knowledge of the characteristics of the dose distributions resulting from such small fields is highlighted. A notoriously tricky endeavour, there are a number of means for calculating and measuring the latter, and these are discussed with a particular emphasis on the use of the most promising technologies: three-dimensional integrating gel dosimetry and Monte Carlo radiation transport modelling. Whilst the focus is issues related to dosimetry (measurement and calculation), difficulties related to positioning and immobilisation are also discussed.
Figure 1. An indication (based on a PubMed search of published literature) of the increasing implementation of both intra- and extra-cranial stereotactic radiotherapy. Note the logarithmic scale. ‘Stereotactic’ includes both intra- and extra-cranial.
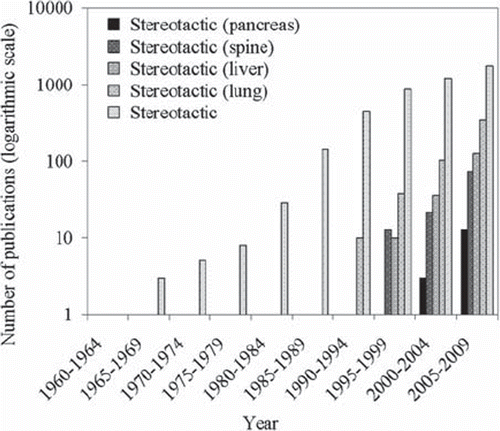
A contemporary definition of stereotactic radiotherapy is defined here as ‘the use of beams of ionising radiation from multiple directions intersecting at a target (usually intracranial) spatially defined using a fixed three-dimensional coordinate system’. Stereotactic radiosurgery may be defined similarly, with the difference that it implies a single fraction treatment. The term radio-surgery is something of a misnomer, since it is a non-invasive operation. Stereotactic radiotherapy/radiosurgery is often considered, by default, to refer to intracranial treatments due to widespread usage following the early adoption of such treatments and the amenability of the cranium to frame fixation and target localisation. This has led to the frequent use of the term ‘extracranial stereotactic radiotherapy’ for stereotactic body radiotherapy.
Stereotactic radiotherapy (SRT) and radiosurgery (SRS)
Stereotactic radiotherapy and radiosurgery for treatment of intracranial lesions
Intracranial SRT and SRS. The origins of stereotactic intracranial surgery may be traced back to work first presented at the 1906 meeting of the British Medical Association in Toronto. In 1908, after several years of use in studies of the structure and functions of the cerebellum in various animals, Horsley and Clarke published details of a stereotactic instrument that incorporated a frame attached to the skull and a three dimensional coordinate system that facilitated the precise application of an insulated needle for excitation or electrolysis [Citation1]. Based on the same fundamental principles, stereotactic radiosurgery (SRS) is a single fraction radiotherapy modality for the treatment of intracranial lesions, employing stereotactic apparatus and multiple small beams delivered through non-coplanar isocentric arcs. The word ‘radiosurgery’ is attributed to Leksell, a neurosurgeon [Citation2]. Initially, SRS was used primarily for treatment of arteriovenous malformations (AVM) [Citation3,Citation4] and other benign brain lesions [Citation5]. More recently, SRS has become increasingly applied for the treatment of malignant brain lesions, including primary tumours and metastases. Stereotactic radiotherapy (SRT) refers to the same procedure as SRS, but involves multiple dose fractions. SRS and SRT rely on 3D localisation of the lesion, utilising immobilisation devices. The stereotactic apparatus is employed during imaging and treatment for target localisation and head immobilisation. This must be done with a high degree of accuracy, necessitating meticulous quality assurance (QA) processes. Conformity in dose is sought with use of collimated beams, optimised arc angles and multiple isocentres. Alternatively, it is possible to dynamically shape the field during rotation with use of mini- / micro- multileaf collimators.
Stereotactic radiosurgery is typically delivered via either the Leksell Gamma-Knife system (LGK; Elekta) or with a conventional megavoltage (MV) linac. The former is a commercialised piece of equipment incorporating a modified 60Co unit with 201 convergent beams directed through variable circular collimators [Citation6]. SRS with a linac is sometimes referred to as ‘x-ray knife’ treatment. This involves the use of multiple non-coplanar arcs of circularly or dynamically shaped fields that converge on the isocentre. A stereotactic frame is fixed to the patient's head and locked onto the treatment couch. The target coordinates are matched to the machine isocentre with a high degree of precision.
In summary, there are several key points that may be made regarding the efficacy of stereotactic approaches:
SRT results in comparable survival rates and better normal tissue sparing than conformal radiotherapy [Citation7–9].
The use of linac-based stereotactic techniques (with a multileaf collimator) can be advantageous, in terms of dose homogeneity and normal tissue sparing [Citation10–14].
The addition of intensity modulation to stereotactic treatments results in improved dose conformality and improved organ-at-risk sparing [Citation15–19].
The brain is known to exhibit sensitivity for acute and delayed radiation damage. Review of published clinical data reveals a number of key trends regarding complications following SRT and SRS:
There are a range of immediate SRT/SRS potential side-effects, affecting about one third of patients, though these are typically moderate and short term [Citation20].
Although accurate localisation reduces the likelihood, there is potential for severe late effects ranging from neurological impairment to death [Citation5,Citation21–25].
Little is known about long-term neuropsychological effects. Clinical findings show children exhibit cognitive decline subsequent to fractionated radiotherapy of brain tumours; data for adults is comparatively scarce, with preliminary finding suggesting some cognitive function, such as memory, may be particularly vulnerable [Citation26].
There is a risk of radiation-induced cancer (particularly meningiomas [Citation27]) resulting from intracranial treatments in general [Citation28–31]; however, the likelihood of radiation-induced tumours becoming manifest following stereotactic techniques is relatively low [Citation32].
There are reported cases of radiation induced-induced tumours from stereotactic radiosurgery, the majority of which are glioblastomas [Citation33–38].
Stereotactic radiotherapy and radiosurgery may also be employed to extracranial regions. Generally referred to as stereotactic body radiotherapy, though the term extracranial stereotactic radiotherapy is often still applied, is discussed in the following section.
Stereotactic body radiotherapy (SBRT) and the need for accurate dose delivery: The potential for radiation toxicity
Overview. As mentioned earlier, stereotactic radiotherapy generally implies treatment of intracranial lesions. An obvious extension is to apply similar treatment techniques to small tumours at other anatomic locations; such treatments are often referred to as ‘extracranial stereotactic radiotherapy’ or ‘stereotactic body radiotherapy’ (SBRT). A review of the relevant scientific literature indicates that extracranial treatments are of most interest in relation to the lung, liver and the spine.
There are several key differences between conformal or intensity-modulated radiotherapy and SBRT. In the former case, doses of up to 3 Gy/fraction are typically given over 10–30 fractions, whilst for SBRT up to 45 Gy/fraction may be given in just a few (<5) fractions. Margins are also of the order of millimetres for SBRT, whereas intensity modulated radiotherapy (IMRT) margins are typically of the order of centimetres. It is important to note this latter point, since often treatment planning systems have a dose grid resolution of the same order of magnitude as the margins in the case of SBRT (e.g. 1–2 mm grid size for AAA/PBC).
One critical element of SBRT is the use of a suitable immobilisation methodology. The earlier forays into SBRT were facilitated by apparatus such as an extracranial stereotactic frame proposed by Hamilton et al. for treatment of spinal lesions [Citation39] and a body frame with vacuum bag implemented by Lax et al. for treatment of lesions of the liver and lung [Citation40]. Ultimately, image-guidance may be considered the ideal approach, with applications in not only initial patient setup but also real-time monitoring of the target (or surrogate marker) during treatment.
For lung treatments, a range of immobilisation and positioning devices have been employed: the Elekta Body Frame (Elekta Oncology, Stockholm) with accuracies ranging from 1.8 to 5 mm [Citation41–44], the MI BodyFIX (Medical Intelligence, Schwabmuenchen) with accuracies ranging from 2.5 to 3 mm [Citation45] and the Leibinger Body Frame (Leibinger, Freiburg) with accuracies of 2 to 4.4 mm [Citation46]. For liver treatments, the Elekta Body Frame has been used with an accuracy of ≤ 4.4 mm [Citation41], the MI BodyFIX has been employed with a reported accuracy of ≤ 3.2 mm [Citation45] and the Leibinger Body Frame has been used for liver treatments with a reported accuracy of 1.8–4.4 mm [Citation47]. Tokuuye et al. found motion was significantly reduced just by making the patient lie ventrally on the couch and strapping the jaw and arms down (achieving an accuracy of approximately 5 mm) [Citation48]. For spinal treatments a range of approaches have been taken. These include use of the MI BodyFIX with approximately millimetre accuracy [Citation49], use of a body cast with ∼3 mm accuracy [Citation50] and approaches using frameless real-time tracking of fiducial markers achieving accuracies of approximately 2 mm [Citation51].
Stereotactic body radiotherapy is facilitated by such approaches to immobilisation, with further distinctions from other techniques in methods and importance of dose prescription/verification and hypofractionation. The ultimate objectives remain the same: the dose to the tumour volume must be maximised, whilst minimising doses to normal tissues; the following discussion concentrates on the clinically reported acute and delayed side-effects of SBRT.
SBRT of the lung. Complicated by the added issue of tissue heterogeneities (see the following section on charged particle equilibrium), small (≤4 or 5 cm) lung tumours may be treated with SBRT. Hiraoka et al. [Citation52] identify several further complications, namely: accurate body fixation; tumour motion due to respiration; optimal treatment regimens; the limited data for normal tissue toxicity resulting from large-fraction irradiation; and optimal fractionation schemes. gives an overview of some studies employing SBRT for the treatment of primary lung cancers, with the local control specified (for median follow-up varying between 11 and 43 months).
Figure 2. An overview of some studies on SBRT for the treatment of primary lung cancer, showing the number of patients, N, dose, number of fractions and local control rate. Median follow-up varied between 11 and 43 months [Citation42,Citation44,Citation56–64].
![Figure 2. An overview of some studies on SBRT for the treatment of primary lung cancer, showing the number of patients, N, dose, number of fractions and local control rate. Median follow-up varied between 11 and 43 months [Citation42,Citation44,Citation56–64].](/cms/asset/292be26f-3ff6-479c-85a3-ae0bedf9c02b/ionc_a_551665_f0002_b.gif)
One key difficulty with treatment of lung tumours is immobilisation in the presence of tumours moving with patient respiration.
The first clinical stereotactic body frame involved fixating patients using a vacuum pillow, such that cranial SRT coordinates could be employed for extracranial SBRT [Citation53,Citation54].
Work on SBRT for lung tumours started in earnest in the 1990s. Clinical outcomes for SBRT were first published in Acta Oncologica [Citation55].
With regard to the issue of respiratory motion, three methods are typically employed to minimise its impact: respiratory holding, regulation and gating. Respiratory holding involves the patient holding their breath for a period of beam-on time. Respiratory regulation involves externally applied pressure to control the diaphragm. Respiratory gating involves alternating beam-on/off time according to patient motion, where the latter is reflected by the movement of external or implanted fiducial markers.
What is considered as an ‘optimum’ treatment plan differs between countries and undoubtedly between individual centres.
The Japanese approach stresses dose homogeneity, with prescription specified at the isocentre; whereas the United States approach involves dose prescription at the PTV margin, with less concern for homogeneity.
The toxicity concerns relate mainly to pulmonary complications, radiation pneumonitis, haemoptysis and radiation esophagitis [Citation65]. Other concerns include the effect on the central bronchus, pulmonary artery, oesophagus, heart and spinal cord, for which there has not been significant follow-up [Citation66]. Toxicity falls into various grades defined by the Radiation Therapy Oncology Group (RTOG, United States) as outlined in .
The ROSEL trial compared surgery with radiosurgery for lung patients and suggested guidelines for stereotactic body radiotherapy incorporating, in particular, tighter normal tissue dose constraints for complication minimisation [Citation67]. The study also discusses the caution that must be taken when calculating dose distributions (and retrospectively interpreting clinical data) with simplistic algorithms, as discussed later in the present paper.
There is strong contemporary interest in radiobiological modelling as a means of determining preferential dose distributions and schedules for the minimisation of toxicity and overall improved efficacy; Chi et al. presented an interesting paper recently linking radiobiological modelling with patient outcomes [Citation68].
Table I. The Radiation Therapy Oncology Group (RTOG, United States) definitions of the various grades of pulmonary toxicity.
Milano et al. [Citation69] provide a good review of normal tissue toxicity following SBRT. Acute fatigue, coughing and dermatitis are commonly reported. Acute pneumonitis is also reported, though typically not beyond grade 3. gives an overview of the toxicity observed in some recent studies of SBRT as applied to the lung.
Figure 3. Various recent studies reporting observations of toxicity at or above Grade 3 (refer to for definition) post-SBRT. The dose in Gy is given, along with the number of fractions. N refers to the total patient population, and n refers to the number of patients exhibiting toxicity (these numbers are given explicitly in the figure) [Citation57,Citation70–75].
![Figure 3. Various recent studies reporting observations of toxicity at or above Grade 3 (refer to Table I for definition) post-SBRT. The dose in Gy is given, along with the number of fractions. N refers to the total patient population, and n refers to the number of patients exhibiting toxicity (these numbers are given explicitly in the figure) [Citation57,Citation70–75].](/cms/asset/173fae6a-9c62-4aa0-a9c1-622e76382c17/ionc_a_551665_f0003_b.gif)
SBRT of the liver. The liver is also amenable to therapy with SBRT. The desired advantage of SBRT is that better normal tissue sparing should be achievable than conventional or conformal radiation delivery approaches.
Radiation-induced liver damage (RILD) is dependent on dose and the irradiated volume, and a good review of reported RILD data may be found in a book chapter by Herfarth and Münter [Citation76].
Lax et al. [Citation40] presented the first implementation of SBRT for liver malignancies.
Single fraction radiosurgery has been shown to have detrimental consequences; Blomgren et al. [Citation55] treated five patients, one of whom died two days after treatment, the other four experiencing marginal recurrence.
More positive results were achieved using hypofractionation; two patients died from RILD (liver cirrhosis), but other patients suffered at worst nausea, fever or chills post-radiosurgery (N = 24) [Citation53].
Wulf et al. [Citation46] treated patients (N = 24) with liver tumours using 3 × 10 Gy, resulting in 76% local control (with 12 month follow-up). Wulf et al. [Citation77] then published a later study with better statistics (N = 39), treating with a high dose (3 × 12.5 Gy, or 1 × 20 Gy) and low dose (3 × 10 Gy) regime, yielding local control of 92% (with 12 month follow-up). The high dose group exhibited better local control (100% and 82% at 1 and 2 years respectively) than the low dose group (86% and 56% at 1 and 2 years respectively).
Data from a multi-centre United States trial [Citation78] showed local control (for N = 21) of 93% at 18 months.
Acute fatigue, nausea, diarrhoea and dermatitis are commonly reported adverse reactions to SBRT of the liver. However, grade ≥3 toxicity (such as hepatic failure, bowel perforation/obstruction and gastrointestinal bleeding) are rare; provides an overview of reported toxicity at grades 3 or higher. The most striking data in is that of the study by Hoyer et al. [Citation79]. The latter study relates to colorectal carcinoma liver metastases. Overall survival was 67% and 13% after one and five years respectively. Of the patients exhibiting toxicity grades of 3, three patients had intestinal toxicity, two had nausea, two had diarrhoea, ten had pain, and two had skin reactions. Of the patients exhibiting grade 4 toxicity, one had hepatic failure and one had pain. For the patient who had fatal hepatic failure, 60% of the liver received a dose >10 Gy and the patient received a median liver dose of 14.4 Gy. Patients were given three fractions over a period of 5–8 days; the other studies in gave three fractions in 3–14 days or six fractions in 14 days. Overall survival in the Hoyer et al. study was 67% over one year to 13% over five years, with local control of 86% over two years.
