Abstract
Boron Neutron Capture Therapy for liver malignancies is being investigated at the University of Mainz. One important aim is the set-up of a reliable dosimetry system. Alanine dosimeters have previously been applied for dosimetry of mixed radiation fields in antiproton therapy, and may be suitable for measurements in mixed neutron and gamma fields. Material and methods. Two experiments have been carried out in the thermal column of the TRIGA Mark II reactor at the University of Mainz. Alanine dosimeters have been irradiated in a phantom and in liver tissue. Results. For the interpretation and prediction of the dose for each pellet, beside the results of the measurements, calculations with the Monte Carlo code FLUKA are presented here. For the phantom, as well as for the liver tissue, the measured and calculated dose and flux values are in good agreement. Discussion. Alanine dosimeters, in combination with flux measurements and Monte Carlo calculations with FLUKA, suggest that it is possible to establish a system for monitoring the dose in a mixed neutron and gamma field for BNCT and other applications in radiotherapy.
The number of liver tumours has doubled in the last 30 years, due to an increase in factors causing liver cancer [Citation1]. Apart from primary liver cancer, there are several kinds of cancer in other body regions, which preferentially develop metastases in the liver. About 20% of all primary and secondary malignancies involve the liver [Citation2], but therapy remains still very limited. The greatest success is promised by transplantation but donor organs are rare. In the late stages of the disease, only palliative treatment is possible [Citation3]. From studies elsewhere, it has been found that Boron Neutron Capture Therapy (BNCT) might be a promising alternative to existing therapies for liver malignancies, which consequently is being investigated at the University of Mainz.
BNCT is based on neutron capture of the boron isotope 10B. Due to the high cross section for capture and the following decay of the nucleus into an alpha particle and a lithium ion, it is possible to use 10B in cancer therapy. The produced particles have a small range in tissue because of their high Linear Energy Transfer (LET), which is the amount of energy transferred to material as an ionising particle travels through it. If boron is concentrated selectively in the tumour, damage occurs within the tumour cells only. Optimal treatment requires irradiation of the liver in a homogeneous neutron field and prevention of irradiation to the surrounding organs, which can be achieved through auto-transplantation. The plan at the University Hospital Mainz is to explant and preserve the liver after an infusion of a borono-phenylalanine fructose complex solution (BPA-F), to transport it to the TRIGA Mark II reactor in Mainz [Citation4], to irradiate the organ in the thermal column of the reactor and to bring back and re-implant it afterwards. An analogue treatment has been already performed twice within the TAOrMINA project at the University of Pavia [Citation5].
In radio-oncology, dose planning is mandatory in order to maximise the dose to the tumour and to minimise the dose in the surrounding, tumour-free tissue. The dose planning for the intended treatment in Mainz is challenging. The absorbed dose highly depends on different parameters, such as the morphology of the tissue irradiated, the local boron concentration reached in the different compartments after infusion, and the particle-field used for the irradiation.
Due to the varying radiobiological effects on different morphologies, the Relative Biological Effectiveness (RBE) has been introduced which leads to a biological weighted dose when multiplied with the absorbed dose. RBE values are determined by the research group in Mainz during in vitro and in vivo experiments. Boron concentrations are determined using several analytical techniques adapted to the special demands of the intended treatment.
A feasibility study [Citation6] showed that the thermal column of the TRIGA reactor will be well suited after some reconstruction. As in all reactors, the irradiation field is a mixed neutron-gamma field, produced in nuclear reactions in the reactor core. Furthermore, neutrons generate secondary particles due to nuclear interactions with different materials of the reactor and the organ itself.
All treatment related parameters are brought together for dose planning in Monte Carlo (MC) simulations. To get reliable simulations, validation is mandatory. This validation is carried out using other MC codes and mainly experiments. Most simulations in our group are carried out with MCNP [Citation7], the most common MC code used in BNCT. To confirm the achieved results, especially concerning mixed radiation fields, the first experiments using alanine pellets and the MC code FLUKA [Citation8] have shown to be a reliable option. The alanine dosimeters are suitable for any dose applied during the planned treatment. Two experiments have been carried out at the TRIGA reactor. Alanine pellets have been irradiated in two materials to simulate the situation of an irradiation in an explanted organ; both set-ups have been simulated using MC-modelling. The main purpose of the presented work was the confirmation of the applicability of the combination of FLUKA and alanine pellets for the TRIGA reactor.
