Abstract
Background. Radiation-induced lung damage (RILD) is an important problem. Although physical parameters such as the mean lung dose are used in clinical practice, they are not suited for individualised radiotherapy. Objective, quantitative measurements of RILD on a continuous instead of on an ordinal, semi-quantitative, semi-subjective scale, are needed. Methods. Hounsfield unit (HU) changes before versus three months post-radiotherapy were correlated per voxel with the radiotherapy dose in 95 lung cancer patients. Deformable registration was used to register pre- and post-CT scans and the density increase was quantified for various dose bins. The dose-response curve for increased HU was quantified using the slope of a linear regression (HU/Gy). The end-point for the toxicity analysis was dyspnoea ≥ grade 2. Results. Radiation dose was linearly correlated with the change in HU (mean R2 = 0.74 ± 0.28). No differences in HU/Gy between groups treated with stereotactic radiotherapy, conventional radiotherapy alone, sequential or concurrent chemo- radiotherapy were observed. In the whole patient group, 33/95 (34.7%) had dyspnoea ≥ G2. Of the 48 patients with a HU/Gy below the median, 16 (33.3%) developed dyspnoea ≥ G2, while in the 47 patients with a HU/Gy above the median, 17 (36.1%) had dyspnoea ≥ G2 (not significant). Individual patients showed a nearly 21-fold difference in radiosensitivity, with HU/Gy ranging from 0 to 10 HU/Gy. Conclusions. HU changes identify objectively the whole range of individual radiosensitivity on a continuous, quantitative scale. CT density changes may allow more robust and accurate radiogenomics studies.
Side effects of radiotherapy are important: they hamper radiation dose escalation or optimal combination of radiotherapy with systemic treatment, may be detrimental for patients’ wellbeing and can be a source of increased treatment costs. Currently used radiotherapy dose, fractionation and volume schedules are in general based on physical constraints aiming to limit severe side effects to 5–10% of the patient population. However, this also means that about 90% of patients could receive a higher radiotherapy dose without undue side effects, which may in turn lead to higher local tumour control rates. Although the physical models are of great value in current clinical practice and have allowed for a more objective and individualised approach, they have several shortcomings.
In radiotherapy of lung cancer, radiation-induced lung damage (RILD) is one of the most important dose limiting side effects. The probability for RILD is typically inferred from the mean lung dose (MLD) or the V20 (percentage of the lung volume receiving more than 20 Gy); however, the area under the curve (AUC) of the receiver operator curve (ROC) for development of RILD is typically only 0.60–0.65, which leaves much room for improvement [Citation1,Citation2]. The addition of dosimetric parameters for other organs such as the heart may improve the prediction of RILD; however, still discarding huge differences between individuals in their radiosensitivity [Citation3,Citation4]. The latter observation has fuelled research to correlate genetic features with radiation-induced side effects; a field termed “radiogenomics”. Although correlations between some single nucleotide polymorphisms (SNP) and RILD have been reported, reproducibility remains problematic, as it is in radiogenomics in general [Citation5–15].
The inability to find correlations between genetic features and RILD may be caused by the inaccuracy with which this side effect is scored. First, all scoring systems are semi-quantitative at best and have ordinal scales that differ between research groups [Citation16,Citation17]. The consequence is that the continuous nature of lung damage is discarded and that the resolution of the system is low. Some scoring systems include the use of medication (steroids), which may be partially related to the physician preferences. The use of medication is a binary endpoint as is the grading of dyspnoea according to a certain threshold, e.g. dyspnoea grade 2 or more. It is thus not surprising that the detection resolution is low. Equally important is the difficulty to know the causal relationship between dyspnoea or the use of steroids and the administration of radiotherapy [Citation18]. Uncertainty was observed in about 25–50% of patients, i.e. in up to half of the individuals’ dyspnoea or the use of steroids was scored as RILD and attributed to radiotherapy, while it may have been due to other medical conditions such as heart disease, exacerbation of chronic obstructive lung disease (COPD) or infections.
An objective system that allows defining radiation injury of the lung on a continuous scale would therefore be highly welcomed. Lung density changes during and after radiotherapy measured on CT scans have been reported to be a robust way to measure radiation-induced changes after stereotactic radiotherapy [Citation19,Citation20]. In this study, we use CT density changes after radiotherapy to investigate more specifically lung damage induced by radiotherapy.
