Abstract
Clinically, wounds on the face tend to heal with less scarring than those on the trunk, but the causes of this difference have not been clarified. Fibroblasts obtained from different parts of the body are known to show different properties. To investigate whether the characteristic properties of facial and trunk wound healing are caused by differences in local fibroblasts, we comparatively analyzed the functional properties of superficial and deep dermal fibroblasts obtained from the facial and trunk skin of seven individuals, with an emphasis on tendency for fibrosis. Proliferation kinetics and mRNA and protein expression of 11 fibrosis-associated factors were investigated. The proliferation kinetics of facial and trunk fibroblasts were identical, but the expression and production levels of profibrotic factors, such as extracellular matrix, transforming growth factor-β1, and connective tissue growth factor mRNA, were lower in facial fibroblasts when compared with trunk fibroblasts, while the expression of antifibrotic factors, such as collagenase, basic fibroblast growth factor, and hepatocyte growth factor, showed no clear trends. The differences in functional properties of facial and trunk dermal fibroblasts were consistent with the clinical tendencies of healing of facial and trunk wounds. Thus, the differences between facial and trunk scarring are at least partly related to the intrinsic nature of the local dermal fibroblasts.
Introduction
Fibroblasts are the most common cells present in connective tissues, where they synthesize extracellular matrix (ECM) and play a critical role in wound healing [Citation1]. Fibroblasts are known to be composed of diverse cell populations and manifest phenotypic differences in their function, such as ECM production and organization, and production of growth factors and cytokines [Citation2–5]. These differences in properties are notable in cutaneous pathological conditions such as keloid [Citation6], hypertrophic scar [Citation7], scleroderma [Citation8], café au lait macule [Citation9], and neurofibroma [Citation10], and even under physiological conditions, fibroblasts exhibit differences; this is known as fibroblast heterogeneity [Citation3–5]. The functional differences are particularly evident between superficial dermal fibroblasts and deep dermal fibroblasts [Citation3–5,11–15]. Heterogeneity also exists between anatomical locations, as several recent studies have indicated that fibroblasts from different body sites retain positional information and topographic differentiation patterns in the expression of genes in vitro [Citation16,17]. However, there are limited reports of the differences in wound healing-associated functions between dermal fibroblasts based on body sites [Citation18].
Table 1. Profiles and data description codes of samples
Clinically, wounds on the face and trunk show different tendencies for wound scarring [Citation19]. Facial incisional wounds, particularly preauricular incisional wounds, heal with less scarring than similar wounds on the trunk. Factors such as the innate properties of resident cells, thickness and compositional structure of the dermis, perfusing blood flow, and mechanical stresses such as skin tension are thought to be the reasons for such differences [Citation19,20]. However, to our knowledge, no studies have elucidated the specific mechanisms responsible.
In order to clarify whether the characteristic properties of facial and trunk scarring are due to differences in local fibroblasts, functional differences between facial dermal fibroblasts and trunk dermal fibroblasts were investigated, using primary facial superficial dermal fibroblasts (FS), facial deep dermal fibroblasts (FD), trunk superficial dermal fibroblasts (TS), and trunk deep dermal fibroblasts (TD). Thus, cellular proliferation kinetics, and expression and production of 11 fibrosis-associated factors, including representative ECM metabolism-associated factors or cytokines, which are considered to be related to scar fibrosis, were investigated and compared between facial and trunk dermal fibroblasts.