Figure 4. Various recent studies reporting observations of toxicity at or above Grade 3 post-SBRT for liver lesions. The dose in Gy is given, along with the number of fractions. N refers to the total patient population, and n refers to the number of patients exhibiting toxicity (the latter is shown explicitly in the figure) [Citation78–81].
![Figure 4. Various recent studies reporting observations of toxicity at or above Grade 3 post-SBRT for liver lesions. The dose in Gy is given, along with the number of fractions. N refers to the total patient population, and n refers to the number of patients exhibiting toxicity (the latter is shown explicitly in the figure) [Citation78–81].](/cms/asset/408b77b6-9d9d-4e7c-84cd-d8e4c02e94c5/ionc_a_551665_f0004_b.gif)
SBRT of the pancreas. Radiosurgery for tumours of the pancreas is an emergent mode of therapy. Recent data indicates that, of the forms of fatal cancer, pancreatic cancer is the fourth most common (6% of all fatal cancers) [Citation82].
Koong et al. [Citation83] treated patients (N = 15) for cancer of the pancreas using the Cyber-Knife system, in three dose regimes (3 patients treated with 15 Gy, 5 with 20 Gy and 7 with 25 Gy). No acute toxicity was exhibited, and local control was achieved with all patients in the high dose regime
The Cyber-Knife is differentiated from other linac-based stereotactic units by being robotically-mounted with an image guidance system and circularly-collimated fields; there are of the order of 150 Cyber-Knife units worldwide, most of which are in the United States.
Hoyer et al. [Citation84] reported that all patients (N = 22) treated for advanced local pancreatic cancer with 45 Gy in 3 fractions developed grade 2–4 nausea and pain, with the grade 3–4 toxicities including diarrhoea, nausea, pain, mucositis, ulceration.
In a later study, Koong et al. [Citation85] employed 45 Gy IMRT with a single-fraction 25 Gy radiosurgical boost (N = 16). Two patients had acute grade 3 gastroparesis and one patient exhibited late gastrointestinal bleeding.
SBRT of the spine. The spine, being the most common site for bone metastases, may benefit from stereotactic radiosurgery because of the potential to target spinal column metastases whilst sparing critical tissues.
Gerszten et al. [Citation86] treated 500 spinal metastases in 393 patients using SRS, with doses of 12.5–25 Gy to the 80% isodose curve (with a mean dose to the spinal cord of 10 Gy). Long-term pain reduction was reported by 86% of patients.
Gibbs et al. [Citation87] used the Cyber-Knife to deliver 16–25 Gy in 1–5 fractions (N = 74) with a spinal cord limit of 10 Gy. Several cases of severe myelopathy were reported (though two of these patients had received previous radiotherapy treatments); 84% of patients reported resolution or improvement of symptoms.
Ryu et al. [Citation88] used SRS to treat patients (N = 49) using 10–16 Gy (at the 90% isodose curve), with 46% reporting complete pain relief and 19% with partial relief, with a one-year survival of 74%.
Yamada et al. [Citation89] treated patients (N = 93) using 18–24 Gy to the whole vertebrae; achieving local control of 90% (15 month follow-up) and survival of 36% at 45 months.
Concluding thoughts
Stereotactic radio-therapy and -surgery for the treatment of tumours both intracranial and at other anatomical locations is widely employed because of the highly localised doses achievable. Furthermore, it is a highly patient-driven modality, because of its non-invasive nature. However, the approaches described invariably result in the irradiation of significant healthy tissue, despite the small fields involved. SRS (single fraction) and SRT (few fractions) are associated with high doses per fraction compared to other techniques and, as such, accurate localisation is critical. Consequences for irradiated tissue are more significant.
There are many contributing factors that influence the extent of complications in a given treatment. The focus of the present work is that of small-field dosimetry, which – if inaccurate – can lead to complications for the patient. Quality assurance (QA) processes are routine in clinical radiotherapy departments and it would be unusual for a given QA to be performed incorrectly. Rather, the focus of the current paper is to address issues associated with the accuracy of current dose measurement and calculation methodologies as applied to stereotactic radiotherapy. Consider, for example, SBRT of the lung. There is a strong contemporary interest in motion compensation; patient fixation devices typically have accuracies of 2–5 mm. However, even if a tumour is guaranteed to be ‘stationary’ and modern treatment approaches facilitate precise delivery of planned doses – if the accuracy of the calculated dose is poor then this will have direct consequences for the patient. Doses to the periphery of lung tumours may be of the order of 10% lower than at the centre, which is often not accurately predicted by treatment planning systems [Citation90]. It is worth bearing in mind that the use of multiple convergent fields is of course advantageous not only in the reduction of integral healthy tissue dose but also results in a reduction in peripheral under-dosage. The study by Taylor et al. [Citation90] indicates that for circumstances where multiple beams or arcs are employed the aforementioned ∼10% under-dosage at the periphery of lung tumours drops to the order of 5%. This is poorly predicted by conventional treatment planning algorithms such as pencil beam convolution, although better predicted by the analytical anisotropic algorithm. This was highlighted recently by Timmerman et al. [Citation91], who reported on the Radiation Therapy Oncology Group 0236 Phase II trial, whereby the prescribed 20 Gy per fraction dose (totalling 60 Gy) was found to be only 18 Gy per fraction (totalling 54 Gy) – an error that arose because of dose calculation inaccuracy. This highlights the necessity for accurate dose calculation. However, even if accurate dose calculation in treatment planning could be guaranteed, it is still nonetheless subject to the accuracy with which doses were measured during commissioning. Dose calculation and dose measurement are of critical importance.
This necessitates accurate knowledge of dosimetry – particularly of field edges and penumbra, which is difficult in the case of small fields, where the periphery occupies a large fraction of the field area. The complexities with dose prescription, measurement and calculation make understanding the dose characteristics of small fields a challenging task. These issues are discussed in the following sections.
The difficulty of dose prescription
One of the more complex issues in stereotactic radiotherapy is the choice of prescription point or volume. The system of prescribing and reporting described by the International Commission on Radiation Units and Measurements (ICRU) in their reports 52 and 60 is not commonly used for stereotactic procedures [Citation92,Citation93]. While the concepts of clinical target volume (CTV), internal target volume (ITV) and planning target volume (PTV) are well accepted, the use of a reference point for reporting is rare.
The reason for this is illustrated by . If one prescribes to a location in the target such as the isocentre or the point of maximum dose, one would expect that no point in the target receives much less than the prescription dose; typically not more than 5% less [Citation93]. In that case, the edge of the target would align with the 95% isodose curve where the dose fall-off is still relatively shallow. If one selects a lower isodose curve for prescription, the dose gradient is much sharper, leading to better conformality. The sacrifice in this case is reduced target homogeneity – as the maximum dose will typically exceed the prescription dose by over 20%.
Figure 5. A sketch of a tumour and isodose curves illustrating different concepts of dose prescription.
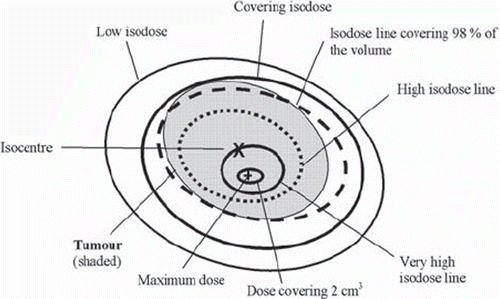
In different countries there is a different emphasis regarding the objective to be achieved when prescribing stereotactic radiotherapy [Citation94–99]. In Japan, the emphasis is on dose homogeneity, in which case prescribing to an ICRU reference point is appropriate. In North America, the preferred approach is to prescribe to an isodose curve covering the target (or the large majority thereof). The latter method is also more common in IMRT, whereby the inverse treatment planning process uses dose volume constraints that are more easily associated with prescription to isodose volumes. The Scandinavian approach in particular deliberately employs quite inhomogeneous dose distributions [Citation100,Citation101].
Ultimately, it is quite difficult to compare the outcomes of clinical series that utilise the same nominal dose but employ different prescription systems. In practice, many approaches are possible, as illustrated in . It is essential that reporting of dose delivered to targets in stereotactic procedures includes a clear description of the prescription rules. As many of the extracranial targets are also subject to motion, this must be taken into consideration when prescribing [Citation93,Citation102].
Small field dosimetry
The complexities of small fields
Small, conformal fields are desirable for the treatment of small lesions. The three-dimensional dose distributions delivered by techniques such as SRS, SRT and IMRT should conform tightly to the PTV. This is particularly so in the case of SRS where the relatively high doses in a single fraction demand strict conformality. The historical limitations in the implementation of small fields may have included technical difficulties of small target localisation and the integration of diagnostic and therapeutic procedures, in addition to a lack of dosimetric data for small fields. Contemporary technology in the field of radiotherapy has overcome many of these technical limitations. However, there is still concern regarding the use of small fields (generally below about 4 × 4 cm2) because of a lack of detailed knowledge about the characteristics of the radiation fields. To deliver small-field treatments with confidence, precise and accurate measurements of the dose profiles, percent depth-dose curves and output factors for small fields are necessary for input into the clinical planning software. There are difficulties associated with this arising from (i) the lack of electronic equilibrium in small fields, and (ii) ‘detector volume averaging’, which results from the finite volume of the detector and has the effect of smoothing of the penumbra.
Calculation of absorbed dose is significantly simplified if a state of ‘radiation equilibrium’ exists in the region of interest. For a detailed discussion of the theory of radiation equilibrium, the interested reader is referred to Ch. IV of Attix , Ch. II of Metcalfe et al. [Citation104,Citation105] or Ch. I (Vol. I) of Kase et al.; though there are many other good references.
In reality, the finite nature of media means that equilibrium conditions are rarely completely met; however, often for (one or more components of) a radiation field, a state of equilibrium may be met, albeit approximately, with a high degree of accuracy. Equivalently to radiation equilibrium, ‘charged particle equilibrium’ exists for a volume if each charged particle of a certain type and energy exiting the volume is replaced by a particle of the same type and energy entering. The International Commission of Radiation Units and Measurements [Citation107] defines charged particle equilibrium as existing when “the energies, number and direction of the charged particles are constant throughout the volume of interest”. The importance of charged particle equilibrium is that, under these conditions, dose (a measurable quantity) may be directly related to the collision kerma (a calculable quantity, proportional to the photon energy fluence).
As one would expect, there is a significant problem relating to electronic disequilibrium of small fields as the lateral range of electrons extends beyond the radius of the field. As a result, small field dosimetry can be inherently difficult – not only to measure, but also to calculate. Furthermore, the density of the medium also influences the degree of disequilibrium. The range of electrons is inversely proportional to the density of the medium in which they travel, hence, for low density media, disequilibrium will exist even for relatively large field sizes. As a result, because of the densities and energies typical of radiotherapy, one must be cautious with fields smaller than about 4 × 4 cm2.
The algorithms used to compute dose in contemporary radiotherapy treatment plans often assume electronic equilibrium (this is discussed in greater detail in section five of the present work). This can result in the erroneous calculation of dose distributions, particularly in the vicinity of inhomogeneities and with small fields. Treatment planning software requires measured dosimetric information as input. The measurement of small fields for this purpose, and for the purpose of delivery verification, is inherently difficult.
Measurement of small-field dose characteristics: Difficulties of conventional methods and the potential for three-dimensional dose verification using radiosensitive gel dosimeters
The difficulty of small-field measurement: Volume averaging. Stereotactic radiosurgery of small tumours was proposed almost 60 years ago [Citation2], but difficulties with the implementation of small fields for radiotherapy still remain, with one significant issue being the measurement of small fields. To perform measurements of sufficient accuracy, the detector should be media-matched and not perturb the radiation field. A high degree of spatial resolution is required and, as such, a detector with a very small sensitive volume is required.
The use of a detector of finite size leads to a detector volume averaging effect. In the case of very small fields, significant errors can occur if the size of the field approaches that of the active volume of the detector. A notable effect (which can occur for any field of sharp dose gradient) is broadening of the penumbrae of the dose profiles. The clinical consequence of this is systematic exposure of larger volumes of healthy tissue in the region of the targeted tumour. It also leads to miscalculation of dose volume histograms (DVH) as well as tumour control and normal tissue complication probabilities (TCP and NTCP respectively) [Citation108].
The complexities of small-field dosimetry is highlighted by the fact that much work is currently going into the development of reference dosimetry protocols specifically for small fields [Citation109].
Available dosimeters and their potential limitations. Ionisation chambers are the standard radiation dosimeter in a clinical medical physics department. Absolute dosimetry with ionisation chambers for small photon fields is often limited by the lack of electronic equilibrium in the radiation field and the size of the chamber cavity volume [Citation110,Citation111]. It is important that the chamber cross-section be smaller than the homogeneous dose regions in which it is placed [Citation112,Citation113]; chamber positioning should be known to better than 1 mm [Citation114]. The finite size of an ionisation chamber introduces a volume averaging effect which can lead to overestimation of penumbra [Citation115,Citation116]. Micro-ionisation chambers have been developed to help overcome the volume limitation. A study of a micro-chamber, Farmer chamber and waterproof scanning chamber showed that the larger the chamber, the greater the under-dose at the field's centre for small fields [Citation117]. Calibration involves the use of broad beams, which means that this dosimetric data may not be directly applicable in the case of small fields. Stopping power ratios facilitate conversion of dose in the chamber cavity to dose in surrounding water. The depth dependence of water/air stopping power ratios has been studied via Monte Carlo simulation showing that, for a 6 MeV photon spectrum, discrepancies of around 1% may exist between broad and narrow fields [Citation110,Citation118,Citation119], with greater disparity for higher energies [Citation120]. A study of a new parallel-plate micro-chamber showed that the dosimeter was under-responsive for small fields, as verified by comparison with Monte Carlo simulation [Citation121]. Martens et al. showed that the PinPoint type ionisation chamber over-responds to low energy Compton scattered photons and is limited to fields greater than 2 cm [Citation122]. Water-proof sleeves for ionisation chambers can result in discrepancies of up to 0.8% depending on the material and beam energy [Citation123]. All these works ultimately indicate that the applicability of ionisation chambers to small field measurements may be limited and a combination of measurement modalities may be appropriate.
The use of smaller detectors may introduce other problems. For instance, semiconductor dosimeters such as silicon diodes and metal oxide semiconductors – field effect transistors (MOSFET) may be used for small field dosimetry. These have the significant advantage of small size and real-time readout. However, diode detectors are not without limitations. These include temperature dependence [Citation124] and dose rate dependence [Citation125], between which there also exists a correlation [Citation126,Citation127]. There is also a directional dependence that arises from the junction geometry [Citation128,Citation129]. There is typically an over-response to low energy components of the spectrum; diodes have been shown to overestimate output factors by up to 7% because of low-energy photons [Citation115]. For this reason, backscatter filters are sometimes used [Citation124]. Correction factors are often needed for the field size, focus to surface distance and phantom thickness [Citation127]. MOSFET dosimetry is achieved via assessment of the radiation damage to the device; the consequence of this is a radiation sensitivity that changes over time and finite life. In general, the characteristics of diodes must be checked, as they differ even for the same type of diode produced by the same manufacturer [Citation130–132].