Methods and patients
Patients
CT scans of lung cancer patients treated with radiotherapy with curative intent were retrospectively retrieved from the prospective database of the University Hospitals Leuven and Maastro clinic. All patients had a planning CT scan prior to radiotherapy and an a priori scheduled CT scan three months after radiotherapy. With the latter inclusion criterion, individuals that would have been entered that underwent a CT of the chest that was specifically requested because of symptoms were avoided. Patients were treated with radiotherapy alone, sequential chemotherapy and radiotherapy or concurrent chemo- radiotherapy.
For non-small cell lung cancer (NSCLC), in case of induction chemotherapy, three cycles of cisplatin and gemcitabine were given, with at least three weeks between the last infusion of gemcitabine and the first day of radiotherapy. Patients were scheduled to receive up to 70 Gy to the planning target volume (PTV). Concurrent chemotherapy consisted of two cycles of either cisplatin and vinorelbine or cisplatin and etoposide given during radiotherapy tot a dose up to 69 Gy. Patients treated with stereotactic radiotherapy (SBRT) had coin lesions and received three fractions of 18 Gy. Small cell lung cancer (SCLC) patients were treated with concurrent cisplatin and etoposide and 45 Gy (1.5 Gy twice-daily). Planning and execution of radiotherapy was according to European Organisation for Research and Treatment of Cancer (EORTC) guidelines in both institutions [Citation21].
Dyspnoea was scored with the CTCAE 4.0 (common toxicity criteria for adverse events) instrument before and during therapy and every three months thereafter. In this study, the maximal dyspnoea immediately after the end of radiotherapy to four months after radiation was used as an endpoint.
A total of 95 patients were included, 71 with NSCLC and 24 with SCLC. Radiotherapy was given as a single treatment modality in 19 patients, including 14 treated with SBRT. Sequential chemotherapy and radiotherapy was given in 30 NSCLC patients and concurrent chemo-radiotherapy in 46 (24 SCLC and 22 NSCLC). There were 71 males and 24 females, with a mean age of 66.3 ± 8.4 years (range 43–83). The pre-treatment FeV1 (forced expiratory volume at 1 second) was 2.0 ± 0.8 litres (range 0.8–3.77). The smoking status was: current smoker 32 patients (33.6%), former smoker (quitted smoking more than one year before treatment) 54 patients (56.8%), never smoker two patients (2.3%), smoking status unknown seven patients (7.3%). The mean MLD was 13.6 ± 4.3 Gy (range 3.6–22.0).
Imaging
Radiation treatment planning four-dimensional (4D)-CT scans were used as baseline scans, with all breathing cycles available. Follow-up scans were acquired in the inspirational breath-hold on a diagnostic CT scanner with a curved table top. To compensate for different positioning and breathing state, deformable registration between the baseline and follow-up scans was used. In Maastro clinic, this was done with an in-house developed non-rigid registration package (Mathworks, Natick, MA, USA) validated for image registration inside the thorax [Citation22]. In Leuven, MIM-VISTA (MIM Software Inc., Cleveland, OH, USA) was used [Citation20]. Delineations of the lung and the PTV, together with the 3D dose distribution (AAA calculation algorithm, or superposition algorithm) were exported from the treatment planning system (Eclipse, Varian Medical Systems, Inc., Palo Alto, CA, USA and XiO, Elekta, Stockholm, Sweden). As at the boundaries between the lungs and the ribs slight erroneous registrations may lead to huge differences in HU, lung contours were eroded by 5 mm.
The dose distribution within the eroded lung contours and excluding the PTV was binned into dose bins. A dose response curve (DRC) was generated for each patient, showing the average HU change as a function of dose per bin. The DRCs of all patients for each treatment group were combined to create population DRCs. The population curve was created from the weighted contribution of each patient based on the relative lung volume at that dose.
The dose in all dose bins in all patients was re-calculated to equivalent dose in 2 Gy fractions with an α/β value of 3 Gy.
Variations in HU may be due to technical reasons, breathing [Citation23] and changes over time in the same individual [Citation24]. This may introduce a variation of about 15 HU. Intravenous contrast increases HU by 15–20 HU [Citation25], which is considered to be an important enhancement. In order to take into account variability and to be sure to deal with a significant increase of HU, we arbitrary decided that patients with an increase of more than 0.5 HU per Gy dose in each voxel of lungs (i.e. 35 HU increase for a radiotherapy dose of 70 Gy) were considered as having a significant increase.
Statistical analysis
Results are expressed as mean ± standard deviation (SD) or 95% confidence intervals (CI). The range is given between brackets. Linear regression was used to correlate HU change over time with radiation dose. Differences between groups were calculated with Wilcoxon's signed rank tests and correlations with a Spearman's statistic. Proportions were compared with a χ2-test. P-values less than 0.05 were considered to be significant.
