Abstract
Intervertebral discs comprise the largest avascular cartilaginous organ in the body, and its nutrient condition can be impaired by degeneration, aging and even metabolic disease. The unique microenvironment brings special stresses to various disc cell types, including nucleus pulposus cells, notochordal cells, annulus fibrosus cells and endplate chondrocytes. These cells experience nutrient starvation, acidic stress, hypoxic stress, hyperglycemic stress, osmotic stress and mechanical stress. Understanding the detailed responses and complex adaptive mechanisms of disc cells to various stresses might provide some clues to guide therapy for disc degeneration. By reviewing the published literatures describing disc cells under different hostile microenvironments, we conclude that these cells exhibit different responses to microenvironmental stresses with different mechanisms. Moreover, the interaction and combination of these stresses create a complex environment that synergistically increase or decrease influences on disc cells, compared with the effects of a single stress. Interestingly, most of these stresses activate autophagy, a self-protective mechanism by which dysfunctional protein and organelles are degraded. It is becoming clear that autophagy facilitates the cellular adaptation to stresses and might play a central role in regulating the adaptation of disc cells under stress. Therefore, autophagy modulation might be a potential therapeutic method to treat disc degeneration.
Introduction
Intervertebral disc degeneration (IDD), the primary cause of low back pain, is considered to arise from abnormal mechanical loading, unfavorable inheritance, smoking, obesity and aging (Citation1–4). However, cell death has recently been considered an important contributor to IDD (Citation5). Disc cells, including nucleus pulposus (NP) cells, annulus fibrosus (AF) cells, notochordal cells (NCs) and endplate chondrocytes, produce extracellular matrix (ECM) and maintain biomechanical function. To maintain the balance of ECM synthesis and degradation in discs, these cells secrete matrix metalloproteinases (MMPs) and disintegrin and metalloproteinase with thrombospondin motifs (ADAMTSs), as well as their inhibitors, tissue inhibitors of metalloproteinases (TIMPs) (Citation6). Excessive levels of MMPs and ADAMTSs disturb the balance by degrading collagen II and aggrecan. Given the importance of disc cells, understanding the responses, adaptations and related mechanisms of these cells to microenvironmental stresses is crucial for developing new therapeutic approaches to treat IDD.
Lying between the vertebrae, intervertebral discs (IVDs) comprise the largest avascular cartilaginous organ in the body, and its nutrient supply depends on the diffusion and convection through the cartilaginous endplate (CEP) and outer AF, neither of which are efficient transport mechanisms (Citation7,Citation8). Material transport, including the import of essential nutrients and the export of metabolic wastes, can be interrupted by endplate calcification and mechanical loading (Citation9). In addition, the extracellular environment can be further damaged by degeneration, aging and even metabolic diseases, such as diabetes mellitus. Given the unique microenvironment, these cells, especially the NP cells, experience various stresses including starvation, acidic stress, hyperglycemia, hypoxia and even mechanical stress. Under these stresses, cellular physiological processes such as proliferation, growth and protein synthesis could be impaired to some extent, resulting in a series of changes, such as cell cluster formation, viability loss, cell senescence, apoptosis and autophagy (Citation10).
In order to relieve stress and reinstate homeostasis, most mammalian cells rely on intrinsic adaptive mechanisms, such as cell cycle arrest, programed cell death and macroautophagy referred to simply as “autophagy” (Citation11). Autophagy can be induced in cultured mammalian cells by a variety of stress stimuli, including hypoxia, starvation, endoplasmic reticulum stress and acidic stress (Citation12).
Although there are many reviews regarding IVD nutrition (Citation7,Citation13,Citation14), there are few reviews detailing the responses and adaptations of disc cells. Hence, we examined the existing evidence for the response and adaptation of disc cells under different microenvironmental stresses, as well as the role of autophagy in disc cell adaptation, to enhance the understanding of the mechanisms involved in IDD, which will hopefully guide future research and identify novel treatments.