Kron gives a detailed overview of the application of thermoluminescent detectors (TLD) in dosimetry [Citation104,Citation133]. TLD have the advantage of small size and are routinely used in clinical environments. TLD are considered to be effectively water equivalent [Citation133,Citation134]; the most common material used to construct TLD is lithium fluoride doped with magnesium and titanium (LiF:Mg,Ti), or, more recently with magnesium, copper and phosphorous – resulting in greater sensitivity [Citation135,Citation136]. Sensitivity depends on composition and thermal history, and may vary between and even within batches. Sensitivity has been shown to decrease at a rate of about 1.5% per 10 Gy of absorbed dose [Citation136]. Supralinearity is also an issue and must be corrected for. TLD do not appear to exhibit significant dose-rate dependence up to 108 Gy/s [Citation137]. The TL signal does, however, vary with the radiation quality in a manner that is most significant for low energy photons [Citation104]. The use of high Z-number dopants may lead to over-response because of the greater interaction probability of low-energy photons. Above 1 Gy, the energy response changes according to the energy spectrum of photons and is not readily corrected for, potentially resulting in a loss of precision for high doses. Radiation attenuation within the TL material may also have an effect, as does the readout process and thermal history. Ultimately, the factors relating dose to light emission are many and complex, which means that TLD are often restricted to relative dosimetry. TLD precision down to ± 0.2% is possible [Citation138], but typically a reproducibility of ± 2% for a single rod is achievable with effort [Citation104].
Being very small and suited to use in regions of high dose gradient, diamond detectors have been of recent interest in dosimetry. Simply, these consist of a diamond housed in a small cylinder with a bias applied via two gold contacts – the resistance being inversely proportional to the dose rate of the incident radiation. Theoretical aspects have been studied by Hoban et al [Citation139]. A good overview of the advantages of diamond detectors is provided by Laub et al [Citation140]. They exhibit resistance to radiation damage of 0.05% kGy−1 [Citation141], a sensitivity of approximately 0.05 μC Gy−1 [Citation142] and a stability of 0.67% SD (over 13 weeks) [Citation139]. It has been shown that to obtain sufficient response stability, pre-irradiation of several Gy is required if the detector has not been used for more than one hour. An extra dose is required if the bias was left on while irradiation was interrupted – even for a few minutes. The most apparent problem is dose rate dependence, necessitating the use of correction factors [Citation140].
Films are one of the earliest applied methods of detecting x-rays and their use in a clinical environment is standard practice. One problem with radiographic film is the over-response to low-energy photons [Citation143] resulting from the high atomic number of the active material. There are further processes that may result in darkening of the film during processing that mean film is not readily usable for absolute dosimetry. Underexposure may occur when measuring low-energy photons [Citation144,Citation145] with radiochromic films. Broadly, disadvantages include energy dependence, orientation of radiochromic films, processing conditions, film density variation and inhomogeneities due to air pockets inside the film jacket [Citation146]. The obvious limitation of film is the inability for in vivo measurement and the restriction of two dimensional planar dosimetry. Attempts to obtain 3D detail by stacking radiographic films within a phantom [Citation147] are simple methods to obtain 3D dose information, but are limited by the geometry of the positioning structure and the loss of tissue-equivalence.
Published works dealing with the measurement of small fields using various dosimeters. There are a number of published studies describing the measurement of small fields with various dosimeters. Garcia-Vicente et al. [Citation148] investigated the detector (2 mm diode and 5.5 mm ionisation chamber) size effect in conformal radiotherapy (CRT) and found that organs at risk (OAR) received higher doses when a 5.5 mm detector was used to measure profiles as compared to when a 2 mm detector was used. The NTCP of the brain stem in hypophysis chordoma treatments was doubled when the larger detector was used. Laub and Wong [Citation149] investigated the effect of detector size on the dosimetry of small fields and steep dose gradients in the context of IMRT. They found discrepancies of 10% when comparing measurements taken with film to calculated profiles based on ionisation chamber measurements used in commissioning the IMRT tool. Discrepancies of around 6% were found (at isocentre) when using a 0.6 cm3 Farmer chamber. In the same experimental arrangement, differences of about 2% were found when using a 0.015 cm3 pinpoint ion chamber.
Dawson et al. studied the penumbra of 60Co, 6 MV and 31 MV x-rays using three commercially available detectors (a silicon diode and two ionisation chambers) and a series of in-house ionisation chambers of internal diameters between 0.3 and 1.4 cm. They demonstrated that the width of the penumbrae increase linearly with the internal diameter of the ionisation chamber. True penumbrae were determined via extrapolation. Metcalfe et al. [Citation151] took a similar approach, extrapolating back from measurements taken with multiple detectors of different sensitive volumes. They measured the penumbral width at Dmax with a diode, film and TLD. The sensitive widths of these measurement systems were 2.5, 2.0 and 1.0 mm respectively and the 80–20% penumbra was measured to be 3.6, 3.6 and 3.4 mm respectively. Westermark et al. [Citation115] undertook measurements using a diamond detector, liquid ionisation chamber, plastic scintillator and two Si diodes. One of the diodes was a double-diode using two parallel opposed active volumes with compensating interface perturbations. The volumes of the scintillator and ionisation chamber result in a broadened penumbra. Deviations in output factors varied up to around 10% amongst the detector types. The diamond detector matched the ionisation chamber within 1% for field sizes ranging from 3 × 3 cm2 to 15 × 15 cm2.
Sibata et al. [Citation111] corrected for the ionisation chamber detector size effect in beam profile measurements by extrapolation to zero detector size and a simple convolution method. Higgins et al. [Citation152] also implemented an analytical deconvolution algorithm (similar to those used in radiology applications) to correct for the influence of the finite detector volume on the measured dose. Chang et al. [Citation153] undertook a computational convolution approach to investigate the detector averaging effect. This was found to match penumbrae widths using the backwards extrapolation approach described earlier. Garcia-Vicente et al. [Citation154] determined the spatial convolution kernel for several detectors experimentally, and later used an analytical solution of the integral equation for a general profile fitting function using Gaussian convolution kernels [Citation155]. Van't Veld et al [Citation156]. used the latter model and measured data to determine the detector line spread functions of an ionisation chamber exposed to various energy sources. They found that, following correction, larger volume detectors (such as the IC15, Wellhöfer) can then be used for high resolution relative dosimetry. More recently, Sahoo et al. [Citation157] employed two semi-empirical methods to determine true profiles by elimination of the detector volume averaging effect. The first method shifts the profile based on published deconvolution methods, while the second shifts the measured profile according to the value of an analytical function related to the second derivative of the real profile at a given point.
shows a comparison of different detector types as applied to small-field measurements. The main figure shows the relative central axis dose factors for stereotactic fields as a function of field diameter (or diameter of equivalent area) for a 2 mm plane-parallel ionisation chamber [Citation158], silicon electron diode (Scanditronix, Wellhofer Germany), Kodak EDR2 radiographic film (Kodak Inc., Rochester, NY), micro-MOSFET (Wollongong, Australia), Type 31006 PTW PinPoint cylindrical ionisation chamber (PTW-Freiburg, Germany) and GafChromic Type HS Radiochromic film (ISP Corp., Wayne, NJ). Measurements are in each case normalised to the standard 10 × 10 cm2 reference field. Beneath the main figure is a plot of the same data presented relative to the 2 mm plane-parallel ionisation chamber. Note that for 0.5 cm fields discrepancies vary from a factor of 1.1 up to 1.7, and with increasing field size the disparity between the different measurement techniques decreases. This highlights the complexity of small-field measurement.
Figure 6. The main figure shows a comparison of different detector types as applied to small field measurement, based on data from McNiven et al. [Citation158]. Shown are the relative central axis dose factors for stereotactic fields as a function of field diameter (or diameter of equivalent circle) for a 2 mm plane-parallel ionisation chamber, silicon electron diode, Kodak EDR2 radiographic film, micro-MOSFET, Type 31006 PTW PinPoint cylindrical ionisation chamber and GafChromic Type HS Radiochromic film. Measurements are in each case normalised to the standard 10 × 10 cm2 reference field. Beneath the main figure is a sub-plot of the same data presented relative to the 2 mm plane-parallel ionisation chamber.
![Figure 6. The main figure shows a comparison of different detector types as applied to small field measurement, based on data from McNiven et al. [Citation158]. Shown are the relative central axis dose factors for stereotactic fields as a function of field diameter (or diameter of equivalent circle) for a 2 mm plane-parallel ionisation chamber, silicon electron diode, Kodak EDR2 radiographic film, micro-MOSFET, Type 31006 PTW PinPoint cylindrical ionisation chamber and GafChromic Type HS Radiochromic film. Measurements are in each case normalised to the standard 10 × 10 cm2 reference field. Beneath the main figure is a sub-plot of the same data presented relative to the 2 mm plane-parallel ionisation chamber.](/cms/asset/fc4047f5-3219-4c65-bec2-da7697874cbc/ionc_a_551665_f0006_b.gif)
In summary, there are several key observations that may be made regarding the measurement of small-field dosimetric characteristics using common dosimeters:
In general, there is good agreement between various dosimeter types when measuring beam characteristics of broad beams.
For small fields, however, different dosimeters exhibit poor agreement (deviations of up to 10% may exist).
Measured penumbrae width increase linearly with detector size.
‘True’ penumbrae may be determined indirectly via extrapolation or the use of deconvolution methods following measurement using multiple dosimeters of different effective volumes.
Clinical effects of detector volume average include overdosing of organs-at-risk and inaccurately calculated tumour control probabilities and normal tissue complication probabilities (because TPS calculations use measured data).
Radiosensitive gels for small-field dosimetry. The ideal dosimeter for stereotactic radiotherapy measurements would necessarily possess properties such that it would not be subject to volume averaging issues, as well as being media-matched, so as not to perturb the radiation field. Consequently, gel dosimeters – which comprise both the detector and phantom material – are a very promising tool for investigation of small fields. Their manufacture results in a mass density close to that of water, and the effective atomic number of the vast majority of gel dosimeters closely matches that of water, for both photon and electron interaction processes, over a broad energy range [Citation159,Citation160]. For example, consider the densities of PAGAT gel [Citation161], water and soft tissue: which are 1026, 1000 and 1060 kg.m−3 respectively; the spectrally-weighted effective atomic numbers (for a 6 MV radiotherapy beam) are 3.40, 3.36 and 3.36 respectively [Citation159]. Furthermore, after accurate calibration, gel dosimeters may be employed for absolute dosimetry [Citation162–165]. Read-out of the gels may be performed using magnetic resonance imaging (MRI), optical computed tomography (OCT) or x-ray computed tomography (CT).
There have been a number of publications detailing the application of gel dosimetry to the small fields involved in stereotactic radiotherapy.
Coffey et al. [Citation166] used gel (XO) and TLD dosimetry to verify localisation and dose in a head phantom irradiated using a Gamma-Knife unit. Schulz et al. [Citation167] used Fricke-based gels in a spherical glass phantom to investigate dose distributions from a radiosurgery, reading out data using MRI. Guo et al. [Citation168] evaluated magnetic-resonance-guided Gamma-Knife radiosurgery using a ferrous-sulphate gel dosimeter irradiated using 4 mm collimators and read out via MRI. Cosgrove et al. [Citation169] studied the reproducibility of a polymer-type gel (polyacrylamide gel, PAG) in the context of stereotactic radiotherapy, using plans of varying complexity, using magnetic resonance imaging to read out the gels. The relative dose distributions matched quite well, though the gels measured absolute doses up to >20% higher than the planned doses (attributed to poor calibration). Ertl et al. [Citation170] used a BANG-type polymer gel to measure 8 and 14 mm dose profiles delivered with a Gamma-Knife unit, reading out doses via MRI. Gel measurements agreed well with film and calculated doses. Grebe et al. [Citation171] used polymer gel dosimeters to investigate the small-field doses shaped using a micro-multileaf collimator, in the context of dynamic arc radiosurgery and radiotherapy. Pappas et al. [Citation172] used VIPAR-type gel dosimeters (read out with MRI), radiographic film and a PinPoint ionisation chamber to investigate 5 and 10 mm stereotactic fields. A spatial resolution of 0.13 mm was achieved with the gel, and a significant difference was found between penumbral measurements. The penumbrae from 5 mm and 10 mm collimators were 1.34 mm and 1.70 mm as measured with gel, 2.23 mm and 2.45 mm as measured with film and 2.25 mm and 2.52 mm as measured with the PinPoint chamber.
Audet et al. [Citation173] used two variants of the PAG type polymeric gel to measure stereotactic fields, using an x-ray computed tomography unit to read out the dose. The relative dose volumes for the PAG1 gel were within 3 mm for the 50% and 80% isodose surfaces, while the PAG2 gel exhibited poor contrast. Novotny et al. [Citation174] used polymer gel dosimetry (read out using MRI) to characterise fields of 4–18 mm and a 4 mm field delivered to a rat-brain phantom; results agreed closely with TPS predictions. Novotny et al. [Citation175] used thermoluminescent, semiconductor and gel dosimetry to study Gamma-Knife dose distributions in the context of stereotactic radiotherapy of a rat brain. Watanabe et al. [Citation176] used BANG-type polymer gels to measure Gamma-Knife dose distributions, with a particular focus on the effects of magnetic-resonance image distortion on the obtained dosimetric data. The same phantoms were CT-scanned for comparison. The authors found a 1.3–2.3 mm (or 2 pixel) shift, the magnitude of which varied with receiver bandwidth.
Boudou et al. [Citation177] investigated the dose distributions in synchrotron stereotactic radiotherapy with a Fricke gel dosimeter and Monte Carlo calculations. They found that the measured maximum dose was significantly lower than the calculated dose, which the authors attributed to ionic diffusion within the gel. Isbakan et al. [Citation178] used homogenous and heterogeneous gel phantoms to investigate Gamma-Knife radiosurgery fields, reading out the dose data using MRI. Spatial and dosimetric differences were found between the measured and calculated data for the inhomogeneous case. Papagiannis et al. [Citation179] used polymeric gel dosimeters to study clinical Gamma-Knife fields, using MRI to read out the dosimetric data. Measured and calculated target coverage were 94% and 96% respectively. Watanabe et al [Citation180]. used BANG-type gel dosimeters to calculate the tumour control probability (TCP) and the normal tissue complication probability (NTCP) for Gamma-Knife treatments. TCP values based on measured data were up to 7% smaller than those based on calculated data, while NTCP values were 7–24% higher (for two-thirds of treatments). Pappas et al. [Citation181] investigated detector volume-averaging and perturbation effects by comparing ion-chamber measurements to equivalent volumes in a polymer gel dosimeter, with the objective of differentiating the latter two effects. The authors found that detector composition, not just volume, is an important consideration for small-field measurements.
Boudou et al. [Citation182] continued earlier studies of synchrotron stereotactic radiotherapy dose distributions, using a normoxic polymer gel (nPAG) and Monte Carlo calculations. The gels were irradiated within an anthropomorphic head phantom and read out with magnetic resonance imaging. Isbakan et al. [Citation183] continued earlier studies of Gamma-Knife fields, using a MAGIC-type normoxic polymer gel read out with MRI to investigate small-field dose distributions in the vicinity of inhomogeneities (air pockets). Wong et al. [Citation184] used PAG-type gel dosimeters to study 6 × 6 mm2 and 18 × 18 mm2 fields. The penumbral dose was shown to drop off more rapidly with the gel measured data compared to data obtained using radiochromic film and diode measurements. Geso et al. [Citation185] used PAG-type gel dosimeters and Gafchromic film to measure the dose enhancement in the vicinity of an aneurism clip – as relevant to intracranial stereotactic radiotherapy. Dose increases of the order of 20% were observed close to the clip surface. Results were verified using Monte Carlo dose calculations. Pappas et al. [Citation186] undertook measurement of small fields with a pinpoint ion chamber, a diamond detector and a silicon diode array, using measurements taken with a polymer gel dosimeter as the reference data. Profiles were obtained for 7.5, 15 and 30 mm small fields that were delivered with a BrainLAB conical collimation device mounted on a Varian 600C Clinac. Measurements of the full-width half-maximum (FWHM) obtained using gel were shown to match the nominal fields well, compared to other dosimeters; see . These kinds of measurements are made complex by issues such as alignment of the profile with the field diameter and energy response effects due to scatter-influenced spectral changes. Bjoreland et al. [Citation187] used gel dosimetry to verify treatment plans for AVMs, normalising to liquid ionisation chamber measurements. The measured and calculated dose distributions agreed within 3% and 2 mm for 93% of points in the target volume for all plans studied. Babic et al. [Citation188] used radiochromic ferrous xylenol orange and leuco crystal violet micelle gels to measure relative dose factors and percent depth dose curves for beams of 4.9–28.1 mm produced with a stereotactic collimator mounted on a Varian 2100EX. Data was read out with an optical cone-beam computed tomography scanner and compared to film measurements. Charest et al. [Citation189] evaluated an in-house stereotactic frame for rat-brain irradiation. The authors removed the brains of a rat and filled the cavity with a polymeric gel, which was then irradiated using a Gamma-Knife unit. Pourfallah et al. [Citation190] recently used a PAGAT-type polymer gel dosimeter (read out with MRI) to investigate the influence of inhomogeneities on the dose distribution of Gamma-Knife fields.