Results
Dyspnoea after radiotherapy
Dyspnoea grade 2 or more occurred in 33/95 (34.7%) of patients after radiotherapy and was significantly higher with increasing MLD, but only with a weak correlation (R = 0.08; p = 0.027). Dyspnoea ≥G2 incidence was 9/34 (26.5%) in the group with a MLD < 10 Gy, 12/27 (44%) when the MLD was 10–14.9 Gy and 11/34 (32%) with MLD-values between 15.0–22.0 Gy. The distribution of MLD is given in .
HU changes and correlation with dyspnoea
The mean HU/Gy in patients from Maastricht was 1.8 ± 2.1; those from Leuven 1.4 ± 1.3 (none of the differences were significant).
HU change (ΔHU) at baseline compared to three months post-treatment was linearly correlated with the radiotherapy dose: mean R2 = 0.74 ± 0.28 (0.24–0.99), p = 0.07 (Supplementary Figure 1, available online at http://informahealthcare.com/doi/abs/10.3109/0284186X.2013.813074).
The distribution of ΔHU per Gy is depicted in Supplementary Figure 2 (available online at http://informahealthcare.com/doi/abs/10.3109/0284186X.2013.813074). The HU increase per Gy for each treatment group were: for SBRT: 1.4 ± 1.3 for the α/β corrected values and 2.1 ± 1.9 for the non-α/β corrected slope, for non-SBRT radiotherapy alone: 1.7 ± 1.7; for sequential chemo-radiotherapy: 1.5 ± 1.6; and for concurrent chemo-radiation: 2.1 ± 2.8 (all differences not significant, p-values > 0.35). We therefore pooled all treatment groups. For the whole group, the mean ΔHU/Gy was 1.7 ± 2.0 (range −1.6–10.7), the median ΔHU/Gy was 1.1.
A striking heterogeneity between patients in ΔHU /Gy was observed (). Of the 48 patients with a ΔHU/Gy below the median, 16 (33.3%) developed dyspnoea ≥ G2, while in the 47 patients with a ΔHU/Gy above the median, 17 (36.1%) had dyspnoea ≥ G2 (p = 0.77).
Figure 2. Changes in Hounsfield units (HU) per Gy (y-axis) for each individual patient (x-axis) (n = 95).
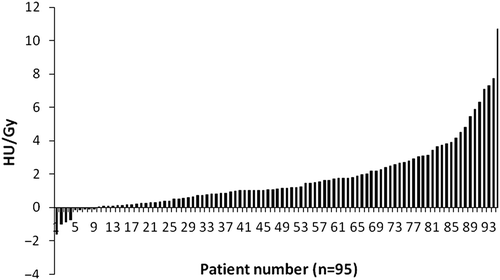
As the radiation response of the lungs has an important volume dependency, volume effects were investigated. Only a small volume effect on the ΔHU /Gy was observed (Supplementary Figure 3, available online at http://informahealthcare.com/doi/abs/10.3109/0284186X.2013.813074). With a change of 0.5 HU/Gy considered to be of importance, 26 patients had no significant HU changes, whereas 69 had. Of the 26 patients without significant changes, eight patients (31%) had dyspnoea ≥ G2. In the 69 individuals with HU change, 25 patients (36%) had dyspnoea ≥ G2 (p < 0.001).
HU changes identify more appropriately individuals with a radiosensitive phenotype than dyspnoea
In the whole patient group, 33/95 (34.7%) had dyspnoea ≥ G2, whereas 69/95 (72.6%) had a significant change in HU.
Assuming that a change in HU of more than 0.5/Gy is relevant (see methods section), the 69 patients with HU changes had a range between 0.51 HU/Gy and 10.7 HU/Gy. Twenty-five of these 69 patients had dyspnoea ≥ G2.
Discussion
RILD is of obvious relevance for it is an important dose-limiting toxicity and it impairs patients’ wellbeing and quality of life. Dose-volume parameters such as the MLD or V20 are used in clinical practice as safe thresholds [Citation21]. As these systems ignore the large individual variability in radiation susceptibility, much research has been reported on genetic correlations with RILD [Citation5–10]. Examples include SNPs for TGF-β, heat-shock proteins, ATM polymorphisms, polymorphisms for base excision repair genes and non-homologous end joining gene and inflammation-related genes. However, reproducibility of these results appear difficult [Citation14,Citation15]. An important reason is that the endpoints for RILD vary between studies and investigator groups and are typically scored on a partly subjective, ordinal scale [Citation16,Citation17]. Moreover, dyspnoea or steroid use after radiotherapy, both typically used as surrogate measures of RILD, may be due to other causes than RILD in up to 50% of patients [Citation18]. There is therefore a genuine need to develop more specific tools to determine objectively RILD on a continuous scale that allows the broad spectrum of individual radiosensitivity to be taken into account.