Autophagy
As a self-protective mechanism, autophagy degrades dysfunctional cellular organelles and proteins by lysosomal enzymes (Citation15). When confronted with dramatic extracellular environment changes or pathogen invasion, mammalian cells can create double-membraned vesicles named autophagosomes in the cytoplasm, sequestering the organelles and protein. Autophagosomes fuse with lysosomes to produce autophagolysosomes and then degrade the sequestered contents with proteolytic enzymes in the lysosomes () (Citation16). Generally, the basal level of autophagy controls cellular quality; however, the exact role of autophagy in stressed cells is extremely complicated and has aroused great interest in skeletal biology (Citation15). Hostile environments induce cells to upregulate autophagy to remove toxic protein aggregates and provide amino acids and fatty acids. Autophagy activation has been shown to ameliorate the progression of experimental rat osteoarthritis (Citation17). Conversely, loss of autophagy can lead to Parkinson's disease and cardiac dysfunction (Citation18,Citation19). However, autophagy plays dichotomous roles in several diseases. Excessive autophagy might exacerbate the death of chondrocytes and synovial fibroblasts in rheumatoid arthritis (Citation20,Citation21). “Autophagic cell death”, which refers to cell death-associated autophagy, is extensively used in studies about autophagy, though direct and precise evidence demonstrating that autophagy leads to the execution of cell death remains limited (Citation22).
Figure 1. Autophagy induction and execution in disc cells. Under different stresses and following rapamycin treatment, disc cells produce a double-membrane structure termed as a phagophore, which engulfs dysfunctional organelles and proteins. As the vesicle elongates, the phagophore becomes the autophagosome, which fuses with the lysosome, producing the autolysosome and degrading the sequestered contents with proteolytic enzymes.
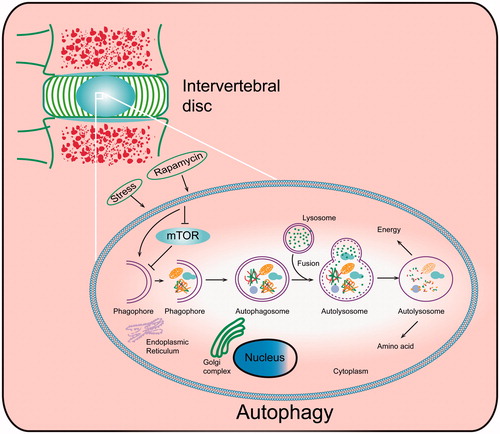
Depending on whether the mammalian target of rapamycin (mTOR) is involved or not, autophagy activation pathways are divided into mTOR-dependent and mTOR-independent (Citation23). mTOR inhibition could promote autophagy; mTOR in the mTOR complex 1 (mTORC1), a highly conserved serine/threonine protein kinase, is associated with cell growth, proliferation and survival (Citation24). Microenvironmental stress suppresses the phosphorylation of mTOR and its substrates, including the 4E-binding protein 1 (4E-BP1) and ribosomal S6 protein kinase (p70S6K), impairing the signal translation and protein production (Citation25). mTOR combines with autophagy-related genes (ATGs) to regulate autophagy initiation, formation and degradation (Citation23). Atg5, 6, 8 (Atg5, Beclin-1 and LC-3 in mammals) are the main regulators of autophagic formation; therefore, they are usually utilized as markers to detect autophagy.
Apoptosis and autophagy, both forms of programed cell death, can be sequentially induced by the same stress, but there are many differences and interactions between the two processes (Citation26). Compared with the intracellular organelle changes that occur during autophagy activation, the process of apoptosis involves a series of changes that affect cellular morphology, ultimately resulting in apoptotic body formation (Citation27). The activation of apoptosis accompanied by increased levels of caspases and catabolic hydrolases leads to cell death; however, proper autophagy activation protects cells against stresses (Citation27). Only when the stress reaches a lethal level does the over-activation of autophagy promote cell death. Within the same environment, autophagy interacts with apoptosis, forming a complex network to help cells confront and adapt to hostile stress (Citation26).
Starvation stress
Cell starvation is the first and main stress experienced by disc cells due to decreased nutritional diffusion (for small molecules) and convection (for large molecules) through the CEP and AF in the disc. Because it is difficult to perform nutrition research in vivo, glucose- or serum-free medium is usually used to assess the response and adaptation of AF and NP cells to starvation conditions (Citation28,Citation29) ().
Table 1. Responses and adaptations of disc cells to starvation.