Materials and methods
Cell Isolation and Culture
Facial (preauricular) and trunk (lateral thoracic) skin was obtained during reconstructive surgery from seven healthy donors without antecedent operative invasion to the biopsied site (four females and three males; age, 46.6 ± 14.4 years). Profiles and data description codes are shown in . The research protocol was approved by the internal review board of our university hospital. Informed consent was obtained from all patients. After resection of subcutaneous tissues, specimens were washed three times in phosphate-buffered saline, and the external and internal surfaces were dermatomed in order to obtain superficial and deep dermal samples, respectively. To avoid cellular selection bias, both samples were incubated with 0.25% trypsin and 0.02% EDTA in phosphate-buffered saline for 16–24 hr at 4°C and the epithelium was separated from the superficial dermal sample. From the separated superficial and deep dermal samples, human fibroblasts were cultured as explants and maintained at 37°C under a 5% CO2 atmosphere in fibroblast growth medium (FGM) consisting of Dulbecco’s modified Eagle’s medium (DMEM) supplemented with 10% fetal calf serum and 0.6 mg/ml glutamine. After about 3 weeks, primary cultures were subcultured. Medium was replaced every 3 days during cell culture.
Proliferation Assay
Cells at the third passage were plated in triplicate in 12-well plates at 1.0 × 104 cells/well in FGM. Medium was replaced every 4 days and the cell number was manually counted at the same time until day 32.
Quantification of mRNA by Real-Time Polymerase Chain Reaction
Cells at four or five passages were plated in 12-well plates at 1.0 × 104 cells/well in FGM. On day 4, total RNA was isolated using an RNeasyTM Mini Kit and QIA shredder (both from QIAGEN, Hilden, Germany), followed by reverse transcription using a High Capacity RNA-to-cDNA kit. Wound maturation-associated genes (listed in ) were quantified using real-time polymerase chain reaction (PCR). Reaction mixtures comprised 10 μl of FAST SYBR® Green Master Mix and 1 μl of cDNA sample and RNase-free water with the indicated primer concentrations. Reactions were performed and monitored using the StepOnePlusTM real-time PCR system. All PCR reagents and the PCR system were obtained from Applied Biosystems (Foster City, CA, USA). PCR comprised 40 cycles, consisting of denaturing at 95°C (3 s) and annealing/extension at 60°C (15 s). Primer sequences were designed based on previous studies and were optimized for concentration [Citation8,21–25]. Primers for which amplification efficiency was between 1.95 and 2.05 were employed for the study. Sequences and optimized concentrations for each primer are shown in . For quantification, expression levels were calculated by the comparative CT method using glyceraldehyde-3-phosphate dehydrogenase (GAPDH) and human acidic ribosomal protein (HARP) as an endogenous reference gene. Preliminary tests confirmed that both the endogenous controls offered similar results. Therefore, we decided to use GAPDH as an internal control throughout the study.
Table 2. Primer sequences and optimized concentrations for real-time PCR
Measurement of ECM and Cytokines by Enzyme-Linked Immunesorbent Assay
Samples for enzyme-linked immunesorbent assay (ELISA) were collected under the same culture conditions as cDNA. Soluble and sediment type I collagens were quantitatively analyzed by ELISA (Human collagen I EIA kit; Applied Cell Biotechnologies, Inc., Yokohama, Kanagawa, Japan) according to the manufacturer’s instructions. Transforming growth factor-β1 (TGF-β1), transforming growth factor-β2 (TGF-β2), and connective tissue growth factor (CTGF) in culture supernatants were also measured by ELISA (QuantikineTM from R&D Systems (Minneapolis, MN, USA) for TGF-β1 and TGF-β2, and Human CTGF ELISA Kit from Cusabio, Inc., Wuhan, Hubei, China, for CTGF), in accordance with the manufacturer’s instructions. Levels of each factor were measured using a microplate reader (Power Scan® HT; Dainippon Pharmaceutical, Osaka, Japan). Data are expressed as secreted factors per 1.0 × 104 cells at the time of harvest.
Statistical Analyses
Differences in values between groups of cells were analyzed by paired Student’s t-test. In the proliferation assay, associations between cell number of facial dermal fibroblasts and trunk dermal fibroblasts at confluence (on day 32) were statistically analyzed using Pearson’s correlation index. Values of p < 0.05 were considered to be statistically significant. Chronological changes in cell number are presented as means ± standard error of the mean, and other data are presented as means ± standard deviation.