Figure 7. (a) The agreement between nominal beam diameters and those measured (FWHM) with VPL radiosensitive gel. The y = x line indicates perfect agreement. (b) A comparison of beam diameters as measured with various dosimeters, expressed as a ratio with the measured data plotted in (a). The PinPoint detector suggests larger penumbra, while the diamond and silicon diode (DOSI) detectors give lower estimates of the penumbra. Based on data from Pappas et al. [Citation186].
![Figure 7. (a) The agreement between nominal beam diameters and those measured (FWHM) with VPL radiosensitive gel. The y = x line indicates perfect agreement. (b) A comparison of beam diameters as measured with various dosimeters, expressed as a ratio with the measured data plotted in (a). The PinPoint detector suggests larger penumbra, while the diamond and silicon diode (DOSI) detectors give lower estimates of the penumbra. Based on data from Pappas et al. [Citation186].](/cms/asset/d86658d1-949e-48df-b2ff-8faac3d036f1/ionc_a_551665_f0007_b.gif)
The large number of studies employing gel dosimetry clearly reflects the recognition of its acceptance for three-dimensional small-field dosimetry in a research context. However – as evidenced by the aforementioned papers – despite being used widely for such applications since the mid-nineties, gel dosimetry is rarely used as part of routine clinical practice. There are a number of limitations associated with gel dosimetry that must be overcome for this to be achieved. For instance, it is often not practical to have a laboratory space within a clinical physics department that facilitates chemical handling and the manufacture of gel dosimeters. The reproducibility of gel dosimeters is also an issue, requiring additional volumes of gel to be made within the same batch for the purposes of calibration. Read-out of gel dosimeters using MRI requires not only development of an associated protocol, but MRI time and access are often difficult or expensive to obtain. The advent of optical computed tomography for gel readout may help solve the latter problem. However, these optical scanners are generally designed for light-absorbing gels such as Fricke gels, which suffer from rapid ion diffusion, and the use of polymeric gel dosimeters is not straightforward, since these are light-scatterers and hence generate artefacts that hide the true dose information.
compares output factors corresponding to 5 mm, 7.5 mm and 10 mm beams from a CyberKnife unit for different detector types. In the case of the 5 mm field, there is good agreement (within uncertainty) between the gel, Gafchromic film and diode. In the case of the diode, there appears to be (insignificant) overestimation of the output factor; this is most likely a consequence of the reduced water equivalence of the Silicon detector, which has been shown to result in such overestimation [Citation191]. The PinPoint detector tends to underestimate the output factors, which is a consequence mostly of volume averaging effects. gives a qualitative overview of the advantages and disadvantages of various detector types for the measurement of small-field dose distributions.
Figure 8. This shows the measured output factors for 5 mm, 7.5 mm and 10 mm beams from a CyberKnife unit. The majority of dosimeters give lower output factors than those measured with gel (VIPAR). For the Gafchromic film [Citation192,Citation193], diode [Citation193–196] and PinPoint chamber [Citation192,Citation194,Citation195] measurements the error bars represent the standard deviation in different published values while the error bars for the gel [Citation192] and TLD measurements [Citation196] correspond to the uncertainty of the published measurements.
![Figure 8. This shows the measured output factors for 5 mm, 7.5 mm and 10 mm beams from a CyberKnife unit. The majority of dosimeters give lower output factors than those measured with gel (VIPAR). For the Gafchromic film [Citation192,Citation193], diode [Citation193–196] and PinPoint chamber [Citation192,Citation194,Citation195] measurements the error bars represent the standard deviation in different published values while the error bars for the gel [Citation192] and TLD measurements [Citation196] correspond to the uncertainty of the published measurements.](/cms/asset/4668ec83-e0db-431a-8005-0a5005e75dcc/ionc_a_551665_f0008_b.gif)
Table II. A qualitative overview of the advantages and disadvantages of the different dosimeters as applied to small field dosimetry.
In addition to the measurement of dose for the purposes of verification, accurate dose calculation is of critical importance in a clinical context. Unfortunately, the complex physics of small-field radiation (particularly in the vicinity of inhomogeneities) means that dose computation using treatment planning systems (TPS) is sometimes of questionable accuracy. Some of the assumptions in TPS algorithms are not always met (consider, in particular, electronic equilibrium), and approximations may not be valid. Although every dose calculation algorithm incorporates some level of approximation, full Monte Carlo dose calculation algorithms are often considered a ‘gold standard’ because they explicitly model the transport of radiation and are frequently cited as the most reliable reference calculation. In the following section, the use of Monte Carlo dose calculation methods is reviewed in the context of stereotactic radiotherapy.
Calculation of small-field dose characteristics
Dose calculation for treatment planning
Initially, treatment planning involved the use of empirical techniques, which limits the treatment geometries that can be employed confidently. Contemporary radiotherapy treatment planning systems provide the capacity to computationally determine the dose to be delivered to a patient. Despite knowledge of radiation transport equations, analytical dose algorithms lack the generality required for use in treatment geometries. Monte Carlo methods are often considered to represent the gold standard in dose calculation, modelling the interactions of all primary radiations and those generated from subsequent secondary particle cascades. This, however, is not widely adopted in a clinical context because of the high demand on the central processing unit (CPU) time. Hence, the option most frequently employed is to adopt semi-analytical algorithms.
A variety of algorithms exist, including what Knoos et al. refer to as ‘type A’ algorithms that employ path-length scaling (such as pencil beam) and ‘type B’ algorithms that incorporate some (approximation of) lateral electron transport (such as AAA). While of acceptable accuracy for many applications, the use of TPS-calculated dose distributions for very small fields is sometimes dubious – including cases involving inhomogeneities. The reason for this is that TPS algorithms incorporate a number of approximations – such as the assumption of electronic equilibrium. Rather than provide a detailed review on the features and accuracy of TPS algorithms (readers are in this case referred to the review by Ahnesjo and Aspradakis [Citation197]), in this section we will concentrate on the application of Monte Carlo methods to small-field dose calculation. There is clearly evidence of a trend towards the inclusion of Monte Carlo modules in planning systems to improve confidence when dealing with cases where TPS accuracy might be limited. This indicates the obvious future reliance on Monte Carlo techniques.
General purpose Monte Carlo radiation transport codes
Monte Carlo radiation transport simulation explicitly models the interaction of individual particles, overcoming many of the limitations of contemporary treatment planning algorithms. The key elements of the Monte Carlo method applied to radiation transport are:
Particles are generated according to distributions describing the radiation source.
A (pseudo-) random number generator provides random numbers in the interval [0,1].
Probability distribution functions based on interaction cross sections are sampled.
Particles are stepped distances between events (collision/scatter).
Each individual outcome is ‘scored’ (accumulated) for specified quantities of interest (such as the dose deposited in a volume).
As the number of particle ‘histories’ that are simulated increases the inherent statistical uncertainty decreases, and thus the better the estimation of the quantity of interest.
There is a general scientific consensus that Monte Carlo dose calculation may be used as a standard by which to compare other calculation or measurement methods. There are numerous available Monte Carlo radiation transport codes that may be employed for the investigation of stereotactic fields. These include the Electron Gamma Shower (EGS) codes (including EGS4, EGS5 and EGSnrc), the Monte Carlo N-Particle (MCNP) code [Citation198], and GEANT4 [Citation199]. These codes were originally developed for high-energy applications. As indicated in , in the context of stereotactic radiotherapy the EGS codes are most frequently employed. Aside from confidence in the accuracy of the transport algorithms, the reason for the widespread use of this code in particular is likely to be due to the easy implementation of the BEAM package, which makes component-by-component modelling of a linear accelerator readily achievable.
Figure 9. A breakdown of 37 relevant scientific papers (employing general Monte Carlo codes) indicates that the Electron Gamma Shower codes are the primary codes employed in the study of stereotactic fields, with EGS4 being employed most frequently in the early 2000s and EGSnrc being employed in the late 2000s.
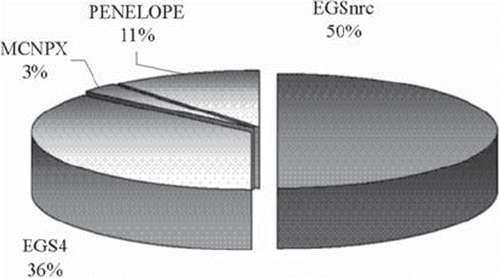
Studies employing EGS4 and EGSnrc for small-field dose calculation
The majority of papers dealing with Monte Carlo calculations in the context of stereotactic radiotherapy from the late 1990s to early 2000s employed EGS4. Heydarian et al. [Citation110] studied field sizes between 7 mm and 23 mm from a Siemens Mevatron, comparing various dosimeters to Monte Carlo. Scielzo et al. [Citation200] investigated SRS treatment planning using Monte Carlo for a Varian 2100C, observing significant differences with the TPS particularly near inhomogeneities. Verhaegen et al. [Citation119] investigated spectral properties for field sizes between 1.25 cm and 5 cm on a Varian 600SR. Monte Carlo calculations agreed with measurements within several percent subsequent to detector volume correction. De Vlamynck et al. [Citation201] compared a diamond detector and parallel plate ionisation chamber to Monte Carlo for an Elekta SL25, observing slight discrepancies in penumbra. Cheung et al. [Citation202] compared GafChromic film to Monte Carlo for field sizes between 4 mm and 18 mm on a Gamma-Knife unit; discrepancies of up to 10% were attributed to issues of energy dependence. Westermark et al. [Citation115] compared various dosimeters against Monte Carlo for field sizes ≥4 mm from a Varian 2100C. Haryanto et al. [Citation203] compared various detectors against Monte Carlo for scatter factors (field sizes of 1–15 cm) on an Elekta Sli. Deng et al. [Citation204] investigated commissioning of Monte Carlo treatment planning for SRS (for a Cyber-Knife unit), comparing measurements to Monte Carlo and attributing an observed difference of 9.5% to detector volume averaging. Paskalev et al. [Citation205] looked at field sizes of 1.5–3 mm from a Varian Clinac-18, comparisons with film showing a maximum discrepancy of 0.3 mm. Deng et al. [Citation206] developed a multiple source model using EGS4 to represent their Cyber-Knife SRS unit (field sizes of 5–60 mm). Tsougos et al. [Citation207] investigated an in-house 6 MV SRT unit design using Monte Carlo methods and various dosimeters. Jin et al. [Citation208] used an EGS4-based code (MCSIM) to optimise SRT beam margins, for a Siemens Primart.
The majority of recent (past several years) publications employ EGSnrc – an evolution of EGS4. Jones et al. [Citation209] studied 0.5–10 cm fields and found that the TPS significantly under- or over-estimated dose with small fields in the vicinity of heterogeneities. Capote et al. [Citation210] compared Monte Carlo calculations to micro-ionisation chamber measurements for a Siemens Primus Clinac, observing differences of up to 9% in the penumbral regions. Jones and Das [Citation211] compared TPS algorithms to Monte Carlo (using published Mohan et al. [Citation212] spectra) and found discrepancies for field sizes under 3 cm. Lydon [Citation213] investigated fields sizes from 1 mm to 30 mm from a Siemens Primus, compared various detectors to Monte Carlo calculations, and found the TPS underestimated doses by up to 45% for the very small fields. Ding et al. [Citation214] described commissioning of Monte Carlo models for SRS applications, comparing calculations with measurements (and finding detector-detector variation) for a Varian Trilogy. Araki et al. [Citation191] investigated field sizes from 5–60 mm for a Cyber-Knife unit, comparing Monte Carlo to measured data. Sterpin et al. [Citation215] compared the anisotropic analytical algorithm (AAA) TPS to Monte Carlo and measurements on an Elekta SL25 linac, generally finding good agreement except near inhomogeneities. Sanchez-Doblado et al. investigated [Citation216] field sizes of 5–10 mm on a Siemens Primus, comparing various detectors against Monte Carlo calculated scatter factors, and showed the influence of spot-size. Cheng et al. [Citation217] investigated field sizes of 1–10 cm on a Varian 2100, extrapolated data to a ‘zero’ field size, and compared Monte Carlo to measured data for tissue maximum ratios. Ding et al. [Citation218] studied the accuracy of TPS calculated dose distribution by comparison to Monte Carlo for clinical fields with a Varian 2100. Paschialis et al [Citation219]. investigated the characteristics of an in-house collimator design using Monte Carlo methods and measured data, for a Siemens Primus. Zhao et al [Citation220]. looked at the small fields (1–2.5 cm) from a TomoTherapy unit, finding that Monte Carlo generally agreed well with film (99.8% of voxels meeting the 2%/2 mm γ-criterion. Scott et al. [Citation221] investigated field sizes from 1 cm to 10 cm from a Varian 2100C, comparing Monte Carlo to various detectors, the latter exhibiting up to 18% variation in output factors for the 0.5 cm field. Sham et al. [Citation222] measured spot sizes from the Varian 21EX, comparing measurements to Monte Carlo for field sizes of 1.5–5 mm. Ding et al. [Citation223] compared Monte Carlo to measurements with a 0.6 mm diode for 7.5–30 mm field sizes. Francescon et al. [Citation195] compared Monte Carlo calculated scatter factors with those measured for field sizes between 5 mm and 10 mm for a Cyber-Knife unit. Heydarian et al. [Citation224] characterised the SRS linac beams from an Elekta Synergy-S using Monte Carlo methods. Scott et al [Citation225]. showed that the output factors for sub-centimetre fields (from a Varian 2100C) are very sensitive to the dimensions of the electron-beam incident on the bremsstrahlung target. For linac-based SRT, Belec et al. and Kairn et al. constructed models of the BrainLAB mini-multileaf collimator with different levels of geometric accuracy and efficiency, both yielding results that match experiment well [Citation226–228]. Moiseenko et al. demonstrated, using EGSnrc, the limited accuracy and questionable appropriateness of simplistic treatment planning algorithms for SBRT of the lung. They found that potentially just three quarters of the PTV is covered by the 95% isodose line.
In summary:
EGS was the Monte Carlo code of choice for stereotactic radiotherapy applications in the 1990s.
Comparisons of EGS to treatment planning calculations revealed discrepancies of up to 5% and 20% for PTVs in homogeneous and heterogeneous regions respectively.
Dosimeter measurements have been compared to EGS for field sizes of the order of millimetres, with the Monte Carlo code used to identify issues of detector volume averaging.
EGSnrc is currently the most frequently employed full Monte Carlo code for stereotactic radiotherapy applications.
EGSnrc has highlighted poor treatment planning system dose calculations, particularly in the vicinity of heterogeneities (in particular lung).
EGSnrc has been used to verify detector measurements of stereotactic fields, and has informed the correction of output factors for small field sizes.
Recalculation of patient plans using EGSnrc has revealed the caution with which one must interpret clinical trial data (where delivered doses are often significantly lower than indicated by the TPS).