Cell numbers decrease from the periphery of the AF to the NP as the nutrition level declines toward the NP (Citation30). Glycolysis is the main process by which NP cells obtain energy (Citation31); therefore, glucose levels in discs determine NP cell viability. Using agarose gels and chambers to mimic nutrient diffusion through the endplate, Horner et al. (Citation32) found that the bovine NP cell viability decreased with the increased cell density and greater distance from the medium, and viability was also significantly suppressed by glucose-free medium (Citation32,Citation33). When subjected to serum starvation, apoptosis and G1 arrest were induced in AF and NP cells isolated from rat and human discs (Citation28,Citation34–36). However, transfection of recombinant human telomerase reverse transcriptase (hTERT) gene attenuated the apoptosis and cell arrest previous negative influences, suggesting the importance of senescence in the cellular response to starvation (Citation35). Additionally, microarray analysis identified a group of genes that was upregulated by starvation, mainly including genes involved in DNA damage checkpoints (Citation37). Therefore, it is reasonable to conclude that starvation could induce disc cells to become senescent. In terms of cell function, serum starvation inhibited the expression of collagen II and aggrecan (Citation38).
The balance of nutrient supply and cellular demand determines cell viability under starvation conditions. When nutrients, especially amino acids, are scarce in culture medium, some cancer cells respond by impeding the biosynthetic machinery via inhibiting mTOR and activating autophagy to maintain the energy balance (Citation39). In chondrocytes, AMP-activated protein kinase (AMPK), an enzyme that could regulate cellular energy status, was also involved in autophagy upregulation (Citation40). Although multiple lines of evidence have suggested a role for sirtuins in the induction of autophagy, no similar study has yet been reported in the cartilage or disc (Citation41). The sirtuins, a conserved family of class III histone deacetylases, regulate a variety of cellular processes, including autophagy, senescence and apoptosis by consuming the nicotinamide adenine dinucleotide (NAD+) (Citation42). During the process of disc aging and degeneration, the nutrition supply declines due to calcification of endplate cartilage; therefore, autophagy increased in the aged and degenerated NP to address the tension between nutrient supply and demand (Citation43,Citation44). In vitro, rat AF cells subjected to starvation with Earle's balanced salts solution and 1% fetal bovine serum (FBS) in Dulbecco’s minimum essential medium (DMEM) expressed higher levels of autophagy compared to cells grown in control conditions, and autophagy inhibited starvation-induced apoptosis (Citation28,Citation43), indicating that autophagy might be a major mechanism to improve the adaptive ability of disc cells in low-nutrient microenvironments. We also found a similar adaptive mechanism in rat NP cells (Citation45). Furthermore, autophagy could even supply amino acids to yeast cells under starvation, which might be an important energy source (Citation46). However, the role of mTOR in disc cells experiencing nutritional changes is not fully understood. Meanwhile, some other adaptive mechanisms have also been reported in discs. Transfection of the bcl-2 gene could directly inhibit apoptosis, inhibit caspase3 activation, and increase the expression of collagen II and aggrecan mRNA (Citation38). In addition, the above-mentioned genes identified with microarray analysis might be promising targets to protect NP cells under starvation conditions.
Acidic stress
Due to ischemia and hypoxia, disc cells, especially NP cells, mainly rely on glycolysis for ATP production, even under aerobic conditions (Citation13,Citation14). Therefore, extracellular acidity is an inevitable consequence of increased lactic acid produced by anaerobic glycolysis and the slow diffusion of lactic acid across the matrix. In addition, free cations, including H+ ions, could be attracted by the numerous negative-charged groups in glycosaminoglycan (GAG) in the disc, leading to a high concentration of free cations and low PH around NP cells (Citation47). Compared with that in cartilage, the matrix acidity in a degenerated disc is even more pronounced (Citation48), with pH value dropping dramatically from 7.0 to 7.2 in healthy discs to 5.7–6.5 in degenerated ones (Citation49–51). In addition, the intracellular pH of NP cells is typically maintained at 6.0 as a result of high lactic acid production.
The viability and function of different disc cells can be impaired by the acidic microenvironment (). Our previous study demonstrated that acid promoted apoptosis of rat endplate chondrocytes via the ovarian cancer G protein-coupled receptor 1 (OGR1) (Citation52). Similarly, articular chondrocytes also underwent apoptosis due to the acidic microenvironment (Citation53). On the other hand, Razaq et al. (Citation54) found that when the pH of the extracellular environment decreased from 7.4 to 6.3, the production of sulfated GAG and TIMP-1 in bovine NP cells was significantly inhibited. Consistent with this, Ohshima et al. (Citation55) found that proteoglycan synthesis in human NP peaked at pH 7.2–6.9 and declined steeply below pH 6.8, resulting in only 20% of normal production at pH 6.3. Importantly, the research excluded the effect of lactic acid itself on the acid-induced negative influence (Citation55). When analyzing the influence of interactions of different microenvironments on NP cells, Neidlinger-Wilke et al. (Citation56) found that ECM mRNA levels were suppressed by acid. However, while TIMP was inhibited in an acidic microenvironment, MMPs were found to be insensitive to the pH change, leading to an imbalance of pro- and anti-ECM degradation (Citation54).