Results
Cell Morphology and Proliferation
Morphologically, no differences were observed between dermal fibroblasts obtained from the face and trunk, while superficial dermal fibroblasts and deep dermal fibroblasts showed apparent differences regardless of donor site. Superficial dermal fibroblasts were smaller and spindle-shaped when compared with deep dermal fibroblasts, which tended to broadly spread on the surface (). In addition, with regard to proliferation kinetics, no differences were observed between facial and trunk dermal fibroblasts, although the cellular density of superficial dermal fibroblasts tended to be higher than that of deep dermal fibroblasts on proliferation assay (A), as shown in the comparative description of cell numbers of FS, FD, TS, and TD on day 32 (B). Cell numbers at confluency on day 32 demonstrated that for superficial dermal fibroblasts and deep dermal fibroblasts, respectively, cellular density of facial and trunk dermal fibroblasts from the same donor showed significant correlations (C).
Figure 1. Cell morphology of FS, TS, FD, and TD. Phase contrast microscopic findings for FS, TS, FD, and TD from donor No. 4 at 4, 12, and 32 days after cell seeding. Scale bar indicates 100 μm.
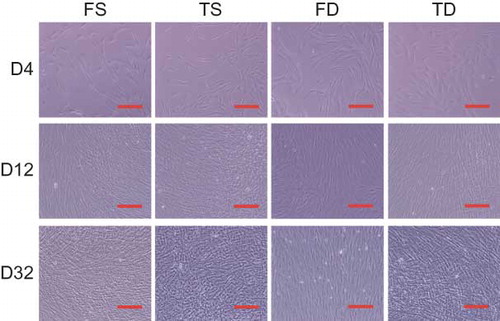
Figure 2. Cell proliferation of FS, TS, FD, and TD. (A) Chronological cell number in four cell fractions is noted (n = 7 for each). Error bars indicate SEM. (B) Cell count of FS, TS, FD, and TD on day 32 (n = 7 for each). (C) Correlation of saturated cell number between facial and trunk fibroblasts on day 32.
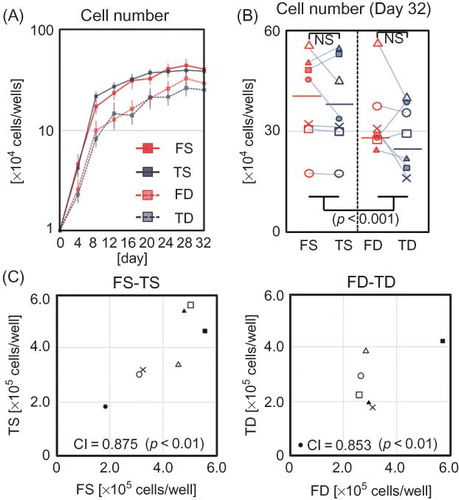
mRNA Expression of Fibrosis-Associated Factors
In order to investigate the functional differences between facial and trunk dermal fibroblasts, mRNA expression of fibrosis-associated factors in superficial dermal fibroblasts and deep dermal fibroblasts was quantitatively compared using real-time PCR.
Among superficial dermal fibroblasts, FS showed lower expression of ECMs such as type I and III collagens, fibronectin, TGF-β1 and TGF-β3, and CTGF when compared with TS. On the other hand, expression of TGF-β2 was higher in FS than in TS. Expression of MMP1, ASMA, bFGF, and HGF showed no clear trends. Among deep dermal fibroblasts, FD showed lower expression of TGF-β1 and CTGF than TD, while no clear trends were seen for other factors ().
Production of Fibrosis-Associated Factors
In order to further confirm the differences between facial and trunk dermal fibroblasts, protein production of type I collagen, TGF-β1, TGF-β2, and CTGF were compared by ELISA.
Among superficial dermal fibroblasts, FS showed significantly lower production of type I collagen, TGF-β1, and CTGF than TS. this was consistent with the results of mRNA expression analysis. In addition, production of TGF-β2 showed the same trend as for mRNA expression, with higher production in FS than that in TS, although the differences were not significant. Among deep dermal fibroblasts, similar to results of mRNA expression analysis, FD showed lower production of TGF-β1 and CTGF than TD, and no clear trends were seen for type I collagen and TGF-β2 ().