Studies employing other available codes for small-field dose calculation
Other codes have also been employed for the study of stereotactic fields, albeit with less frequency than the EGS-based transport codes. Boudou et al. [Citation229] used MCNPX to investigate the potential for synchrotron-based SRT (with beam energies of 50–85 keV). Moskvin et al. [Citation230] verified PENELOPE for Monte Carlo calculation of Gamma-Knife SRS fields against measured data and calculations by other authors (using EGS4). Two years later, Moskvin et al. [Citation231] investigated the effects of inhomogeneities using PENELOPE and a heterogeneous phantom (again, for their Gamma-Knife unit), and found that the TPS underestimated dose by up to 7%. Lax et al. [Citation232] compared pencil beam and collapsed cone-convolution algorithms to PENELOPE calculations (for a Varian 2300CD), finding that the pencil beam in particular significantly overestimated dose. Panettieri et al. [Citation233] compared three TPS algorithms to PENELOPE Monte Carlo calculations for a Varian 2100CD. The TPS were found to overestimate dose by up to 10% in the periphery of the gross-target volume.
Monte Carlo codes optimised for radiotherapy applications
The complexity of non-equilibrium dosimetry means that Monte Carlo calculated small-field dose distributions would be the clinical ideal; however, prohibitively long computation times restrict routine clinical use. The discussion thus far has deliberately focused on general purpose Monte Carlo codes which are capable of modelling a large number of particle types and interaction modes over a very broad energy range. The advent of simplified Monte Carlo TPS algorithms that employ some simplifications and approximations are likely to be an improvement over other contemporary TPS algorithms. These include hybrid approaches in treatment planning systems whereby some component of the calculation is undertaken with Monte Carlo whilst others are undertaken using more computationally efficient algorithms [Citation234,Citation235]. Another strategy is to employ a Monte Carlo code which has been entirely optimised for radiotherapy applications. See the TG105 report for greater detail on the clinical implementation of Monte Carlo [Citation236]. summarises available Monte Carlo codes optimised for radiotherapy. Ultimately, it is probable that a high-efficiency full Monte Carlo model that explicitly simulates all aspects of radiation transport will remain the ultimate desideratum. Such a code, if one could be made to run within a clinically-acceptable timeframe, would be attractive because of its flexibility, in the sense that functionality beyond the most common treatment conditions would be available.
Table III. A summary of different Monte Carlo codes optimised for radiotherapy applications.
Rogers and Mohan [Citation237] suggest comparisons in terms of geometry (both homo- and hetero-generous), uncertainties and issues of approximations in the underlying physics. shows a comparison of simulation times for various full Monte Carlo and treatment planning optimised Monte Carlo codes, relative to EGS4, achieved when using a simple standard geometry specified by Rogers and Mohan. This indicates the speed that may be achieved with the TPS Monte Carlo implementations.
Figure 10. A comparison of the simulation times of various treatment planning optimised and full Monte Carlo radiation transport codes. The data is based on that compiled by Chetty et al. [Citation236]. The times presented are relative to EGS4 (which were performed using the PRESTA algorithm and in a Cartesian geometry, DOSXYZ). The simulations were undertaken for the simple geometry specified by Rogers and Mohan [Citation237], under which conditions the codes generally agreed within 1%. Note the logarithmic scale.
![Figure 10. A comparison of the simulation times of various treatment planning optimised and full Monte Carlo radiation transport codes. The data is based on that compiled by Chetty et al. [Citation236]. The times presented are relative to EGS4 (which were performed using the PRESTA algorithm and in a Cartesian geometry, DOSXYZ). The simulations were undertaken for the simple geometry specified by Rogers and Mohan [Citation237], under which conditions the codes generally agreed within 1%. Note the logarithmic scale.](/cms/asset/652d4131-852e-4d43-8897-25c57f41b0cb/ionc_a_551665_f0010_b.gif)
Ultimately, while such codes will likely be a significant improvement over traditional TPS algorithms, one must be cautious not to make the inference that Monte Carlo dose calculation in a treatment planning system necessarily refers to explicit modelling of all particles and their progeny. The reason for the strong interest in Monte Carlo methods as applied to stereotactic radiotherapy is the increased confidence it gives in the accuracy of calculated dose distribution, which can be very complex for small fields.
Aside from the complexity of in-field doses, TPS algorithms also fail to accurately calculate out-of-field doses, as shall be discussed in the following section.
Out-of-field dose from stereotactic fields
Treatment planning systems (TPS) are normally commissioned using measured data that extend only a few centimetres beyond the field edge, with penumbra defined as 80 to 20% of the maximum dose for the field. Dose extending outside the field is not intended to be used for the overall calculation of the dose distribution or contribute to the inverse optimisation procedure. As a result, one would expect the dose distributions predicted by the TPS to be inaccurate in regions far from the primary field. Even low-dose regions in close proximity to the main treatment field have been shown to be calculated inaccurately by treatment planning systems. In intensity-modulated radiotherapy it is possible for radiation several centimetres beyond the field edge to be within the PTV. Jang et al. [Citation250] showed this recently for intensity-modulated radiotherapy by comparison to Monte Carlo dose calculation using the EGSnrc user code.
There is a significant body of work relating to the measurement and calculation of out-of-field doses for external beam radiotherapy [Citation251]. However, there is relatively little literature pertaining to peripheral doses from stereotactic techniques. While treatments such as intensity-modulated radiotherapy involved doses of a couple of Gy over a large number of fractions to achieve a total of 60–70 Gy, stereotactic radiotherapy often employs doses of 10–20 Gy per fraction in a hypofractionated regime of few fractions. The out-of-field doses from each individual fraction are thus of interest. Most investigations have been concerned with out-of-field doses from SRT rather than SBRT. Ioffe et al. [Citation252] assessed the dose rate as a function of distance from the isocentre in an anthropomorphic phantom for Gamma-Knife treatments. Maarouf et al. [Citation253] estimated the doses received by organs at risk and assessed the risk of late effects (such as secondary tumours or hereditary disorders) following stereotactic linac radiosurgery of intracranial tumours. Thermoluminescent detectors were placed superficially at various anatomic locations of interest, with measured doses in the order of cGy and mGy. Hasanzadeh et al. [Citation254] also undertook TLD measurements in an anthropomorphic phantom, measuring dose in untargeted organs for Gamma-Knife radiosurgery. In the same year, Petti et al. [Citation255] used TLDs to measure the dose at various depths and distances outside the treatment field for Cyber-Knife treatments of a thorax lesion and brain lesion in an anthropomorphic phantom. Peripheral doses were found to be two to five times higher than a comparable Gamma-Knife treatment and up to four times higher than an IMRT treatment, with the relatively large peripheral dose attributed to leakage resulting from the different shielding designs. Chuang et al. [Citation256] investigated reduction of out-of-field doses from the Cyber-Knife system resulting from a shielding upgrade, with the observation that doses were generally reduced by 20 to 55%. Di Betta et al. recently compared out-of-field doses from stereotactic treatments with a linac with MMLC, linac with cones, Cyber-Knife, TomoTherapy and Gamma-Knife. Doses to the testes (for instance) were 0.28 1.1, 1.6, 2.2, 5.8 and 10 mGy for the Gamma-Knife, linac with MMLC, TomoTherapy, linac with cones, Cyber-Knife (with shielding) and Cyber-Knife (no shielding) respectively [Citation257].
The latter studies mentioned have focused on the Cyber-Knife and Gamma-Knife systems. More recently, Taylor et al. [Citation258] investigated out-of-field doses from mini-multileaf collimator shaped fields. The authors found doses of the order of cGy in out-of-field regions – a substantial dose in radiation protection terms – and observed that simple treatment strategies such as aligning the craniocaudal direction of the patient with the x-plane of the collimator can reduce dose by up to an order of magnitude. The latter result was confirmed in a later study of doses in small-field radiotherapy of paediatric patients, whereby Taylor et al. [Citation259] found that doses are on average 40% less along the x-plane, compared to the y-plane (where x- and y-planes are defined by the direction of motion of the x- and y-jaws respectively). Furthermore, the authors also found that far from the primary field about half the out-of-field dose is due to leakage; that the use of a multi-mode linac with a bending magnet resulted in dose about 40% higher than the straight waveguide single-mode unit, and that coplanar treatments with beams avoiding the trunk of the body can reduce dose to organs at risk by an order of magnitude. Ultimately, there has been relatively little study of out-of-field doses from stereotactic fields, and more studies are needed to assess the magnitude of peripheral doses and the potential for radiation-induced complications, such as carcinogenesis, cardiac and respiratory problems. This is particularly so for paediatric patients and those with a non-malignant primary, whose life expectancy is otherwise long.
Conclusions
Despite the concept of stereotactic radiotherapy having existed for half a century, there are nonetheless difficulties associated with the clinical use of small radiation fields resulting from issues of electronic disequilibrium and detector volume averaging effects. An overview of the clinical efficacy of intra- and extra-cranial stereotactic radiotherapy and radiosurgery has been given, with a deliberate emphasis on the potential for detriment. High resolution dosimetry is of key importance, and in this regard three-dimensional gel dosimetry shows great promise for dose measurement. Monte Carlo radiation transport is capable of accurate small-field dose calculation in heterogeneous media. However, the limitations of both gel dosimetry and Monte Carlo techniques currently restrict routine clinical implementation. Improvements in technology including motion compensation strategies and image guidance (including on-board or in-room imaging for image-guided radiotherapy) will serve to increase the use of stereotactic body radiotherapy. The challenges around dosimetry and dose calculation remain of high importance in stereotactic radiotherapy and stereotactic body radiotherapy.
Declaration of interest: The authors report no conflicts of interest. The authors alone are responsible for the content and writing of the paper.
References
- Horsley C, Clarke R. The structure and functions of the cerebellum examined by a new method. Brain 1908;31: 45–124.
- Leksell L. The stereotactic method and radiosurgery of the brain. Acta Chir Scand 1951;102:316–9.
- Yamamoto Y, Coffey R, Nichols D, Shaw E. Interim report on the radiosurgical treatment of cerebral arteriovenous malformations: The influence of size, dose, time and technical factors on obliteration rate. J Neurosurg 1995;83:832–7.
- Steiner L, Lindquist C, Adler J, Torner J, Alves W, Steiner M. Clinical outcome of radiosurgery for cerebral arteriovenous malformations. J Neurosurg 1992;77:1–8.
- Flickinger J, Kondziolka D, Lunsford L. Radiosurgery of benign lesions. Semin Radiat Oncol 1995;5:220–4.
- Leksell L. Stereotactic radiosurgery. J Neurol Neurosurg Psych 1983;46:797–803.
- Selek U, Chang E, Hassenbusch S, Shiu A, Lang F, Allen P, . Stereotactic radiosurgical treatment in 103 patientss for 153 cerebral melanoma metastases. Int J Radiat Oncol Biol Phys 2004;59:1097–106.
- Le Q, Tate D, Koong A, Gibbs I, Chang S, Adler J, . Improved local control with stereotactic radiosurgical boost in patients with nasopharyngeal carcinoma. Int J Radiat Oncol Biol Phys 2003;56:1046–54.
- Goyal L, Suh J, Reddy C, Barnett G. The role of whole brain radiotherapy and stereotactic radiosurgery on brain metastases from renal cell carcinoma. Int J Radiat Oncol Biol Phys 2000;47:1007–12.
- Kulik C, Caudrelier J, Vermandel M, Castelain B, Maouche S, Rousseau J. Conformal radiotherapy optimization with micromultileaf collimators: Comparison with radiosurgery techniques. Int J Radiat Oncol Biol Phys 2002;53: 1038–50.
- Shiu A, Kooy H, Ewton J, Tung S, Wong J, Antes K, . Comparison of miniature multileaf collimation (MMLC) with circular collimation for stereotactic treatment. Int J Radiat Oncol Biol Phys 1997;37:679–88.
- Combs S, Schulz-Ertner D, Thilmann C, Edler L, Debus J. Fractionated stereotactic radiation therapy in the management of primary oligodendroglioma and oligoastrocytoma. Int J Radiat Oncol Biol Phys 2005;62:797–802.
- Aoki M, Abe Y, Hatayama Y, Kondo H, Basaki K. Clinical outcome of hypofractionated conventional conformation radiotherapy for patients with single and no more than three metastatic brain tumors, with noninvasive fixation of the skull without whole brain irradiation. Int J Radiat Oncol Biol Phys 2006;64:414–8.
- Tokuuye K, Akine Y, Sumi M, Kagami Y, Ikeda H, Oyama H, . Reirradiation of brain and skull base tumors with fractionated stereotactic radiotherapy. Int J Radiat Oncol Biol Phys 1998;40:1151–5.
- Benedict S, Carnidale R, Wu Q, Zwicker R, Broaddus W, Mohan R. Intensity-modulated stereotactic radiosurgery using dynamic micro-multileaf collimation. Int J Radiat Oncol Biol Phys 2001;50:751–8.
- Carnidale R, Benedict S, Wu Q, Zwicker R, Gaballa H, Mohan R. A comparison of three stereotactic radiotherapy techniques: Arcs vs concoplanar fixed fields vs intensity modulation. Int J Radiat Oncol Biol Phys 1998;42:431–6.
- Little D, Chang E, Lii M, Maor M, Shiu A. Supplement 2055: Comparison of intensity-modulated and shaped beam conformal stereotactic conformal radiotherapy plans for complex intracranial lesions. Int J Radiat Oncol Biol Phys 2003;57:376.
- Bues M, Kooy H, Hacker F, Tarbell N, Chapman P, Loeffler J. Supplement 2080: Micro-IMRT for stereotactic radiotherapy of intracranial tumours. Int J Radiat Oncol Biol Phys 1999;45:319.
- Pagnini P, Wazer D, Tsai M. Supplement 189: A dosimetric analysis of multiple couch angles optimized dual-dynamic intensity modulated radiotherapy plans for the treatment of intracranial lesions. Int J Radiat Oncol Biol Phys 1999;45: 246.
- Werner-Wasik M, Rudoler S, Preston P, Hauck W, Downes B, Leeper D, . Immediate side effects of stereotactic radiotherapy and radiosurgery. Int J Radiat Oncol Biol Phys 1999;43:299–304.
- Lee A, Ng S, Ho J, Tse V, Poon Y, Tse C, . Clinical diagnosis of late temporal lobe necrosis following radiation therapy for nasopharyngeal carcinoma. Cancer 1988;61: 1535–42.
- Marks L, Spencer D. The influence of volume on the tolerance of the brain to radiosurgery. J Neurosurg 1991;71: 177–80.
- Ianssen T, Lydersen S, Torp S, Juul R. Malignant brain tumors – effect of repeated resection and adjuvant treatment. Int Congr Ser 2004;1259:45–52.
- Jensen A, Brown P, Pollock B, Stafford SL, Link M, Garces Y, . Gamma knife radiosurgery of radiation-induced intracranial tumors: Local control, outcomes, and complications. Int J Rad Biol Phys 2005;62:32–7.
- Korytko T, Radivoyevitch T, Colussi V, Wessels B, Pillai K, Maciunas R, . 12 Gy gamma knife radiosurgical volume is a predictor for radiation necrosis in non-AVM intracranial tumors. Int J Radiat Oncol Biol Phys 2006;64: 419–24.
- Roman D, Sperduto P. Neuropsychological effects of cranial radiation: Current knowledge and future directions. Int J Radiat Oncol Biol Phys 1995;31:983–98.
- Gomori J, Shaked A. Radiation induced meningiomas. Neuroradiology 1982;23:211–2.
- Modan B, Baidatz D, Mart H, Steinitz R, Levin S. Radiation-induced head and neck cancers. Lancet 1974;1:277–9.
- Sadetzki S, Flint-Richter P, Ben-Tal T, Nass D. Radiation-induced meningioma: A descriptive study of 253 cases. J Neurosurg 2002;97:1078–82.
- Ron E, Modan B, Boice J, Alfandary E, Stovall M, Chetrit M, . Tumors of the brain and nervous system after radiotherapy in childhood. N Engl J Med 1988;319:1033–9.