Table 2. Responses and adaptations of disc cells to acidic stress.
Recent studies focusing on the effect of acid on chondrocytes have revealed an important role of acid-sensing ion channels (ASICs) (Citation57,Citation58). ASICs are a superfamily of amiloride-sensitive cationic channels and have six subunits: ASIC1a, ASIC1b, ASIC2a, ASIC2b, ASIC3, ASIC4. In the rat chondrocyte, blocking ASICs with amiloride prevented apoptosis in vitro (Citation59). ASIC3 was detected in NP cells and was suggested to act as a protective mediator in acidic microenvironments (Citation60,Citation61). However, there is limited research into whether ASICs mediate the influence of acidic environments on disc cells. Interestingly, ASICs are involved in the nociceptive signaling of nerves stimulated by acid and inflammatory factors, both of which can be found in fissured and degenerated AF (Citation62,Citation63), indicating a potential protective role of ASICs in discogenic pain.
An increasing number of studies have demonstrated an association between acidic stress and autophagy (Citation64–66). Acidic microenvironments promote autophagy in melanoma cells, while inhibition of autophagy with small interfering RNA for ATG5 significantly increased cell death (Citation64). ATG5 is an essential protein for the formation of pre-autophagosomes (Citation67). It has been reported that extracellular acidification could inhibit the phosphorylation of mTOR, which controls protein synthesis and energy expenditure (Citation68). AMPK and Ca2+ are the main messengers mediating the effect of acid on mTOR (Citation68). Under acidic stress, inhibition of mTOR might induce disc cells to restrain energy-consuming anabolic processes to adapt to inhospitable microenvironments. We recently found that lactic acid could enhance the level of autophagy in rat NP cells, providing further support for this hypothesis (Citation69). The further revelation of a possible protective role of autophagy in disc cells against acidic microenvironments might be an important step to prevent IDD.
Hyperglycemia stress
Disc cell viability is inhibited by both low and high glucose (). Clinically, the incidence of diabetes mellitus in patients with lumbar or cervical IVD herniation who undergo surgery is significantly higher than that in the age-matched patients (Citation70,Citation71). The outcome of spinal surgery for patients with co-morbid diabetes and degenerated spinal disease is poorer than those without diabetes (Citation72). We previously reported that IVDs from rats with type I diabetes mellitus had reduced mRNA and protein levels of aggrecan and collagen II compared with normal discs (Citation73). The spontaneously diabetic Otsuka-Long-Evans-Tokushima fatty (OLETF) rats exhibited a higher degree of transition to fibrocartilaginous NP, indicating that NP in the diabetic rat was more inclined to be premature (Citation74). Consequently, disc height and NP and AF cell morphologies and viabilities were altered by diabetes (Citation75).
Table 3. Responses and adaptations of disc cells to hyperglycemia stress.
NC proliferation was inhibited by high glucose from 0.1 to 0.4 M in a dose- and time-dependent manner (Citation76). It has been demonstrated that diabetes or high glucose could enhance apoptosis of rat NPs and NCs both in vitro and in vivo (Citation73,Citation74,Citation76). The specific mechanism underlying the induction of apoptosis by high glucose might be the mitochondrial (intrinsic) pathway, evidenced by increased levels of Bid, cytochrome-c and caspase-9 proteins rather than caspase-8 protein (Citation76). However, we found that caspase-8 was also enhanced in diabetic rats, indicating the differences of apoptotic signal pathways between in vitro and in vivo conditions (Citation73). The role of the endoplasmic reticulum pathway in hyperglycemia-induced apoptosis remains to be elucidated (Citation77,Citation78).
In terms of in vivo cell function, levels of MMPs, including MMP-1, -2, -3 and -13, were all enhanced in diabetic rats (Citation74,Citation75). Compared with the significant increase of MMP expression, TIMP-2 was only slightly elevated in rats, which resulted in a lower net inhibitory effect on MMP activity (Citation74). The results of in vitro and in vivo studies were similar (Citation76). The increased levels and activities of MMPs in diabetic discs directly inhibited GAG expression (Citation75).