Discussion
In our analysis of seven paired FS, FD, TS, and TD samples from the same individuals, facial and trunk dermal fibroblasts obtained from the same layers of dermis showed identical morphology and proliferation kinetics, while differences in depth of origin distinctly affected cell morphology and proliferation kinetics, as indicated in previous reports [Citation11–15]. On the other hand, the cellular density of facial and trunk dermal fibroblasts from the same depth of dermis in the same individuals showed significant, positive correlations. The proliferative capability of superficial and deep dermal fibroblasts is thought not to be influenced by anatomical site, but is specific to donor individuals.
In a subsequent study, mRNA expression of 11 genes considered to be associated with scarring (i.e., fibrosis formation) was investigated between facial and trunk dermal fibroblasts. Type I and III collagens, fibronectin, and collagenase expression were investigated because ECM production causes excess deposition of ECM, and were considered to be a profibrotic factor [Citation8,9,26], while collagenase expression is considered to be an antifibrotic factor [Citation11,27]. Other cytokines, such as TGF-βs, CTGF, bFGF, and HGF, were also investigated because these may be involved in fibrotic processes [Citation11,27–31]. TGF-β1 and its downstream mediator CTGF are known to play particular important roles in hypertrophic scar formation and keloids [Citation6,12,24,30,32] and are therefore considered to be dominant profibrotic factors. TGF-β2 [Citation19,31] and TGF-β3 [Citation19,30,31,33,34] also play important roles in the process of fibrotic scar formation, although their relative dominance during scar development is smaller when compared with TGF-β1 and CTGF, and their effects on scarring were not clearly defined as pro- or antifibrotic. On the other hand, HGF is reported to have antifibrogenic effects in various organs [Citation35,36], and much more recently, bFGF was found to prevent fibrogenesis via activation of HGF secretion from adipose-derived cells and dermal fibroblasts [Citation37]. Therefore, we also investigated the expression of HGF and bFGF as possible antifibrotic factors.
Between facial and trunk dermal fibroblasts, differences in expression and/or production of fibrosis-associated factors have been noted. In particular, it was noted that expression and production of TGF-β1 and CTGF, known as one of the most potent fibrosis-inducing factors [Citation6,12,24,30,32], were lower in facial fibroblasts than in trunk fibroblasts. Moreover, with regard to superficial dermal fibroblasts, facial fibroblasts showed lower expression and/or production of ECMs than trunk fibroblasts. This indicates that facial dermal fibroblasts are intrinsically less fibrotic than trunk dermal fibroblasts, although the reasons for the differences in TGF-β2 and TGF-β3 expressions in fibrosis are unclear.
Our study demonstrated that facial and trunk dermal fibroblasts in the superficial and deep dermis possess identical proliferative capacity, but that facial dermal fibroblasts show lower fibrotic activity in mRNA expression and protein production analyses. We believe that these functional differences in local dermal fibroblasts are at least partly responsible for the clinically observed differences in scarring in the face and trunk. (Facial wounds tend to heal with less scarring than trunk wounds.)
As noted by Chang et al. in a genome-wide mRNA expression analysis using a microarray, fibroblasts obtained from various body sites displayed distinct and characteristic transcriptional patterns, particularly with regard to HOX genes established during embryogenesis, and fibroblasts at different locations in the body should be considered as distinct differentiated cell types [Citation16]. During the developmental process, the dermal component is generally derived from lateral plate mesoderm and somite [Citation38], however, the dermis of the face and ventral neck area is specifically differentiated from neural crest cells via formation of mesoectoderm [Citation38,39]. We believe that these developmental differences are the cause of the functional differences observed in our study. Future studies should aim to clarify more fundamental differences between facial and dermal fibroblasts, similar to the work of Yamaguchi et al., which revealed that the physiological differences in melanin pigment between palmoplantar and nonpalmoplantar skin are associated with elevated expression of dickkopf-1, an inhibitor of the canonical Wnt signaling pathway which is also associated with developmental processes [Citation18]. Further clarification of key factors in the anatomical differences in the scarring properties of fibroblasts may contribute to future therapeutic intervention for problematic wound scarring, such as conspicuous scars resulting from excess scar formation, hypertrophic scarring, or keloids.