Material and methods
Alanine dosimetry
The alanine pellets are manufactured and analysed by the Primary Standard Laboratory at the National Physical Laboratory (NPL) in the UK who have long experience with alanine dosimetry in photon fields and well-established readout protocols [Citation9]. The pellets have a diameter of 5 mm and a thickness of 2.2–2.3 mm, consisting of 90% finely grained crystalline alanine powder and 10% paraffin wax. When alanine is irradiated with ionising radiation, stable radicals are formed. Using an electron spin resonance (ESR) spectrometer, the unpaired electrons of the radicals can be detected. The value of the ESR signal correlates directly to the number of radicals and therefore gives the detector response, which is a dose, calculated using a calibration in terms of dose to water in a 60Co gamma ray radiation field.
This response of each pellet RTRIGA, correlates to the absorbed dose DTRIGA, also in terms of dose to water, by a factor called the relative effectiveness (RE), as shown by Equation 1:
RE accounts for differences in radiation-induced radical yield between a 60Co gamma ray irradiation and an irradiation in the mixed neutron and gamma filed of the TRIGA reactor.
To determine these RE values and to predict the dose for each pellet, the Hansen & Olsen alanine detector response model [Citation10–13] is used, together with FLUKA, a multi-purpose Monte Carlo transport code.
The necessary input for such simulations is the irradiated geometry, i.e. structure and composition of the thermal column together with the irradiated materials. In order to limit calculation time, one refrains from simulating the whole TRIGA reactor but relies on a so-called source plane, which is a virtual plane source within the irradiation geometry providing the necessary input for Monte Carlo simulations, such as source strengths and spectra. In the present case, the source plane matches a geometrical cross section within the thermal column and is based on previous simulations and measurements [Citation6]. For the FLUKA simulations, this plane has been split into two separate sources for neutrons and for gamma rays from the reactor core.
Alanine irradiations
All experiments are carried out in the thermal column of the TRIGA Mark II reactor on the campus of the University of Mainz. The reactor was operated in steady state mode at a maximum power of 100 kWth for 13 minutes. The TRIGA has four beam tubes and a thermal column where the alanine dosimetry and other BNCT experiments take place. The thermal column is constructed of graphite and has an aluminium and boral lining. Due to the moderation by the carbon atoms, neutrons inside the column have a predominantly thermal energy distribution. The column can be opened at the exit-facing side and has rectangular channels of different sizes. For the present experiments, a 20 cm × 20 cm channel in the left upper centre has been used. In front of the channel, a 5 cm thick Bismuth shield has been placed to protect irradiated materials from the gamma quanta from the reactor core. In the irradiated material, neutron fluxes of about 2 × 1010 n cm−2 s−1 have been observed, which have been measured by gold-foil activation.
In the first experiment, two sets of 10 alanine pellets each were irradiated in a polymethyl-methacrylate (PMMA) phantom (see ). The pellets have been positioned on the longitudinal axis of the cuboid-shaped phantom. The phantom has a length of 23.0 cm and a height and width of 10.0 cm. The pellets can be placed in a circular channel along the central axis in the cavities of the cylindrical PMMA components, which completely fill the channel. For the irradiation, the phantom is placed in a polyethylene (PE) box, which fits in the 20 cm × 20 cm channel of the thermal column.
In the second experiment, six alanine pellets have been positioned in the resected lobe of a human liver and one additional pellet has been positioned in the back of the irradiation box outside the organ. The resected specimens were obtained during a clinical trial, described in previous work [Citation14], and was packed in a way common in transplant surgery. The organ was packed in bags together with Histidine-Tryptophan-Ketoglutarate (HTK) preservation solution. Sealed in PE foils, the pellets were sewed in lamellas that were cut along the longitudinal axis of the liver tissue, before packing, as shown in . The bag was placed in the same irradiation box as used earlier for the phantom experiments.