In order to further determine the mechanisms and materials involved in the above-described effects of high glucose levels, rat and human NP cells were treated with advanced glycation end products (AGEs) (Citation79). AGEs are the products of glycation reactions in the setting of high glucose concentrations. As expected, AGEs increased the expressions of receptor of AGE (RAGE), MMP2 and phospho-extracellular-signal-regulated kinase (P-ERK), suggesting that the interaction between AGEs and RAGE activated the ERK pathway and led to an increased MMP expression (Citation79). Similarly, in vivo research revealed that AGE accumulation in diabetes-induced degenerated discs was associated with the high expression of tumor necrosis factor (TNF)-α in the NP and AF of streptozotocin-treated mice (Citation75). Therefore, inflammation might also mediate disc degeneration in diabetic subjects. Furthermore, the mitochondrial transmembrane potential of both NC and NP cells could be impaired by high glucose levels due to increased production of reactive oxygen species (ROS) (Citation80–82). Generally, AGEs bind with RAGEs to increase ROS levels, causing oxidative stress and activating mitochondria-mediated apoptosis. In addition, the expressions of manganese superoxide dismutase (MnSOD) and catalase were also adaptively increased (Citation81). Illien-Junger et al. (Citation75) found that the combination of pyridoxamine (PYR, an AGE inhibitor) and pentosanpolysulfate (PPS, a broad acting anti-inflammatory) could maintain disc height, attenuate NP cell morphology, and decelerate the increased expression of MMP-13 and ADAMTS-5 in diabetic mice. The results indirectly demonstrated the role of inflammation and AGEs in diabetes-induced degeneration. In conclusion, the binding of AGEs and RAGEs might induce both inflammation and oxidative stress, leading to a sequence of consequences, including apoptosis, autophagy and catabolic metabolism.
When confronted with hyperglycemia stress, different disc cells could rely on their intrinsic adaptive mechanisms. In response to mitochondrial damage and apoptosis, the levels of antioxidants and autophagy in NC cells were adaptively enhanced by high glucose (Citation83). Similarly, our research also showed that NP cell autophagy was increased in the type I diabetic rat disc (Citation73). Recently, autophagy has been hypothesized to prevent oxidative stress and inflammation in the setting of diabetes (Citation84). By degrading and recycling damaged proteins and organelles, autophagy could maintain mitochondrial integrity and even engulf impaired mitochondria to regulate oxidative stress and inflammation (Citation85,Citation86). In addition, the inhibitory effect of autophagy on apoptosis has been demonstrated with convincing research in osteoarthritis and IDD (Citation28,Citation87). However, the protective role of autophagy in diabetes-induced disc degeneration remains unclear and requires further elucidation. Given the above evidence, drugs that could both promote autophagy and exert anti-inflammation, anti-oxidative and anti-AGEs effects might be useful for regulating homeostasis within disc cells under hyperglycemic stress.
Hypoxic stress
Given its limited vascular distribution, the disc has a slower oxidative metabolism than other tissues (Citation7,Citation88). However, the rate of oxygen consumption is still significant in the disc. The partial pressure of oxygen (pO2) value in the normal outer AF is 7.5 kPa, leaving only 0.5 kPa oxygen in the NP (Citation88,Citation89). Therefore, different disc cells, especially NP cells, are exposed to hypoxia, even in normal conditions. NP cells have adapted to the hypoxia microenvironment, which is to some extent a protective factor. Compared with a normoxic environment, hypoxia ensures a higher survival rate, less apoptosis and greater production of GAG and collagen II in NP cells through a hypoxia inducible factor (HIF)-dependent mechanism (Citation33,Citation90). Because the response of NP cells to hypoxia has been described in detail in previous reviews (Citation10,Citation91), this review mainly focuses on the association between autophagy and hypoxia.
During evolution, disc cells have developed adaptive responses to sustained hypoxia, and these adaptive mechanisms protect discs against further damage. HIF-1, -2 and -3 are transcription factors with important roles in this process (Citation91,Citation92). The expressions of HIF-1α, β and HIF-2α have been detected in NP cells under hypoxia stress. However, only HIF-1β was found in rat AF cells in vivo (Citation93). In a hypoxic microenvironment, the α-subunits of HIF are stable and dimerize with the other subunits to bind hypoxia response elements (HREs) and promote the expression of protective mRNAs and proteins, such as vascular endothelial growth factor (VEGF), cited2 and galectin-3 to inhibit apoptosis (Citation94–96). With regard to the ECM, HIF-1 was reported to promote the synthesis of aggrecan, which is the major proteoglycan expressed in discs (Citation97).