Conclusions
Our study demonstrated that facial and trunk dermal fibroblasts in the superficial and deep dermis possess identical proliferative capacity, but that facial dermal fibroblasts show lower fibrotic activity in mRNA expression and protein production analyses. The differences in functional properties of facial and trunk dermal fibroblasts were consistent with the clinical healing tendencies of facial and trunk wounds. Thus, the differences between facial and trunk scarring are, in part, related to the intrinsic nature of the local dermal fibroblasts.
Acknowledgment
This work was supported by a grant from the Japanese Ministry of Education, Culture, Sports, Science, and Technology (MEXT) KAKENHI 21689046 (Grant-in-Aid for Young Scientists A) and 20390457 (Grant-in-Aid for Scientific Research B).
Declaration of interest
The authors declare that they have no other competing financial interests.
References
- Martin, P. (1997). Wound healing – Aiming for perfect skin regeneration. Science 276:75–81.
- Fries, K.M., Blieden, T., Looney, R.J., Sempowski, G.D., Silvera, M.R., Willis, R.A., and Phipps, R.P. (1994). Evidence of fibroblast heterogeneity and the role of fibroblast subpopulations in fibrosis. Clin. Immunol. Immunopathol. 72:283–292.
- Sorrell, J.M., and Caplan, A.I. (2004). Fibroblast heterogeneity: More than skin deep. J. Cell Sci. 117:667–675.
- Sorrell, J.M., Baber, M.A., and Caplan, A.I. (2004). Site-matched papillary and reticular human dermal fibroblasts differ in their release of specific growth factors/cytokines and in their interaction with keratinocytes. J. Cell. Physiol. 200:134–145.
- Sorrell, J.M., Baber, M.A., and Caplan, A.I. (2008). Human dermal fibroblast subpopulations; differential interactions with vascular endothelial cells in coculture: Nonsoluble factors in the extracellular matrix influence interactions. Wound Repair Regen. 16:300–309.
- Smith, J.C., Boone, B.E., Opalenik, S.R., Williams, S.M., and Russell, S.B. (2008). Gene profiling of keloid fibroblasts shows altered expression in multiple fibrosis-associated pathways. J. Invest. Dermatol. 128:1298–1310.
- Dasu, M.R., Hawkins, H.K., Barrow, R.E., Xue, H., and Herndon, D.N. (2004). Gene expression profiles from hypertrophic scar fibroblasts before and after IL-6 stimulation. J. Pathol. 202:476–485.
- Jelaska, A., Arakawa, M., Broketa, G., and Korn, J.H. (1996). Heterogeneity of collagen synthesis in normal and systemic sclerosis skin fibroblasts. Increased proportion of high collagen-producing cells in systemic sclerosis fibroblasts. Arthritis Rheum. 39:1338–1346.
- De Schepper, S., Boucneau, J., Vander Haeghen, Y., Messiaen, L., Naeyaert, J.M., and Lambert, J. (2006). Café-au-lait spots in neurofibromatosis type 1 and in healthy control individuals: Hyperpigmentation of a different kind? Arch. Dermatol. Res. 297:439–449.
- Okazaki, M., Yoshimura, K., Suzuki, Y., Uchida, G., Kitano, Y., Harii, K., and Imokawa, G. (2003). The mechanism of epidermal hyperpigmentation in café-au-lait macules of neurofibromatosis type 1 (von Recklinghausen’s disease) may be associated with dermal fibroblast-derived stem cell factor and hepatocyte growth factor. Br. J. Dermatol. 148:689–697.