Results
The measured and calculated total doses for each alanine pellet (RTRIGA) are shown in and for the phantom and liver experiment, respectively. Maximum values of about 23 Gy are reached in both cases in the foremost pellet. For the phantom experiment, a logarithmic decrease with the position on the longitudinal axis can be seen in the measured data to a dose of 9.4 Gy. For the liver experiment, no systematic trend can be observed, due to the difficulties in measuring the exact position of the pellets in soft tissue. The lowest value 9.8 Gy has been reached with the pellet in the back at the box with the largest distance to the reactor core. The measured data in is the average dose value of the two pellets treated under the same conditions in the two runs of the phantom experiment. The measured values differ at most by about 0.1 Gy, the uncertainty of the measurement is indicated by the NPL to be about 3% for gamma ray irradiations. The statistical uncertainties of the calculations are about 8%.
Figure 3. Total dose values DAlanine in Gy for the phantom experiment grey diamonds: measured by the NPL; black squares: calculated by FLUKA.
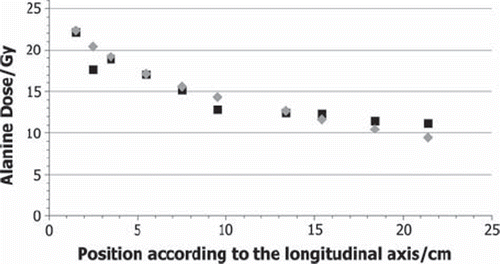
Figure 4. Total dose values DAlanine in Gy for the liver experiment grey diamonds: measured by the NPL; black squares: calculated by FLUKA.
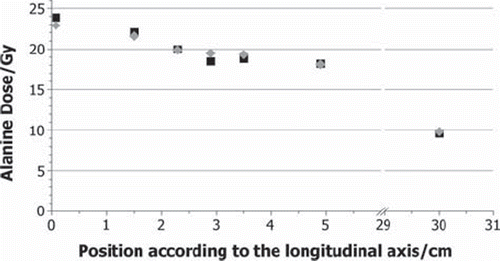
The dose to alanine is the sum of the doses caused by the neutrons and their secondary particles, generated by nuclear reactions, and by gamma rays coming from the reactor core. As a result of the two source planes used this total dose is separated in the calculations into a dose from neutrons and its secondary particles and a dose from the gamma rays from the reactor core. In the calculated total dose and the neutron and gamma dose for the pellets in the phantom experiment is given. shows the same for the liver experiment. It can be seen that the gamma quanta from the reactor generate between 25% and 50% of the total dose. The percentage varies, due to the different behaviour of the gamma ray dose and neutron dose. The neutron dose is continuously decreasing with increasing distance from the core, while the photon dose shows no decrease within an uncertainty of 8% for the calculations.
Figure 5. Calculated segmentation of the Total dose DAlanine (black squares) into the neutron (grey diamonds) and the photon (white triangles) component for the phantom experiment.
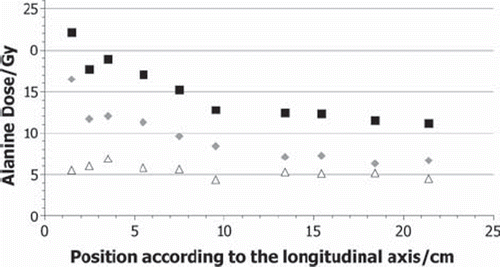
Figure 6. Calculated segmentation of the total dose DAlanine (black squares) into the neutron (grey diamonds) and the photon (white triangles) component for the liver experiment.
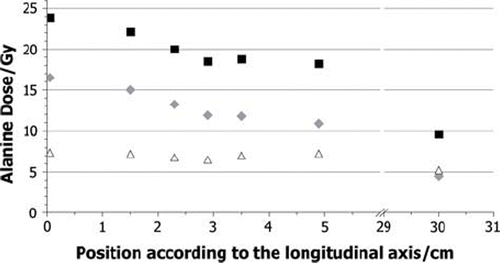
The calculated RE values for each pellet for the neutron dose only and for the total dose of neutrons and gamma quanta from the reactor core are shown in for the phantom experiments and in for the liver experiment. The RE values from the gamma rays from the core only have a constant value of 1 and are not shown in the figures. The RE values for the neutron dose reach values between 0.79 and 0.92, and for total dose between 0.85 and 0.95. Out of the uncertainties of the simulations an error of the RE values of 11% has been calculated.