- Shore R, Albert R, Pasternack B. Follow-up study of patients treated by x-ray epilation for tinea capitis. Resurvey of post-treatment illness and mortality experience. Arch Environ Health 1976;31:21–8.
- Sheehan J, Yen C, Steiner L. Gamma knife surgery-induced meningioma. J Neurosurg 2006;105:325–9.
- Brada M, Rajan B, Traish D, Ashley S, Holmes-Sellors P, Nussey S, . The long-term efficacy of conservative surgery and radiotherapy in the control of pituitary adenomas. Clin Endocrinol 1993;38:571–8.
- Minniti G, Traish D, Ashley S, Gonsalves C, Brada M. Risk of second brain tumor after conservative surgery and radiotherapy for pituitary adenoma: Update after an additional 10 years. J Clin Endocrinol Metab 2005;90:800–4.
- Kaido T, Hoshida T, Uranishi R, Akita N, Kotani A, Nishin A, . Radiosurgery induced brain tumor: Case report. J Neurosurg 2001;95:710–3.
- Shamisa A, Bance M, Nag S, Tator C, Wong S, Noren G, . Glioblastoma multiforme occurring in a patient treated with gamma knife surgery: Case report and a review of the literature. J Neurosurg 2001;94:816–21.
- Yu J, Yong W, Wilson D, Black K. Glioblastoma induction after radiosurgery for meningioma. Lancet 2000;356: 1576–7.
- McIver J, Pollock B. Radiation-induced tumor after stereotactic radiosurgery and whole brain radiotherapy: Case report and literature review. J Neuro-Oncol 2004;66:301–5.
- Hamilton A, Lulu B, Fosmire H, Stea B, Cassady J. Preliminary clinical experience with linear-accelerator-based spinal stereotactic radiosurgery. Neurosurgery 1995;36: 311–9.
- Lax I, Blomgren H, Naslund I, Svanstrom R. Stereotactic radiotherapy of malignancies in the abdomen. Acta Oncol 1994;33:677–83.
- Wulf J, Hadinger U, Oppitz U, Olshausen B, Flentje M. Stereotactic radiotherapy of extracranial targets: CT-simulation and accuracy of treatment in the stereotactic body frame. Radiother Oncol 2000;57:225–36.
- Fukumoto S, Shirato H, Shimzu S, Ogura S, Onimaru R, Kitamura K. Small-volume image-guided radiotherapy using hypofractionated, coplanar, and noncoplanar multiple fields for patients with inoperable state I nonsmall cell lung carcinomas. Cancer 2002;95:1546–453.
- Nagata Y, Negoro Y, Aoki M, Mizowaki T, Takayama K, Kokubo M, . Clinical outcomes of 3D conformal hypofractionated single high-dose radiotherapy for one or two lung tumors using a stereotactic body frame. Int J Radiat Oncol Biol Phys 2002;52:1041–6.
- Hof H, Herfath K, Munter M, Hoess A, Motsch J, Wannenmacher M, . Stereotactic single-dose radiotherapy of stage I non-small-cell lung cancer (NSCLC). Int J Radiat Oncol Biol Phys 2003;56:335–41.
- Fuss M, Salter B, Rassiah-Szegedi P, Cheek D, Cavanaugh S, Herman T. Repositioning accuracy of a commercially available double-vacuum whole body immobilisation system for stereotactic body radiation therapy. Tech Cancer Res Treat 2004;3:59–67.
- Wulf J, Haedinger U, Oppitz U. Stereotactic radiotherapy of targets in the lung and liver. Strahlenther Onkol 2001;177: 645–55.
- Herfath K, Debus J, Lohr F, Bahner M, Rhein B, Fritz P, . Stereotactic single-dose radiation therapy of liver tumors: Results of a phase I/II trial. J Clin Oncol 2001;19:164–70.
- Tokuuye K, Sumi M, Ikeda H, Kagami Y, Murayama S, Nakayama HKM, . Technical considerations for fractionated stereotactic radiotherapy of hepatocellular carcinoma. Jpn J Clin Oncol 1997;27:170–3.
- Chang E, Shiu A, Lii M, Rhines L, Mendel E, Mahajan A, . Phase I clinical evaluation of near-simultaneous computed tomographic image-guided stereotactic body radiotherapy for spinal metastases. Int J Radiat Oncol Biol Phys 2004;59:1288–94.
- Lohr F, Debus J, Frank C, Herfath K, Pastyr O, Rhein B, . Noninvasive patient fixation for extracranial stereotactic radiotherapy. Int J Radiat Oncol Biol Phys 1999;45: 521–7.
- Murphy M. An automatic six-degree-of-freedom image registration algorithm for image-guided frameless stereotaxic radiosurgery. Med Phys 1997;24:857–66.
- Hiraoka M, Matsuo Y, Nagata Y. Stereotactic body radiaiton therapy (SBRT) for early-stage lung cancer/Radiothérapie stéréotaxique pour cancer bronchique localisé. Cancer/Radiothérapie 2007;11:32–5.
- Blomgren H, Lax I, Goeranson H, Kraepelien T, Nilsson B, Naslund I, . Radiosurgery for tumors in the body: Clinical experience using a new method. J Radiosurg 1998;1: 63–74.
- Lax I, Blomgren H, Larson D, Naslund I. Extracranial stereotactic radiosurgery of localized target. J Radiosurg 1998;1: 135–48.
- Blomgren H, Lax I, Naslund I. Stereotactic high dose fraction radiation therapy of extracranial tumors using an accelerator: Clinical experience of the first thirty-one patients. Acta Oncol 1995;34:861–70.
- Lee S, Choi E, Park H. Stereotactic body frame based fractionated radiosurgery in the consecutive days for primary and metastatic tumor in the lung. Lung Cancer 2003;40:309–15.
- McGarry R, Pepiez L, Williams M, Whiteford T, Timmerman R. Stereotactic body radiation therapy of early-stage non-small cell lung carcinoma: Phase I study. Int J Radiat Oncol Biol Phys 2005;63:1010–5.
- Nagata Y, Takayama K, Matsuo Y, Norihisa Y, Mizowaki T, Sakamoto T. Clinical outcomes of a Phase I/II study of 48 Gy of stereotactic body radiation therapy in 4 fractions for primary lung cancer using a stereotactic body frame. Int J Radiat Oncol Biol Phys 2005;63:1427–31.
- Nyman J, Johansson K, Hulten U. Stereotactic hypofractionated radiotherapy for stage I non-small cell lung cancer – mature results for medically inoperable patients. Lung Cancer 2006;51:97–103.
- Onishi H, Kuriyama K, Komiyama T, Tanaka S, Sano M, Marino K. Clinical outcomes of stereotactic radiotherapy for Stage I non-small lung cancer using a novel irradiation technique: Patient self-controlled breath-hold and beam switching using a combination of linear accelerator and CT scanner. Lung Cancer 2004;45:45–55.
- Timmerman R, Papiez L, McGarry R, Likes L, DesRosiers C, Frost S, . Extracranial stereotactic radioablation: Results of a phase I study in medically inoperable stage I non-small cell lung cancer. Chest 2003;124:1946–55.
- Uematsu M, Shioda A, Suda A, Fukui T, Ozeki Y, Hama Y. Compted tomography-guided frameless stereotactic radiotherapy for stage I non-small cell lung cancer: A 5 year experience. Int J Radiat Oncol Biol Phys 2001;51:666–70.
- Wulf J, Haedinger U, Oppitz U, Thiele W, Mueller G, Flentje M. Stereotactic radiotherapy for primary lung cancer and pulmonary metastases: A non-invasive treatment approach in medically inoperable patients. Int J Radiat Oncol Biol Phys 2004;60:189–96.
- Zimmerman F, Geinitz H, Schill S, Grosu A, Schratzenstaller U, Molls M, . Stereotactic hypofractionated radiation therapy for stage I non-small cell lung cancer. Lung Cancer 2005;48:107–14.
- Nagata Y, Matsuo Y, Takayama K, Norihisa Y, Mizowaki T, Sakamoto M, . Survey of SBRT in Japan. Int J Radiat Oncol Biol Phys 2006;66:150–1.
- Nagata Y, Matsuo Y, Takayama K, Norihisa Y, Mizowaki T, Mitsumori M, . Current status of stereotactic body radiotherapy for lung cancer. Int J Clin Oncol 2007;12:3–7.
- Hurkmans C, Cuijpers J, Lagerwaard F, Widder J, ver der Heide U, Schuring D, . Recommendations for implementing stereotactic radiotherapy in peripheral stage 1A non-small cell lung cancer: Report for the Quality Assurance Working Party of the randomised phase III ROSEL study. Radiat Oncol 2009;4:1–14.
- Chi A, Tome W, Fowler J, Kamaki R, Nguyen N, Mehta M, . Stereotactic body radiation therapy in non-small-cell lung cancer: Linking radiobiological modelling and clinical outcomes. Am J Clin Oncol 2010 (in press).
- Milano M, Constine L, Okunieff P. Normal tissue toxicity after small hypofractionated stereotactic body irradiation. Radiat Oncol 2008;3:36–46.
- Le Q, Loo B, Ho A, Cotrutz C, Koong A, Wakalee H, . Results of a phase I dose-escalation study using single-fraction stereotactic radiotherapy for lung tumors. J Thorac Oncol 2006;1:802–9.
- Milano M, Katz A, Muhs A, Philip A, Buchholz D, Schell M, . A prospective pilot study of curative-intent stereotactic body radiation therapy in patients with 5 or fewer oligometastatic lesions. Cancer 2008;112:650–8.
- Onimaru R, Shirato H, Shimizu M, Kitamura K, Xu B, Fukumoto S, . Tolerance of organs at risk in small-volume, hypofractionated, image-guided radiotherapy for primary and metastatic lung cancers. Int J Radiat Oncol Biol Phys 2003;56:126–35.
- Timmerman R, McGarry R, Yiannoutsos C, Papiez L, Tudor K, DeLuca J, . Excessive toxicity when treating central tumors in a phase II study of stereotactic body radiation therapy for medically inoperable early-stage lung cancer. J Clin Oncol 2006;24:4833–9.
- Timmerman R, Paulus R, Galvin J, Michalski J, Straube W, Bradley J, . Toxicity analysis of RTOG 0236 using stereotactic body radiation therapy to treat medically inoperable early stage lung cancer patients. Int J Radiat Oncol Biol Phys 2007;69:S86.
- Zimmerman F, Geinitz H, Schill S, Thamm R, Nieder C, Schratzenstaller U, . Stereotactic hypofractionated radiotherapy in stage I (T1-2 N0 M0) non-small-cell lung cancer (NSCLC). Acta Oncol 2006;45:796–801.
- Herfarth K, Münter M. A review of stereotactic body radiotherapy for liver and pancreas. Treating tumors that move with respiration. Berlin: Springer; 2007. 195–203.
- Wulf J, Gluckenberger M, Haedinger U. Stereotactic radiotherapy of primary liver cancer and hepatic metastases. Acta Oncol 2006;45:838–47.
- Kavanagh B, Schefter T, Cardenes H, Stieber V, Raben D, Timmerman R, . Interim analysis of a prospective phase I/II trial of SBRT for liver metastases. Acta Oncol 2006;45: 848–55.
- Hoyer M, Roed H, Traberg A, Oholhuis L, Peterson J, Nellemann H, . Phase II study on stereotactic body radiotherapy of colorectal metastases. Acta Oncol 2006;45: 823–30.
- Casamassina F, Masi L, Menichelli C, D'Imporanzo E, Polli C, Bonucci I. IGRT stereotactic hypofractionated radiotherapy for the treatment of focal liver malignancies. Int J Radiat Oncol Biol Phys 2008;72:S277–S278.
- Tse R, Hawkins M, Lockwood G, Kim J, Cummings B, Knox J, . Phase I study of individualized stereotactic body radiotherapy for hepatocellular carcinoma and intrahepatic cholangiocarcinoma. J Clin Oncol 2008;26:657–64.
- Jemel A, Siegel R, Ward E, Hao Y, Xu J, Thun M. Cancer Statistics, 2009. CA Cancer J Clin 2009;59:1–25.
- Koong A, Le Q, Ho A, Fong B, Fisher G, Cho C, . Phase I study of stereotactic radiosurgery in patients with locally advanced pancreatic cancer. Int J Radiat Oncol Biol Phys 2004;58:1017–21.
- Hoyer M, Roaed H, Sengelov L, Traberg A, Oholhuis L, Pederson T, . Phase-II study on stereotactic radiotherapy of locally advanced pancreatic carcinoma. Radiother Oncol 2005;76:48–53.
- Koong A, Christofferson E, Le Q, Goodman K, Ho A, Kuo T, . Phase II study to assess the efficacy of conventionally fractionated radiotherapy followed by a stereoatcic radiosurger boost in patients with locally advanced pancreatic cancer. Int J Radiat Oncol Biol Phys 2005;63:320–3.
- Gerszten P, Burton S, Ozhasoglu C, Welch W. Radiosurgery for spinal metastases: Clinical experience in 500 cases from a single institution. Spine 2007;32:193–9.
- Gibbs I, Kamnerdsupaphon P, Ryu M, Dodd R, Kiernan M, Chang SA. Image-guided robotic radiosurgery for spinal metastases. Radiother Oncol 2007;82:185–90.
- Ryu S, Jin R, Jin J, Chen Q, Rock K, Anderson J, . Pain control by image-guided radiosurgery for solitary spine metastases. Radiother Oncol 2008;82:185–90.
- Yamada Y, Bilsky M, Lovelock M, Venkatraman E, Toner S, Johnson J, . High-dose, single-fraction image-guided intensity-modulated radiotherapy for metastatic spine lesions. Int J Radiat Oncol Biol Phys 2008;71:484–90.
- Taylor M, Dunn L, Kron T, Franich R. Dose inhomogeneity in radiotherapy of lung tumours: Calculation of peripheral underdosage. Radiother Oncol 2010;96:S341–S342.
- Timmerman R, Paulus R, Galvin J, Michalski J, Straube W, Bradley J, . RTOG 0236: Stereotactic body radiation therapy to treat medically inoperable early stage lung caner patients. 13th World Conference on Lung Cancer. San Francisco, USA: 2009.
- ICRU. Report No 50: Prescribing, recording and reporting photon beam therapy. Bethesda, MD: International Commission of Radiation Units and Measurements; 1993.
- ICRU. Report No. 62: Prescribing, recording and reporting photon beam therapy (supplement to Report No. 52). Bethesda USA: International Commission of Radiation Units and Measurements; 2000.
- Hiraoka M, Nagata Y. Stereotactic body radiation therapy for early-stage non-small-cell lung cancer: The Japanese experience. Int J Clin Oncol 2004;9:352–5.
- Nagata Y, Hiraoka M, Mizowaki T, Narita Y, Matsuo Y, Norihisa Y, . Survey of stereotactic body radiation therapy in Japan by the Japan 3-D Conformal External Beam Radiotherapy Group. Int J Radiat Oncol Biol Phys 2009;75: 343–7.
- Nagata Y, Matsuo Y, Takayama K, Norihisa Y, Mizowaki T, Mitsumori M, . Current status of stereotactic body radiotherapy for lung cancer. Int J Clin Oncol 2007; 12:3–7.
- Fakiris AJ, McGarry RC, Yiannoutsos CT, Papiez L, Williams M, Henderson MA, . Stereotactic body radiation therapy for early-stage non-small-cell lung carcinoma: Four-year results of a prospective phase II study. Int J Radiat Oncol Biol Phys 2009;75:677–82.
- Timmerman RD, Kavanagh BD, Cho LC, Papiez L, Xing L. Stereotactic body radiation therapy in multiple organ sites. J Clin Oncol 2007;25:947–52.