Changes in lung density due to radiotherapy have been proposed as a way to quantify RILD [Citation19,Citation20,Citation25–28]. In the present study, we show the huge range of individual sensitivity for radiotherapy. Differences up to 20-fold in ΔHU/Gy were observed. No relation was observed between HU changes and important dyspnoea: in patients with a HU/Gy below the median, 33.3% developed dyspnoea ≥ G2, while in patients with a HU/Gy above the median 36.1% had shortness of breath. Over 70% of patients showed significant increases of HU units, thus showing radiation susceptibility.
The use of CT density changes may thus allow a more robust correlation with radiogenomics features than shortness of breath for it identifies double as much radiosensitive individuals than dyspnoea and take into account large quantitative differences in sensitivity. This study is presently ongoing. Furthermore, it is an objective measure that can be used to compare results across studies.
The present study has some obvious shortcomings. First, the patients received different types of treatment and are inhomogeneous regarding their smoking status and gender. However, no significant differences between the ΔHU/Gy values were observed. Second, the CT scans in the follow-up were acquired in another position and on other machines than those taken for radiation planning purposes. This may have introduced some inaccuracies. Third, the elastic deformation algorithms were not the same in the two institutions. The dose-HU slopes nevertheless turned out to be similar. Lung density changes between the mid-ventilation and the maximal inspiration or expiration position are on average 18 HU, which would not change our conclusions. Registration errors range between 0.5 mm and 4.0 mm, which also would not lead to different conclusions. Fourth, the number of patients is reasonable, but not large enough to use them as an external validation set. Lastly, no correlations with genetic features were made.
In our opinion, this study provides data that support the use of CT density changes in future radiogenomics research.
Supplementary Figures 1–3
Download PDF (783.5 KB)Declaration of interest: The authors report no conflicts of interest. The authors alone are responsible for the content and writing of the paper.
References
- Dehing-Oberije C, De Ruysscher D, van Baardwijk A, Yu S, Rao B, Lambin P. The importance of patient characteristics for the prediction of radiation-induced lung toxicity. Radiother Oncol 2009;91:421–6.
- Vogelius IR, Bentzen SM. A literature-based meta-analysis of clinical risk factors for development of radiation induced pneumonitis. Acta Oncol 2012;51:975–83.
- Huang EX, Hope AJ, Lindsay PE, Trovo M, El Naqa I, Deasy JO, et al. Heart irradiation as a risk factor for radiation pneumonitis. Acta Oncol 2011;50:51–60.
- Ghobadi G, van der Veen S, Bartelds B, de Boer RA, Dickinson MG, de Jong JR, et al. Physiological interaction of heart and lung in thoracic irradiation. Int J Radiat Oncol Biol Phys 2012;84:e639–46.
- Pang Q, Wei Q, Xu T, Yuan X, Lopez Guerra JL, Levy LB, et al. Functional promoter variant rs2868371 of HSPB1 is associated with risk of radiation pneumonitis after chemoradiation for non-small cell lung cancer. Int J Radiat Oncol Biol Phys 2013;85:1332–9.
- Xiong H, Liao Z, Liu Z, Xu T, Wang Q, Liu H, et al. ATM polymorphisms predict severe radiation pneumonitis in patients with non-small cell lung cancer treated with definitive radiation therapy. Int J Radiat Oncol Biol Phys 2013;85: 1066–73.
- Yin M, Liao Z, Liu Z, Wang LE, O’Reilly M, Gomez D, et al. Genetic variants of the nonhomologous end joining gene LIG4 and severe radiation pneumonitis in non-small cell lung cancer patients treated with definitive radiotherapy. Cancer 2012;118:528–35.
- Yin M, Liao Z, Liu Z, Wang LE, Gomez D, Komaki R, et al. Functional polymorphisms of base excision repair genes XRCC1 and APEX1 predict risk of radiation pneumonitis in patients with non-small cell lung cancer treated with definitive radiation therapy. Int J Radiat Oncol Biol Phys 2011;81:e67–73.
- Hildebrandt MA, Komaki R, Liao Z, Gu J, Chang JY, Ye Y, et al. Genetic variants in inflammation-related genes are associated with radiation-induced toxicity following treatment for non-small cell lung cancer. PLoS One 2010; 5:e12402.
- Yuan X, Liao Z, Liu Z, Wang LE, Tucker SL, Mao L, et al. Single nucleotide polymorphism at rs1982073:T869C of the TGFbeta 1 gene is associated with the risk of radiation pneumonitis in patients with non-small-cell lung cancer treated with definitive radiotherapy. J Clin Oncol 2009;27: 3370–8.