- Ali-Bahar, M., Bauer, B., Tredget, E.E., and Ghahary, A. (2004). Dermal fibroblasts from different layers of human skin are heterogeneous in expression of collagenase and types I and III procollagen mRNA. Wound Repair Regen. 12:175–182.
- Wang, J., Dodd, C., Shankowsky, H.A., Scott, P.G., Tredget, E.E., and Wound Healing Research Group. (2008). Deep dermal fibroblasts contribute to hypertrophic scarring. Lab. Invest. 88:1278–1290.
- Harper, R.A., and Grove, G. (1979). Human skin fibroblasts derived from papillary and reticular dermis: Differences in growth potential in vitro. Science 204:526–527.
- Azzarone, B., and Macieira-Coelho, A. (1982). Heterogeneity of the kinetics of proliferation within human skin fibroblastic cell populations. J. Cell. Sci 57:177–187.
- Schafer, I.A., Pandy, M., Ferguson, R., and Davis, B.R. (1985). Comparative observation of fibroblasts derived from the papillary and reticular dermis of infants and adults: Growth kinetics, packing density ant confluence and surface morphology. Mech. Ageing Dev. 31:275–293.
- Chang, H.Y., Chi, J.T., Dudoit, S., Bondre, C., van de Rijn, M., Botstein, D., and Brown, P.O. (2002). Diversity, topographic differentiation, and positional memory in human fibroblasts. Proc. Natl. Acad. Sci. USA 99(20):12877–12882.
- Rinn, J.L., Bondre, C., Gladstone, H.B., Brown, P.O., and Chang, H.Y. (2006). Anatomic demarcation by positional variation in fibroblast gene expression programs. PLoS Genet. 2:e119.
- Yamaguchi, Y., Passeron, T., Watabe, H., Yasumoto, K., Rouzaud, F., Hoashi, T., and Hearing, V.J. (2007). The effects of dickkopf 1 on gene expression and Wnt signaling by melanocytes: Mechanisms underlying its suppression of melanocyte function and proliferation. J. Invest. Dermatol. 127:1217–1225.
- Ferguson, M.W., and O’Kane, S. (2004). Scar-free healing: From embryonic mechanisms to adult therapeutic intervention. Philos. Trans. R. Soc. Lond. B Biol. Sci. 359:839–850.
- Gangemi, E.N., Gregori, D., Berchialla, P., Zingarelli, E., Cairo, M., Bollero, D., Ganem, J., Capocelli, R., Cuccuru, F., Cassano, P., Risso, D., and Stella, M. (2008). Epidemiology and risk factors for pathologic scarring after burn wounds. Arch. Facial. Plast. Surg. 10:93–102.
- Thiele, B.J., Doller, A., Kähne, T., Pregla, R., Hetzer, R., and Regitz-Zagrosek, V. (2004). RNA-binding proteins heterogeneous nuclear ribonucleoprotein A1, E1, and K are involved in post-transcriptional control of collagen I and III synthesis. Circ. Res. 95:1058–1066.
- Suga, H., Matsumoto, D., Eto, H., Inoue, K., Aoi, N., Kato, H., Araki, J., and Yoshimura, K. (2009). Functional implications of CD34 expression in human adipose-derived stem/progenitor cells. Stem Cells Dev. 18:1201–1210.
- Goldberg, M.T., Han, Y.P., Yan, C., Shaw, M.C., and Garner, W.L. (2007). TNF-alpha suppresses alpha-smooth muscle actin expression in human dermal fibroblasts: An implication for abnormal wound healing. J. Invest. Dermatol. 127:2645–2655.
- Pohlers, D., Beyer, A., Koczan, D., Wilhelm, T., Thiesen, H.J., and Kinne, R.W. (2007). Constitutive upregulation of the transforming growth factor-beta pathway in rheumatoid arthritis synovial fibroblasts. Arthritis. Res. Ther. 9:R59.