- Timmerman RD, Park C, Kavanagh BD. The North American experience with stereotactic body radiation therapy in non-small cell lung cancer. J Thorac Oncol 2007;2 (7 Suppl 3):S101–S112.
- Lax I. Target dose versus extratarget dose in stereotactic radiosurgery. Acta Oncol 1993;32:453–7.
- Baumann P, Nyman J, Lax I, Friesland S, Hoyer M, Rehn Ericsson S, . Factors important for efficacy of stereotactic body radiotherapy of medically inoperable stage I lung cancer. A retrospective analysis of patients treated in the Nordic countries. Acta Oncol 2006;45:787–95.
- Admiraal MA, Schuring D, Hurkmans CW. Dose calculations accounting for breathing motion in stereotactic lung radiotherapy based on 4D-CT and the internal target volume. Radiother Oncol 2008;86:55–60.
- Attix F. Introduction to radiological physics and radiation dosimetry. Weinheim: Wiley-VCH; 2004.
- Metcalfe P, Kron T, Hoban P. The physics of radiotherapy x-rays from linear accelerators. Madison, WI: Medical Physics Publishing; 1997.
- Metcalfe P, Kron T, Hoban P. The physics of radiotherapy x-rays and electrons. Madison, WI: Medical Physics Publishing; 2007.
- Kase K, Bjarngard B, Attix F. The dosimetry of ionising radiation. Florida, USA: Academic Press; 1987.
- ICRU. Report No 33: Radiation quantities and units. Washington, DC: International Commission of Radiation Units and Measurements; 1980.
- Garcia-Vicente F, Bejar M, Perez L, Torres J. Clinical impact of the detector size effect in 3D-CRT. Radiother Oncol 2005;74:315–22.
- Alfonso R, Andreo P, Capote R, Izewska J, Meghzifene A, Huq M, . Present Status of IAEA/AAPM Recommendations on Small and Composite Field Dosimetry. Med Phys 2010;37:3096.
- Heydarian A, Hoban P, Beddoe A. A comparison of dosimetry techniques in stereotactic radiosurgery. Phys Med Biol 1996;41:93–110.
- Sibata C, Mota H, Beddar A, Higgins P, Shin K. Influence of detector size in photon beam profile measurements. Phys Med Biol 1991;36:621–31.
- Boyer A. Intensity modulated radiotherapy: Current status and issues of interest. Int J Radiat Oncol Biol Phys 2001;51: 880–914.
- Bjarngard C, Tsai J, Rice R. Doses on the central axes of narrow 6 MV x-ray beams. Med Phys 1990;17:794–9.
- Low D, Mutic S, Dempsey J, Gerber R, Bosch W, Perez C, . Quantitative dosimetric verification of an IMRT planning and delivery system. Radiother Oncol 1998;49: 305–16.
- Westermark M, Arndt J, Nilsson B, Brahme A. Comparative dosimetry in narrow high-energy photon beams. Phys Med Biol 2000;45:685–702.
- Rice R, Hansen J, Svensson G, Siddon R. Measurements of dose distributions in small beams of 6 MV x-rays. Phys Med Biol 1987;32:1087–99.
- Low D, Parikh P, Dempsey J, Wahab S, Huq S. Ionization chamber volume averaging effects in intensity modulated radiation therapy beams. Med Phys 2003;30:1706–11.
- Andreo P, Brahme A. Stopping power data for high energy photon beams. Phys Med Biol 1986;31:839–58.
- Verhaegen F, Das I, Palmans H. Monte Carlo dosimetry study of a 6 MV stereotactic unit. Phys Med Biol 1998;43: 2755–68.
- Sanchez-Doblado F, Andreo P, Capote R, Leal A, Perucha M, Arrans R, . Ionization chamber dosimetry of small radiation fields: A Monte Carlo study on stopping power ratios for radiosurgery and IMRT beams. Phys Med Biol 2003;48:2081–99.
- Francescon P, Cora S, Cavendon C, Scalchi C, Reccanello S, Colombo F. Use of a new type of radiochromic film, parallel-plate microchamber, MOSFETs, and TLD 800 microcubes in the dosimetry of small beams. Med Phys 1998;25:503–11.
- Martens C, DeWagter C, DeNeve W. The value of the PinPoint ion chamber for characterization of small field segments used in intensity-modulated radiation therapy. Phys Med Biol 2000;45:2519–30.
- Ross C, Shortt K. The effect of waterproofing sleeves on ionization chamber response. Phys Med Biol 1992;37: 1403–11.
- Grusell E, Rikner G. Evaluation of temperature effects in p-type silicon detectors. Phys Med Biol 1986;31:527.
- Wilkins D, Li X, Cygler J, Gerig L. The effect of dose rate dependence of p-type silicon detectors on linac relative dosimetry. Med Phys 1997;24:879–81.
- VanDam J, Leunens G, Dutreix A. Correlation between temperature and dose-rate dependence of semiconductor response: Influence of accumulated dose. Radiother Oncol 1990;19:345–51.
- Heukelom S, Lanson J, Mijnheer B. Comparison of entrance and exit dose measurements using ionization chambers and silicon diodes. Phys Med Biol 1991;36:47–59.
- Higgins P, Alaei P, Gerbi B, Dusenbery K. In vivo diode dosimetry for routine quality assurance in IMRT. Med Phys 2003;30:3118–23.
- Bjork P, Knoos T, Nilsson P. Comparative dosimetry of diode and diamond detectors in electron beams for intraoperative radiation therapy. Med Phys 2000;27:2580–8.
- Leunens G, VanDam J, Dutreix A, Scheuren Evd. Quality assurance in radiotherapy by in vivo dosimetry: Entrance dose measurements, a reliable procedure. Radiother Oncol 1990;17:141–51.
- Li C, Lamel L, Tom D. A patient dose verification program using diode detectors. Med Dosim 1995;20:209–14.
- Alecu R, Loomis T, Alecu J, Cochran T. Guidelines on the implementation of diode in vivo dosimetry programs for photon and electron external beam therapy. Med Dosim 1999;24:5–12.
- Kron T. Thermoluminescence dosimetry and its applications in medicine—Part 1: Physics, materials and equipment. Aust Phys Eng Sci Med 1994;17:175–99.
- Horowitz Y. Thermoluminescence and thermoluminescent dosimetry. Florida: Boca Raton; 1984.
- Delgado A, Gomez Ros J, Muniz J. Computerised analysis of LiF GR-200 TL signals: Application to dose measurements in the micro Gy range. Radiat Protect Dosim 1995; 60:147–53.
- Horowitz Y. LiF:Mg, Ti versus LiF:Mg, Cu, P: The competition heats up. Radiat Protect Dosim 1993;47:135–41.
- Tochilin E, Goldstein N. Dose rate and spectral measurements from pulsed x-ray generators. Health Phys 1966;47: 497–503.
- Martenssen B. Thermoluminescence of LiF: A statistical analysis of the influence of pre-annealing on the precision of measurements. Phys Med Biol 1969;14:119–30.
- Hoban P, Heydarian M, Beckham W, Beddoe A. Dose rate dependence of a PTW diamond detector in the dosimetry of a 6 MV photon beam. Phys Med Biol 1994;39: 1219–29.
- Laub W, Kaulich T, Nusslin F. A diamond detector in the dosimetry of high-energy electron and photon beams. Phys Med Biol 1999;44:2183–92.
- Planskoy B. Evaluation of diamond radiation dosimeters. Phys Med Biol 1980;25:519–32.
- Vatnitsky S, Jarvinen H. Application of a natural diamond detector for the measurement of relative dose distributions in radiotherapy. Phys Med Biol 1993;38:173–84.
- Butson M, Cheung T, Yu K. Corresponding dose response of radiographic film with layered Gafchromic film. Phys Med Biol 2002;47:N285–N289.
- Kron T, Duggan L, Smith T, Rosenfeld A, Butson M, Kaplan G, . Dose response of various radiation detectors to synchrotron radiation. Phys Med Biol 1998;43: 3235–59.
- Muench P, Meigooni A, Nath R, McLaughlin W. Photon energy dependence of the sensitivity of radiochromic film and comparison with silver halide and LiF TLDs used for brachytherapy dosimetry. Med Phys 1991;18:769–75.
- Cheng C, Das I. Dosimetry of high energy photon and electron beams. Med Phys 1996;23:1225–32.
- Robar J, Clark B. A practical technique for verification of three-dimensional conformal dose distributions in stereotactic radiosurgery. Med Phys 2000;27:978–87.
- Garcia-Vincente F, Bejar M, Perez L, Torres J. Clinical impact of the detector size effect in 3D-CRT. Radiother Oncol 2005;74:315–22.
- Laub W, Wong T. The volume effect of detectors in the dosimetry of small fields used in IMRT. Med Phys 2003;30: 341–7.
- Dawson D, Harper J, Akinradewo A. Analysis of physical parameters associated with the measurment of high-energy x-ray penumbra. Med Phys 1984;11:491–7.
- Metcalfe P, Kron T, Elliot A, Wong T, Hoban P. Dosimetry of 6 MV x-ray beam penumbra. Med Phys 1993;20: 1439–45.
- Higgins P, Sibata C, Siskind L, Sohn J. Deconvolution of detector size effect for small field measurement. Med Phys 1995;22:1663–6.
- Chang K, Yin F, Nie K. The effect of detector size to the broadening of the penumbra – a computer simulated study. Med Phys 1996;23:1407–11.
- Garcia-Vicente F, Degado J, Peraza C. Experimental determination of the convolution kernel for the study of the spatial response of a detector. Med Phys 1998;25:202–7.
- Garcia-Vicente F, Delgado A, Rodriguez R. Exact analytical solution of the convolution integral equation for a general profile fitting function and Gaussian detector kernel. Phys Med Biol 2000;45:645–50.
- Van't Veld A, Van Luijk P, Praamstra F, Van der Hulst P. Detector line spread functions determined analytically by transport of Compton recoil electrons. Med Phys 2001;28: 738–51.
- Sahoo N, Kazi A, Hoffman M. Semi-empirical procedures for correcting detector size effect on clinical MV x-ray beam profiles. Med Phys 2008;35:5124–33.
- McNiven A, Mulligan M, Kron T, Battista J. The response of protoype plane-parallel ionization chambers in small megavoltage x-ray fields. Med Phys 2006;33:3997–4004.
- Taylor ML, Franich RD, Trapp JV, Johnston PN. The effective number of dosimetric gels. Australas Phys Eng Sci Med 2008;31:131–8.
- Taylor ML, Franich RD, Trapp JV, Johnston PN. Electron interaction with gel dosimeters: Effective atomic numbers for collisional, radiative and total interaction processes. Radiat Res 2009;171:123–6.
- Venning A, Hill B, Brindha S, Healy B, Baldock C. Investigation of the PAGAT polymer gel dosimeter using magnetic resonance imaging. Phys Med Biol 2005;50: 3875–88.
- Baldock C, Murry P, Kron T. Uncertainty analysis in polymer gel dosimetry. Phys Med Biol 1999;44:N243–N246.
- Taylor ML, Franich RD, Trapp JV, Johnston PN. A comparative study on the effect of calibration conditions on the water equivalence of a range of gel dosimeters. IEEE Trans Nucl Sci 2009;56:429–36.
- Taylor ML, Franich RD, Johnston PN, Millar RM, Trapp JV. Systematic variations in polymer gel dosimeter calibration due to container influence and deviations from water equivalence. Phys Med Biol 2007;52:3991–4005.
- Trapp J, Michael G, Evans P, Baldock C, Leach M, Webb S. Dose resolution in gel dosimetry: effect of uncertainty in the calibration function. Phys Med Biol 2004;49: N139–N146.
- Coffey C, Sanders M, Cashon K, Miller R, Walsh J, Patel P. A tissue equivalent phantom for stereotactic radiosurgery localization and dose verification. Stereotact Funct Neurosurg 1993;61:130–41.
- Schulz R, Maryanski M, Ibbott G, Bond J. Assessment of the accuracy of stereotactic radiosurgery using Fricke- infused gels and MRI. Med Phys 1993;20:1731–4.
- Guo W, Chu W, Wu M, Chung W, Gwan W, Lee Y, . An evaluation of the accuracy of magnetic-resonance-guided Gamma Knife surgery. Stereotact Funct Neurosurg 1996; 66:85–92.
- Cosgrove V, Murphy P, McJury M, Adams E, Warrington A, Leach M, . The reproducibility of polyacrylamide gel dosimetry applied to stereotactic conformal radiotherapy. Phys Med Biol 2000;45:1195–210.
- Ertl A, Berg A, Zehetmayer M, Frigo P. High-resolution dose profile studies based on MR imaging with polymer BANG™ gels in stereotactic radiaiton techniques. Magn Reson Imag 2000;18:343–9.
- Grebe G, Pfaender M, Roll M, Leudemann L, Wurm R. Dynamic arc radiosurgery and radiotherapy: Commissioning and verification of dose distributions. Int J Radiat Oncol Biol Phys 2001;49:1451–60.
- Pappas E, Seimenis I, Angelopoulos A, Georgolopoulou P, Kamariotaki-Paparigopoulou M, Maris T, . Narrow stereotactic beam profile measurements using N-vinylpyrrolidone based polymer gels and magnetic resonance imaging. Phys Med Biol 2001;46:783–97.
- Audet C, Hilts M, Jirasek A, Duzenli C. CT gel dosimetry technique: Comparison of a planned and measured 3D stereotactic dose volume. J Appl Clin Med Phys 2002;3: 110–8.
- Novotny JJ, Novotny J, Spevacek V, Dvorak P, Cechak T, Liscak R, . Application of polymer gel dosimetry in gamma knife radiosurgery. J Neurosurg 2002;97:556–62.
- Novotny JJ, Novotny J, Spevacek V, Dvorak P, Cechak T, Liscak R, . Evaluation of geometric and dosimetric inaccuracies of stereotactic irradiation in the rat brain. Stereotact Funct Neurosurg 2002;79:57–74.
- Watanabe Y, Perera G, Mooij R. Image distortion in MRI-based polymer gel dosimetry of gamma knife stereotactic radiosurgery systems. Med Phys 2002;29:797–802.
- Boudou C, Biston M, Corde S, Adam J, Ferrero C, Esteve F, . Synchrotron stereotactic radiotherapy: Dosimetry by Fricke gel and Monte Carlo simulations. Phys Med Biol 2004;21:5135–44.
- Isbakan F, Buyuksarac B, Agus O, Ulgen Y, Bilge H, Ozen Z. Relative dose distribution in gamma knife treatment near tissue inhomogeneities. Conf Proc IEEE Eng Med Biol Soc 2005;3:3086–9.
- Papagiannis P, Karaiskos P, Kozicki M, Rosiak J, Sakelliou L, Sandilos P, . Three-dimensional dose verification of the clinical application of gamma knife stereotactic radiosurgery using polymer gel and MRI. Phys Med Biol 2005;50: 1979–90.
- Watanabe Y, Akimitsu T, Hirokawa Y, Mooij R, Perera G. Evaluation of dose delivery accuracy of Gamma Knife by polymer gel dosimetry. J Appl Clin Med Phys 2005;6: 133–42.
- Pappas E, Maris T, Papadakis A, Zacharopoulou F, Damilakis J, Papanikolaou N, . Experimental determination of the effect of detector size on profile measurements in narrow photon beams. Med Phys 2006;33:3700–10.
- Boudou C, Tropres I, Rousseau J, Lamalle L, Adam J, Esteve F, . Polymer gel dosimetry for synchrotron stereotactic radiotherapy and iodine dose-enhancement measurements. Phys Med Biol 2007;52:4881–92.
- Isbakan F, Ulgen Y, Bilge H, Ozen Z, Agus O, Buyuksarac B. Gamma-Knife 3-D dose distribution near the area of tissue inhomogeneities by normoxic gel dosimetry. Med Phys 2007;34:1623–30.