Discussion
After irradiation, the alanine pellets showed no superficial changes in colour or shape. The readout was performed routinely without any problems at NPL. The reproducibility is very good, since pellets treated under the same conditions lead to the same response within the associated uncertainty.
The calculated dose values fit well with the measured data, as can be seen in and . The differences are less than 10% for all experiments. Several improvements and variations are possible in future calculations to further decrease these differences. The used plane source depends on a feasibility study [Citation6] carried out in 2008. Since then, the arrangement of the fuel elements in the reactor core has been changed. This did not lead to any change of flux but may change the input spectra. Furthermore, the shape and composition of the irradiated materials has been simplified to reduce calculation times. With more detailed build-up geometries, a better agreement can be reached in future.
The dose measured by the ESR spectrometer is, as already mentioned, in terms of dose to water, while the calculated dose is in terms of dose to alanine. The difference is in the stopping power of the materials for the particles, which propagate through it. The charged particle spectrum is dominated by protons (energy about 1 MeV), and the differences in stopping powers for the two media are in the 3% regime. This is well below the general uncertainty of the calculations, which is why this effect was not taken into account.
As indicated in and , the two calculated dose components behave differently. Whereas the neutron dose decreases continuously, from about 16 to 5 Gy, because the propagation of neutrons is not disturbed, the parts of the gamma quanta coming from the reactor are absorbed by the bismuth shielding in the channel. As a result of this shielding the attenuation length of the photon flux is large, so that the flux is almost constant for the liver and the phantom and leads to a dose around 6 Gy. The gamma dose in the liver is slightly higher because the liver is simulated with heavier elements like sodium, sulphur, or phosphorus in small concentrations, while the phantom only consists of the light elements carbon, hydrogen, and oxygen. The observed particle spectra in the alanine pellets suggest that the neutron dose is mainly generated through secondary particles of neutron-hydrogen interactions, like recoiling protons of the 1H(n,n′)1H reaction or 2.22 MeV gamma quanta of the 1H(n,γ)2D reaction. Because both materials irradiated contain comparable hydrogen densities the doses are similar. The biggest difference to the measured data can be seen at the second data point at the 2.5 cm position in . As indicated by , the neutron dose seems to be underestimated, while the photon dose shows a regular value. In general, most differences have their origin in fluctuations of the calculated points from the expected progress, which seems to be a statistical problem.
The calculated RE values, presented in and , seem to be affected by the pellet position or, even more likely, by the surrounding material. The amount and type of the secondary particles, which contribute to the neutron dose, are depending on the elemental composition and the density of the irradiated material. Protons with the observed energy have a very limited range, as a result no significant amount of protons with origin outside the pellet contribute to the dose in it. In contrast to gamma quants, which have a much longer range, so that they can reach the pellet when generated outside of it. This consideration fits with the observed data. The more material is around the pellet, the more gamma quants are generated with an RE of 1. The more they contribute to the dose, the higher the RE value is. As already explained, the total RE value varies due to different behaviour of neutrons and gamma quanta in the sample position. The higher the contribution of the gamma dose compared to the neutron dose generated by secondary particles, the higher the total RE value. Therefore, it is not possible to assume one RE value for all doses observed in the thermal column. Instead it is necessary to consider the surrounding material and its composition for each pellet. In the calculations, a trend can be observed in the RE values, especially in the phantom data. The values reach a maximum in the middle of either the phantom or the liver, where the alanine pellets are surrounded by the largest amount of material. After that, a decrease of the values can be seen again. This trend correlates to the considerations made above. Again statistical fluctuations can be seen and an outlier at 13 cm in .