Despite intensive research into the role of HIF in disc cell survival, there are few studies regarding the association between HIF and autophagy in disc cells. HIF-1 activates autophagy in the mouse chondrocyte by promoting mitogen-activated protein kinase (MAPK) and inhibiting mTOR (Citation40,Citation98). By contrast, suppression or knockout of HIF-2 enhanced autophagy, suggesting the inverse regulation of HIF-1 and HIF-2 in autophagy (Citation99). The biochemical characteristics of NP cells are similar to those of chondrocytes; therefore, the relationship between autophagy and HIF in chondrocytes might also exist in NP cells. As mentioned by Risbud et al. (Citation92), the regulation of oxemic conditions within the environment could be a promising approach to restore NP cell function, but the importance of autophagy in the hypoxic disc remains to be elucidated.
Mechanical stress
Embedded in the space between the adjacent vertebrae that bear weight, IVDs experience hydrostatic pressure and tensile stress under normal physiological conditions (Citation100). The mechanics are changed dramatically in degenerated discs; hence, biomechanical alterations have always been regarded as an important cause of IDD.
Unphysiological loading reduces cell viability and stimulates NP and AF cell apoptosis (Citation101–104) (). Under axial 20.8 -N compression for 3 days, the apoptotic incidence of rat tail NP cells was significantly increased (Citation101). Similar to NP cells, the 20% elongation with a frequency of 0.5 Hz could induce apoptosis in ∼28% of rat AF cells after 48 h (Citation105). The pathways involved in disc cell apoptosis induced by mechanical stress mainly include the mitochondrial and endoplasmic reticulum stress pathways (Citation102,Citation105,Citation106). Static compression of 1.3 MPa on rat tail discs for 7 days could even reproduce the process of disc degeneration with the graduate loss of NC cells (Citation107). In addition, dynamic and static compression both promoted the production of total ATP and lactate in porcine AF and NP cells, leading to decreased disc pH (Citation108). Following cell viability changes, the ECM and matrix-degrading enzyme could also be altered by loading changes (Citation107,Citation109). For example, human NP cells in tissue under dynamic compression for 2 h exhibited increased ADAMTS expression and decreased aggrecan expression (Citation109).
Table 4. Responses and adaptations of disc cells to mechanical stress.
Despite intensive research into the responses of different disc cells under mechanical stress, there are few studies of autophagy under loading. Ma et al. (Citation110) found that autophagy triggered by compression in rat NP cells was associated with apoptosis and intracellular ROS production. However, elucidation of the exact role of autophagy and the precise mechanism mediating autophagy activation require further investigation.
Interactions of starvation, hypoxia, acid and mechanics
Given the complexity of discs in vivo, none of the above factors act alone. For example, in vitro cell viability is further impaired by a combination of glucose starvation and extracellular acidity, compared with the effect of a single variable (Citation33). In contrast, a hypoxic microenvironment could prevent apoptosis and protect NP cells in starvation and acidic microenvironments (Citation33,Citation34). Furthermore, these stresses could also influence each other. Porcine AF cells cultured in higher glucose levels exhibited lower oxygen consumption in sealed metabolism chambers (Citation111). An acidic microenvironment was also shown to reduce the oxygen consumption of bovine NP cells (Citation112). However, porcine NP cell oxygen consumption was insensitive to glucose level changes (Citation111). Conversely, the lactic acid production of bovine NP cells, the main source of a low pH microenvironment, was inhibited by high-glucose medium (Citation33). Experiments with a poromechanical finite element model of a human disc revealed that external loading regulates the regional distributions of oxygen and lactate in discs, which was more obvious in the healthy disc (Citation113). Taken together, these different factors form a unique and complex microenvironment, in which different disc cells and even transplanted stem cells must respond to different stresses (Citation10).