- Quesnel, C., Marchand-Adam, S., Fabre, A., Marchal-Somme, J., Philip, I., Lasocki, S., Leçon, V., Crestani, B., and Dehoux, M. (2008). Regulation of hepatocyte growth factor secretion by fibroblasts in patients with acute lung injury. Am. J. Physiol. Lung. Cell. Mol. Physiol. 294:L334–L343.
- Ala-Kokko, L., Rintala, A., and Savolainen, E.R. (1987). Collagen gene expression in keloid. Analysis of collagen metabolism and type I, III, IV and V procollagen mRNAs in keloid tissue and keloid fibroblast cultures. J. Invest. Dermatol. 89:238–244
- Ghahary, A., Shen, Y.J., Nedelec, B., Wang, R., Scott, P.G., and Tredget, E.E. (1996). Collagenase production is lower in post-burn hypertrophic scar fibroblasts than normal fibroblasts and is down-regulated by insulin-like growth factor-1. J. Invest. Dermatol. 106:476–481.
- Derynck, R., and Zhang, Y.E. (2003). Smad-dependent and Smad-independent pathways in TGF-β family signalling. Nature 425:577–584.
- Amjad, S.B., Carachi, R., and Edward, M. (2007). Keratinocyte regulation of TGF-beta and connective tissue growth factor expression: A role in suppression of scar tissue formation. Wound Repair Regen. 15:748–755.
- Barrientos, S., Stojadinovic, O., Golinko, M.S., Brem, H., and Tomic-Canic, M. (2008). Growth factors and cytokines in wound healing. Wound Repair Regen. 16:585–601.
- Shah, M., Foreman, D.M., and Ferguson, M.W. (1995). Neutralisation of TGF-beta 1 and TGF-beta 2 or exogenous addition of TGF-beta 3 to cutaneous rat wounds reduces scarring. J. Cell. Sci. 108:985–1002.
- Frazier, K., Williams, S., Kothapalli, D., Klapper, H., and Grotendorst, G.R. (1996). Stimulation of fibroblast cell growth, matrix production, and granulation tissue formation by connective tissue growth factor. J. Invest. Dermatol. 107:404–411.
- Wu, L., Siddiqui, A., Morris, D.E., Cox, D.A., Roth, S.I., and Mustoe, T.A. (1997). Transforming growth factor beta 3 (TGF beta 3) accelerates wound healing without alteration of scar prominence. Histologic and competitive reverse-transcription polymerase chain reaction studies. Arch. Surg. 132:753–760.
- Murata, H., Zhou, L., Ochoa, S., Hasan, A., Badiavas, E., and Falanga, V. (1997). F-beta3 stimulates and regulates collagen synthesis through TGF-beta1-dependent and independent mechanisms. J. Invest. Dermatol. 108:258–262.
- Ueki, T., Kaneda, Y., Tsutsui, H., Nakanishi, K., Sawa, Y., Morishita, R., Matsumoto, K., Nakamura, T., Takahashi, H., Okamoto, E., and Fujimoto, J. (1999). Hepatocyte growth factor gene therapy of liver cirrhosis in rats. Nat. Med. 5:226–230.
- Liu, Y. (2004). Hepatocyte growth factor in kidney fibrosis: Therapeutic potential and mechanisms of action. Am. J. Physiol. Renal. Physiol. 287:7–16.
- Suga, H., Eto, H., Shigeura, T., Inoue, K., Aoi, N., Kato, H., Nishimura, S., Manabe, I., Gonda, K., and Yoshimura, K. (2009). S collection: Fibroblast growth factor-2-induced hepatocyte growth factor secretion by adipose-derived stromal cells inhibits postinjury fibrogenesis through a c-Jun N-terminal kinase-dependent mechanism. Stem Cells 27:238–249.
- Sadler, T.W. (2009). Langman’s Medical Embryology, 11th ed. Philadelphia: Lippincott Williams & Wilkins.
- Nakamura, H. (1982). Mesenchymal derivatives from the neural crest. Arch. Histol. Jpn. 45:127–138.