- Wong C, Ackerly T, He C, Patterson M, Powell C, Ho A, . High-resolution measurements of small field beams using polymer gels. Appl Radiat Isot 2007;65:1160–4.
- Geso M, Ackerly T, Brown S, Chua Z, He C, Wong C, . Determination of dosimetric perturbations caused by aneurysm clip in stereotactic radiosurgery using gel phantoms and EBT-Gafchromic films. Med Phys 2008;35: 744–52.
- Pappas E, Maris T, Zacharopoulou F, Papadakis A. Small SRS photon field profile dosimetry performed using a PinPoint air ion chamber, a diamond detector, a novel silicon-diode array (DOSI), and polymer gel dosimetry. Analysis and intercomparison. Med Phys 2008;35:4640–8.
- Bjoreland A, Lindvall P, Karlsson A, Gustavsson H, Back S, Karlsson M, . Liquid ionization chamber calibrated dosimetry in conformal stereotactic radiotherapy of brain lesions. Acta Oncol 2008;47:1099–109.
- Babic S, McNiven A, Battista J, Jordan K. Three- dimensional dosimetry of small megavoltage radiation fields using radiochromic gels and optical CT scanning. Phys Med Biol 2009;54:2463–81.
- Charest G, Mathieu D, Lepage M, Fortin D, Paquette B, Sanche L. Polymer gel in rat skull to assess the accuracy of a new rat stereotactic device for use with the gamma knife. Acta Neurochir 2009;151:677–83.
- Pourfallah T, Allahverdi M, Alam N, Ay M, Zahmatkesh M. Differential dose volume histograms of Gamma knife in the presence of inhomogeneities using MRI-polymer gel dosimetry and MC simulation. Med Phys 2009;36: 3002–12.
- Araki F. Monte Carlo study of a Cyberknife stereotactic radiosurgery system. Med Phys 2006;33:2955–63.
- Pantelis E, Antypas C, Petrokokkinos L, Karaiskos P, Papagiannis P, Kozicki M, . Dosimetric characterisation of CyberKnife radiosurgical photon beams using polymer gels. Med Phys 2008;6:2312–20.
- Wilcox E, Daskalov G. Evaluation of GAFCHROMIC EBT film for CyberKnife dosimetry. Med Phys 2007;34: 1983–8.
- Francescon P, Cora S, Cavedon C, Scalchi P, Stancanello J. CyberKnife dosimetric beam characteristics: Comparison between experimental results and Monte Carlo simulation. Robotic Radiosurgery. Sunnyvale CA: CyberKnife Society Press; 2005. 271–80.
- Francescon P, Cora S, Cavendon C. Total scatter factors for small beams: A multidetector and Monte Carlo study. Med Phys 2008;35:504–13.
- Yu C, Jozsef G, Apuzzo M, Petrovich Z. Measurements of the relative output factors for CyberKnife collimators. Neurosurgery 2004;54:157–62.
- Ahnesjo A, Aspradakis M. Dose calculations for external photon beams in radiotherapy. Phys Med Biol 1999;44: R99–R155.
- Team X-MC. MCNP – A general Monte Carlo N-particle transport code (version 5). Los Alamos National Laboratory Report 2003;LA-UR-03-1987.
- Agostinelli S, Allison J, Amako K, Apostolakis J, Araujo H, Arce P, . G4 – a simulation toolkit. Nucl Instr Meth Phys Res A 2003;506:250–303.
- Scielzo G, Grillo Ruggieri F, Schwarz M, Rivolta A, Brunelli B, Surridge M, . The Monte Carlo method and parallel estimation in the drawing up of radiosurgery treatment plans. Radiol Med 1998;95:647–55.
- De Vlamynck K, Palmans H, Verhaegen F, De Wagter C, De Neve W, Thierens H. Dose measurements compared with Monte Carlo simulations of narrow 6 MV multileaf collimator shaped photon beams. Med Phys 1999;26:1874–82.
- Cheung Y, Yu K, Ho R, Yu C. Stereotactic dose planning system used in Leksell Gamma Knife model-B: EGS4 Monte Carlo versus GafChromic films MD-55. Appl Radiat Isot 2000;53:427–30.
- Haryanto F, Fippel ML, W, Dohm O, Nusslin F. Investigation of photon beam output factors for conformal radiation therapy – Monte Carlo simulations and measurements. Phys Med Biol 2002;47:N133–N143.
- Deng J, Ma C, Hai J, Nath R. Commissioning 6 MV photon beams of a stereotactic radiosurgery system for Monte Carlo treatment planning. Med Phys 2003;30:3124–34.
- Paskalev K, Seuntjens J, Patrocinio H, Podgorsak E. Physical aspects of dynamic stereotactic radiosurgery with very small photon beams (1.5 and 3 mm in diameter). Med Phys 2003;30:111–8.
- Deng J, Guerrero T, Ma C, Nath R. Modelling 6 MV photon beams of a stereotactic radiosurgery system for Monte Carlo treatment planning. Phys Med Biol 2004;49: 1689–704.
- Tsougos I, Theodorou K, Bazioglou M, Stathakis S, Kappas C. A comparison of Monte Carlo simulation with expeirmental dosimetric techniques for a 6 MV stereotactic radiotherapy unit. J Buon 2004;9:451–564.
- Jin L, Wang L, Li J, Luo W, Feigenberg S, Ma C. Investigation of optimal beam margins for stereotactic radiotherapy of lung-cancer using Monte Carlo dose calculations. Phys Med Biol 2007;21:3549–61.
- Jones A, Das I, Jones F. A Monte Carlo study of IMRT beamlets in inhomogeneous media. Med Phys 2003;30: 296–300.
- Capote R, Sanchez-Doblado F, Leal A, Lagares J, Arrans R, Hartmann G. An EGSnrc Monte Carlo study of the microionization chamber for reference dosimetry of narrow irregular IMRT beamlets. Med Phys 2004;31:2416–22.
- Jones A, Das I. Comparison of inhomogeneity correction algorithms in small photon fields. Med Phys 2005;32:766–76.
- Mohan R, Chui C, Lidofsky L. Energy and angular distributions of photons from medical linear accelerators. Med Phys 1985;12:592–7.
- Lydon J. Theoretical and experimental validation of treatment planning for narrow MLC defined photon fields. Phys Med Biol 2005;50:2701–14.
- Ding G, Duggan D, Coffey C. Commissioning stereotactic radiosurgery beams using both experimental and theoretical methods. Phys Med Biol 2006;21:2549–66.
- Sterpin E, Tomsej M, De Smedt B, Reynaert N, Vynchier S. Monte Carlo evaluation of the AAA treatment planning algorithm in a heterogeneous multilayer phantom and IMRT clinical treatments for an Elekta SL25 linear accelerator. Med Phys 2007;34:1665–77.
- Sanchez-Doblado F, Hartmann G, Pena J, Rosello J, Russiello G, Gonzalez-Castano D. A new method for output factor determination in MLC shaped narrow beams. Phys Med 2007;23:58–66.
- Cheng C, Cho S, Taylor M, Das I. Determination of zero-field size percent depth doses and tissue maximum ratios for stereotactic radiosurgery and IMRT dosimetry: Comparison between experimental measurements and Monte Carlo simulation. Med Phys 2007;34:3149–57.
- Ding G, Duggan D, Lu B, Hallahan D, Cmelak A, Malcolm A, . Impact of inhomogeneity corrections on dose coverage in the treatment of lung cancer using stereotactic body radiation therapy. Med Phys 2007;34:2985–94.
- Paschialis T, Sandilos P, Tatsis E, Karaiskos P, Antypas C, Chatzigiannis C, . Dosimetric evaluation of a new collimator insert system for stereotactic radiotherapy. Br J Radiol 2007;80:446–51.
- Zhao Y, Mackenzie M, Kirkby C, Fallone B. Monte Carlo calculation of helical tomography dose delivery. Med Phys 2008;35:3491–500.
- Scott A, Nahum A, Fenwick J. Using a Monte Carlo model to predict dosimetric properties of small radiotherapy photon fields. Med Phys 2008;35:4671–84.
- Sham E, Seuntjens J, Devic S, Podgorsak E. Influence of focal spot on characteristics of very small diameter radiosurgical beams. Med Phys 2008;35:3317–30.
- Ding G, Duggan D, Coffey C. A theoretical approach for non-equilibrium radiation dosimetry. Phys Med Biol 2008; 53:3493–9.
- Heydarian M, Asnaashari K, Allahverdi M, Jaffray D. Dosimetric evaluation of a dedicated stereotactic linear accelerator using measurement and Monte Carlo simulation. Med Phys 2008;35:3943–54.
- Scott A, Nahum A, Fenwick J. Monte Carlo modeling of small photon fields: quantifying the impact of focal spot size on source occlusion and output factors, and exploring miniphantom design for small-field measurements. Med Phys 2009;36:3132–44.
- Belec J, Patrocinio H, Verhaegen F. Development of a Monte Carlo model for the Brainlab microMLC. Phys Med Biol 2005;50:787–99.
- Kairn T, Aland T, Fielding A, Franich R, Johnston P, Kakakhel M, . Adapting a generic BEAMnrc model of the BrainLAB m3 micro-multileaf collimator to simulate a local collimation device. Phys Med Biol 2010;55: N451–N463.
- Kairn T, Kenny J, Crowe SF, AL, Franich R, Johnston P, Knight R, . Modelling a complex micro-multileaf collimator using the standard BEAMnrc distribution. Med Phys 2009;37:1761–7.
- Boudou C, Balosso J, Esteve F, Elleaume H. Monte Carlo dosimetry for synchrotron stereotactic radiotherapy of brain tumours. Phys Med Biol 2005;21:4841–51.
- Moskvin V, DesRosiers C, Papiez L, Timmerman R, Randall M, DesRosiers P. Monte Carlo simulation of the Leksell Gamma Knife: I. Source modelling and calculations in homogeneous media. Phys Med Biol 2002;21: 1995–2011.
- Moskvin V, Timmerman R, DesRosiers C, Randall M, DesRosiers P, Dittmer P, . Monte Carlo simulation of the Leksell Gamma Knife: II. Effects of heterogeneous versus homogeneous media for stereotactic radiosurgery. Phys Med Biol 2004;49:4879–95.
- Lax I, Panettieri V, Wennberg B, Amor Duch M, Naslund I, Baumann P, . Dose distributions in SBRT of lung tumors: Comparison between two different treatment planning algorithms and Monte-Carlo simulation including breathing motions. Acta Oncol 2006;45:978–88.
- Panettieri V, Wennberg B, Gagliardi G, Duch MG, M, Lax I. SBRT of lung tumours: Monte Carlo simulation with PENELOPE of dose distributions including respiratory motion and comparison with different treatment planning systems. Phys Med Biol 2007;52:4265–81.
- Freud N, Letang J, Mary C, Boudou C, Ferrero C, Elleaume H, . A hybrid approach for fast simulation of dose deposition in stereotactic synchrotron radiotherapy. IEEE Trans Nucl Sci 2008:1008–17.
- Freud N, Letang J, Mary C, Boudou C, Ferrero C, Elleaume H, . Fast dose calculation for stereotactic sychrotron radiotherapy. IEEE Eng Med Biol Soc 2007: 3914–7.
- Chetty I, Curran B, Cygler J, DeMarco J, Ezzell G, Faddegon B, . Report of the AAPM Task Group No. 105: Issues associated with clinical implementatino of Monte Carlo-based photon and electron external beam treatment planning. Med Phys 2007;34:4818–53.
- Rogers D, Mohan R, Questions for comparison of clinical Monte Carlo codes. Proc XIII Conf Computers in Radiotherapy. Heidelberg: 2000.
- Neuenschwander H, Born E. A macro Monte Carlo method for electron-beam calculations. Phys Med Biol 1992;37: 107–25.
- Neuenschwander H, Mackie T, Reckwerdt P. MMC – A high-performance Monte Carlo code for electron beam treatment planning. Nucl Instr Meth Phys Res B 1995;40: 543–74.
- Cox L, Schach von Wittenau A, Bergstrom P, Mohan R, Libby B, Wu Q, . Photon beam desciption in PEREGRINE for Monte Carlo dose calculations. Utah USA: Medical Physics Publishing; 1997.
- Schach von Wittenau A, Bergstrom P, Cox L. Patient- dependent beam-modifier physics in Monte Carlo photon dose calculations. Med Phys 2000;27:935–47.
- Ma C, Li J, Pawlicki T, Jiang S, Deng J, Lee M, . A Monte Carlo dose calculation tool for radiotherapy treatment planning. Phys Med Biol 2002;47:1671–89.
- Li J, Pawlicki T, Deng J, Jiang S, Mok E, Ma C. Validation of a Monte Carlo dose calculation tool for radiotherapy treatment planning. Phys Med Biol 2000;45:2969–85.
- Kawrakow I, Fippel M, Friedrich K. 3D electron dose calculation using a Voxel based Monte Carlo algorithm (VMC). Med Phys 1996;23:445–57.
- Fippel M. Fast Monte Carlo dose calculation for photon beams based on the VMC electron algorithm. Med Phys 1999;26:1466–75.
- Kawrakow I, Fippel M. VMC++, a fast MC algorithm for Radiation Treatment planning. XIII International Conference on the Use of Computers in Radiation Therapy. Berlin, Heidelberg, New York: Springer; 2000.
- Kawrakow I, Bielajew AF. On the representation of electron multiple elastic-scattering distributions for Monte Carlo calculations. Nucl Instr Meth Phys Res B 1998;134:325–36.
- Siebers J, Keall P, Mohan R. Performance benchmarks of the MCV Monte Carlo system. XIII International Conference on the Use of Computers in Radiation THerapy. Heidelberg: 2000.
- DeMarco J, Solberg T, Smathers J. A CT-based Monte Carlo simulation tool for dosimetry planning and analysis. Med Phys 1997;25:1–11.
- Jang S, Liu HH, Mohan R. Underestimation of low-dose radiation in treatment planning of intensity-modulated radiotherapy. Int J Radiat Oncol Biol Phys 2008;71:1537–46.
- Xu X, Bednarz, Baarli J, Paganatti H. A review of dosimetry studies on external-beam radiation treatment with respect to cancer induction. Phys Med Biol 2008;53:R193–R241.
- Ioffe V, Hudes R, Shepard S, Simard J, Chin L, Yu C. Fetal and ovarian radiation dose in patients undergoing gamma knife surgery. Surg Neurol 2002;58:32–41.
- Maarouf M, Treuer H, Kocher M, Voges J, Gierich A, Sturm V. Radiation exposure of extracranial organs at risk during stereotactic linac radiosurgery. Strahlenther Onkol 2005;181:463–7.
- Hasanzdeh H, Sharafi A, Verdi M, Nikoofar A. Assessment of absorbed dose to thyroid, parotid and ovaries in patients undergoing gamma knife radiosurgery. Phys Med Biol 2006;51:4375–83.
- Petti P, Chaung C, Smith V, Larson D. Peripheral doses in CyberKnife radiosurgery. Med Phys 2006;33:1770–9.
- Chuang C, Larson D, Zytkovicz A, Smith V, Petti P. Peripheral dose measurement for CyberKnife radiosurgery with upgraded linac shielding. Med Phys 2008;35:1494–6.
- Di Betta E, Fariselli, Bergantin A, Locatelli F, Del Vechhio A, Broggi S, . Evaluation of the peripheral dose in stereotactic radiotherapy and radiosurgery treatments. Med Phys 2010;37:3587–94.
- Taylor ML, McDermott LN, Johnston PN, Haynes M, Ackerly T, Kron T, . Stereotactic fields shaped with a micro-multileaf collimator: Systematic characterisation of peripheral dose. Phys Med Biol 2010;55:873–81.
- Taylor ML, Kron T, Franich RD. Assessment of out-of-field doses in radiotherapy of brain lesions in children. Int J Radiat Oncol Biol Phys 2010 (in press).