The central role of autophagy in the adaptation
Based on the previous findings, autophagy plays a central role in disc cell adaptation to different extracellular microenvironment stresses (). Autophagy can be activated by starvation, acid, hypoxia and hyperglycemia, as well as mechanical loading, suggesting that autophagy activation might be a common adaptive mechanism of stressed disc cells. In a hostile microenvironment, autophagy inhibits apoptosis, regulates energy metabolism and even supplies amino acids to different disc cells to decelerate IDD progression. However, the degree of autophagic activation should be controlled to avoid negative influences on cell viability. It is still unknown whether autophagy plays dual roles in discs, and future studies should investigate this interesting point. Senescence, another important change of NP and AF cells, has also been demonstrated to participate in the degeneration process (Citation73,Citation114,Citation115). However, research regarding the association between autophagy and senescence in discs remains limited. Given that cellular starvation occurs even in normal discs, the role of autophagy in normal and hostile microenvironments must be elucidated. Moreover, further explorations of autophagy in discs, especially in vivo studies, might identify useful therapeutic targets for IDD.
Figure 2. The central role of autophagy in regulating the adaptive mechanism of disc cells under different stresses. Compression, starvation, acid, hypoxia and high glucose have all been reported to induce autophagy in disc cells. The dotted line indicates that the pathway has been demonstrated in chondrocyte but not yet in disc cells.
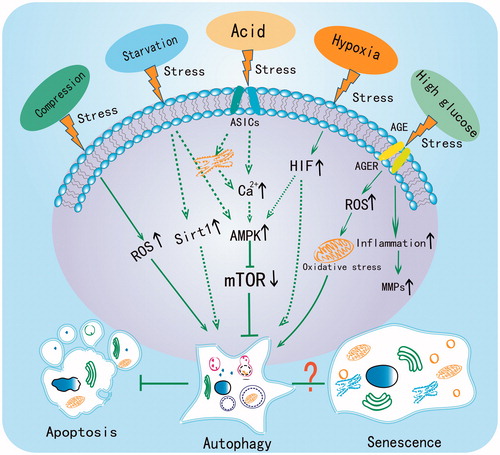
In summary
As a unique organ in the body, the extracellular microenvironment of IVDs can be influenced by aging, degeneration and even metabolic diseases, and the cells must endure starvation, acidity, hypoxia and high glucose. Under these stresses, different types of disc cells exhibit various responses, including apoptosis, senescence, decreased viability and loss of matrix secretion. However, these different stresses have one thing in common – most induce autophagy, which might serve as a central regulator to protect cells against the hostile microenvironment. One shortcoming of the existing literature is that studies were carried out in rodents, and the cells populated in the NP of these animals are NC cells, which are different from those found in adult human discs. Future research, especially in human discs, is required to provide more information about disc cell survival in hostile environments and identify novel treatments for IDD.
Declaration of interest
The authors declare that they have no competing interests. All authors were involved in drafting the article, and all authors approved the final version to be published.
The research was supported by the Natural Science Foundation of China (81372002), the Natural Science Foundation of China (31170925), and the “Technology Innovation Action Plan” Key Project of Shanghai Science and Technology Commission (12411951300).
References
- Kelempisioti A, Eskola PJ, Okuloff A, Karjalainen U, Takatalo J, Daavittila I, Niinimaki J, Sequeiros RB, Tervonen O, Solovieva S, Kao PY, Song YQ, Cheung KM, Chan D, Ala-Kokko L, Jarvelin MR, Karppinen J, Mannikko M. Genetic susceptibility of intervertebral disc degeneration among young Finnish adults. BMC Med Genet 2011;12:153
- Vo N, Wang D, Sowa G, Witt W, Ngo K, Coelho P, Bedison R, Byer B, Studer R, Lee J, Di YP, Kang J. Differential effects of nicotine and tobacco smoke condensate on human annulus fibrosus cell metabolism. J Orthop Res 2011;29:1585–91
- Samartzis D, Karppinen J, Chan D, Luk KD, Cheung KM. The association of lumbar intervertebral disc degeneration on magnetic resonance imaging with body mass index in overweight and obese adults: a population-based study. Arthritis Rheum 2012;64:1488–96