- Barnett GC, Elliott RM, Alsner J, Andreassen CN, Abdelhay O, Burnet NG, et al. Individual patient data meta-analysis shows no association between the SNP rs1800469 in TGFB and late radiotherapy toxicity. Radiother Oncol 2012;105:289–95.
- Talbot CJ, Tanteles GA, Barnett GC, Burnet NG, Chang-Claude J, Coles CE, et al. A replicated association between polymorphisms near TNFα and risk for adverse reactions to radiotherapy. Br J Cancer 2012;107:748–53.
- Barnett GC, Coles CE, Elliott RM, Baynes C, Luccarini C, Conroy D, et al. Independent validation of genes and polymorphisms reported to be associated with radiation toxicity: A prospective analysis study. Lancet Oncol 2012;13: 65–77.
- West CM, Barnett GC. Genetics and genomics of radiotherapy toxicity: Towards prediction. Genome Med 2011;3:52.
- Voets AM, Oberije C, Struijk RB, Reymen B, De Ruyck K, Thierens H, et al. No association between TGF-β1 polymorphisms and radiation-induced lung toxicity in a European cohort of lung cancer patients. Radiother Oncol 2012;105:296–8.
- Lopez Guerra JL, Gomez D, Zhuang Y, Levy LB, Eapen G, Liu H, et al. Change in diffusing capacity after radiation as an objective measure for grading radiation pneumonitis in patients treated for non-small-cell lung cancer. Int J Radiat Oncol Biol Phys 2012;83:1573–9.
- Tucker SL, Jin H, Wei X, Wang S, Martel MK, Komaki R, et al. Impact of toxicity grade and scoring system on the relationship between mean lung dose and risk of radiation pneumonitis in a large cohort of patients with non-small cell lung cancer. Int J Radiat Oncol Biol Phys 2010;77: 691–8.
- Yirmibesoglu E, Higginson DS, Fayda M, Rivera MP, Halle J, Rosenman J, et al. Challenges scoring radiation pneumonitis in patients irradiated for lung cancer. Lung Cancer 2012; 76:350–3.
- Ghobadi G, Hogeweg LE, Faber H, Tukker WG, Schippers JM, Brandenburg S, et al. Quantifying local radiation-induced lung damage from computed tomography. Int J Radiat Oncol Biol Phys 2010;76:548–56.
- Diot Q, Kavanagh B, Schefter T, Gaspar L, Stuhr K, Miften M. Regional normal lung tissue density changes in patients treated with stereotactic body radiation therapy for lung tumors. Int J Radiat Oncol Biol Phys 2012;84: 1024–30.
- De Ruysscher D, Faivre-Finn C, Nestle U, Hurkmans CW, Le Péchoux C, Price A, et al. European Organisation for Research and Treatment of Cancer recommendations for planning and delivery of high-dose, high-precision radiotherapy for lung cancer. J Clin Oncol 2010;28: 5301–10.
- Janssens G, Jacques L, Orban de Xivry J, Geets X, Macq B. Diffeomorphic registration of images with variable contrast enhancement. Int J Biomed Imaging 2011;2011: 891585.
- Sarrut D, Boldea V, Miguet S, Ginestet C. Simulation of four-dimensional CT images from deformable registration between inhale and exhale breath-hold CT scans. Med Phys 2006;33:605–17.
- Bakker ME, Stolk J, Putter H, Shaker SB, Parr DG, Piitulainen E, et al. Variability in densitometric assessment of pulmonary emphysema with computed tomography. Invest Radiol 2005;40:777–83.
- Palma DA, van Sörnsen de Koste JR, Verbakel WF, Senan S. A new approach to quantifying lung damage after stereotactic body radiation therapy. Acta Oncol 2011;50:509–17.
- Partridge M, Yamamoto T, Grau C, Høyer M, Muren LP. Imaging of normal lung, liver and parotid gland function for radiotherapy. Acta Oncol 2010;49:997–1011.
- Nyeng TB, Kallehauge JF, Høyer M, Petersen JB, Poulsen PR, Muren LP. Clinical validation of a 4D-CT based method for lung ventilation measurement in phantoms and patients. Acta Oncol 2011;50:897–907.
- Mattonen SA, Palma DA, Haasbeek CJ, Senan S, Ward AD. Distinguishing radiation fibrosis from tumour recurrence after stereotactic ablative radiotherapy (SABR) for lung cancer: A quantitative analysis of CT density changes. Acta Oncol 2013;52:910–8.