The calculations presented here provide initial information about the composition of the dose and show that it is possible to use alanine dosimeters in combination with FLUKA for the TRIGA Mainz. Further calculations will have to identify the four dose components traditionally used in BNCT [Citation15]. These are the gamma dose, which includes not only the gamma quanta from the reactor, as shown here, but also the gammas generated in (n,γ) reactions, as for hydrogen. Furthermore, the BNCT dose is subdivided into a fast neutron dose, caused by the recoiling protons from neutron-hydrogen collisions and a nitrogen dose, which is caused by protons produced in the nuclear reaction of nitrogen (14N(n,p)14C) with neutrons. The fourth and most important dose component in BNCT, i.e. the boron dose, is not relevant to the alanine pellets, since the alanine pellets contain no boron. The future aim will be the identification of these dose components. Therefore additional calculations and experiments with different phantom materials will be carried out. The final aim is to establish a system for dosimetry monitoring in a mixed neutron and gamma quantum field for BNCT, which could also be used in other applications in radiotherapy.
Acknowledgements
The authors acknowledge support in part by the National Science Foundation under grant CBET-0853157, from the European Union in the form of a Marie Curie International Incoming Fellowship grant # PIIF-GA-2009-234814, by the Danish Cancer Society (www.cancer.dk), the Lundbeck Foundation Centre for Interventional Research in Radiation Oncology (www.cirro.dk), and by the Boehringer Ingelheim Foundation.
Declaration of interest: There are no conflicts of interest to be declared.
References
- Schurr R. Zunahme des hepatozellulären und des intrahepatischen cholangiozellulären Karzinoms im Nordosten Deutschlands. Deutsche MedizinischeWochenschrift 2006;13: 1649–55.
- Jaeck D, Pessaux P. Bilobar colorectal liver metastases: Treatment options. Surg Oncol Clin N Am 2008;17:553–68.
- Jeßke L, Hübner G, Lotterer E, Fleig WE. Maligne Lebertumoren – Früherkennung und lokale Behandlung. Chirurgische Gastroenterologie 2002;18:128–33.
- Eberhardt K, Kronenberg A. The research reactor TRIGA Mainz: A neutron source for versatile applications in research and education. Kerntechnik 2000;69:269–74.
- Zonta A, Prati U, Roveda L, Ferrari C, Zonta S, Clerici AM, . Clinical lessons from the first applications of BNCT on unresectable liver metastases. J Phys: Conference Series 2006;41:484–95.
- Wortmann B. Auslegung und Optimierung einer Bestrahlungseinrichtung für die Bor Neutronen Einfang Therapie an autotransplantierten Organen [PhD-thesis]. Dresden, Germany: Fakultät Maschinenwesen, TU Dresden; 2008.
- X-5 Monte Carlo Team. MCNP: A general Monte Carlo N-Particle transport code. Version 5, LA-UR-03-1987. Los Alamos: Los Alamos National Laboratory; 2003.
- Battistoni G, Muraro S, Sala PR, Cerutti F, Ferrari A, Roesler S, . The FLUKA code: Description and benchmarking. Proceedings of the Hadronic Shower Simulation Workshop 2006. AIP Conf Proc 2007;896:31–49.
- Sharpe P, Sephtan J. An automated system for the measurement of alanine/EPR dosimeters. App Radiat Iso 2000;52: 1185–8.
- Hansen JW, Olsen KJ. Theoretical and experimental radiation effectiveness of the free radical dosimeter alanine to irradiation with heavy charged particles. Radiat Res 1985; 104:15–27.
- Hansen JW, Olsen KJ, Wille M. The alanine radiation detector for high and low LET dosimetry. Radiat Prot Dosimetry 1987;19:43–7.
- Hansen JW, Waligorski MPR, Byrski E. Intercomparison of gamma ray X-ray and fast neutron dosimetry using alanine detectors. Radiat Prot Dosimetry 1989;27:85–92.
- Herrmann R, Jäkel O, Palmans H, Sharpe P, Bassler N. Dose response of alanine detectors irradiated with carbon ion beams. Med Phys 2011;38:1859–66.
- Schmitz T, Blaickner M, Schütz C, Wiehl N, Kratz JV, Bassler N, . Dose calculation in biological samples in a mixed neutron-gamma field at the TRIGA reactor of the University of Mainz. Acta Oncol 2010;49:1165–9.
- International Atomic Energy Agency (IAEA). Current status of neutron capture therapy (IAEA-TECDOC-1223). Vienna: IAEA; 2001.