- Xu HG, Li ZR, Wang H, Liu P, Xiang SN, Wang CD, Zhang XL. Intermittent cyclic mechanical tension-induced down-regulation of ectonucleotide pyrophosphatase phosphodiesterase 1 gene expression is mainly dependent on TGF-beta1 in end-plate chondrocytes. Orthop Surg 2013;5:40–5
- Ding F, Shao ZW, Xiong LM. Cell death in intervertebral disc degeneration. Apoptosis 2013;18:777–85
- Kepler CK, Ponnappan RK, Tannoury CA, Risbud MV, Anderson DG. The molecular basis of intervertebral disc degeneration. Spine J 2013;13:318–30
- Urban JP, Smith S, Fairbank JC. Nutrition of the intervertebral disc. Spine (Phila Pa 1976) 2004;29:2700–9
- Du H, Ma S, Guan M, Han B, Yang G, Zhang M, Liu M. Dynamic contrast enhanced-magnetic resonance imaging study of the nutrition pathway for lumbar intervertebral disk cartilage of normal goats. Orthop Surg 2011;3:106–12
- Huang CY, Gu WY. Effects of mechanical compression on metabolism and distribution of oxygen and lactate in intervertebral disc. J Biomech 2008;41:1184–96
- Huang YC, Leung VY, Lu WW, Luk KD. The effects of microenvironment in mesenchymal stem cell-based regeneration of intervertebral disc. Spine J 2013;13:352–62
- Vicencio JM, Galluzzi L, Tajeddine N, Ortiz C, Criollo A, Tasdemir E, Morselli E, Ben Younes A, Maiuri MC, Lavandero S, Kroemer G. Senescence, apoptosis or autophagy? When a damaged cell must decide its path – a mini-review. Gerontology 2008;54:92–9
- Kroemer G, Marino G, Levine B. Autophagy and the integrated stress response. Mol Cell 2010;40:280–93
- Grunhagen T, Wilde G, Soukane DM, Shirazi-Adl SA, Urban JP. Nutrient supply and intervertebral disc metabolism. J Bone Joint Surg Am 2006;88:30–5
- Grunhagen T, Shirazi-Adl A, Fairbank JC, Urban JP. Intervertebral disk nutrition: a review of factors influencing concentrations of nutrients and metabolites. Orthop Clin North Am 2011;42:465–77, vii
- Shapiro IM, Layfield R, Lotz M, Settembre C, Whitehouse C. Boning up on autophagy: the role of autophagy in skeletal biology. Autophagy 2013;10:7–19
- Pyo JO, Yoo SM, Jung YK. The interplay between autophagy and aging. Diabetes Metab J 2013;37:333–9
- Carames B, Hasegawa A, Taniguchi N, Miyaki S, Blanco FJ, Lotz M. Autophagy activation by rapamycin reduces severity of experimental osteoarthritis. Ann Rheum Dis 2011;71:575–81
- Wang L, Lu K, Hao H, Li X, Wang J, Wang K, Wang J, Yan Z, Zhang S, Du Y, Liu H. Decreased autophagy in rat heart induced by anti-beta1-adrenergic receptor autoantibodies contributes to the decline in mitochondrial membrane potential. PLoS One 2013;8:e81296
- Ahmed I, Liang Y, Schools S, Dawson VL, Dawson TM, Savitt JM. Development and characterization of a new Parkinson's disease model resulting from impaired autophagy. J Neurosci 2012;32:16503–9
- Chang J, Wang W, Zhang H, Hu Y, Wang M, Yin Z. The dual role of autophagy in chondrocyte responses in the pathogenesis of articular cartilage degeneration in osteoarthritis. Int J Mol Med 2013;32:1311–18
- Kato M, Ospelt C, Gay RE, Gay S, Klein K. Dual role of autophagy in stress-induced cell death in rheumatoid arthritis synovial fibroblasts. Arthritis Rheum 2013;66:40–8
- Kroemer G, Levine B. Autophagic cell death: the story of a misnomer. Nat Rev Mol Cell Biol 2008;9:1004–10
- Esclatine A, Chaumorcel M, Codogno P. Macroautophagy signaling and regulation. Curr Topics Microbiol Immunol 2009;335:33–70
- Sengupta S, Peterson TR, Sabatini DM. Regulation of the mTOR complex 1 pathway by nutrients, growth factors, and stress. Mol Cell 2010;40:310–22
- Hung CM, Garcia-Haro L, Sparks CA, Guertin DA. mTOR-dependent cell survival mechanisms. Cold Spring Harbor Perspect Biol 2012;4:a008771
- Marino G, Niso-Santano M, Baehrecke EH, Kroemer G. Self-consumption: the interplay of autophagy and apoptosis. Nat Rev Mol Cell Biol 2014;15:81–94
- Galluzzi L, Aaronson SA, Abrams J, Alnemri ES, Andrews DW, Baehrecke EH, Bazan NG, Blagosklonny MV, Blomgren K, Borner C, Bredesen DE, Brenner C, Castedo M, Cidlowski JA, Ciechanover A, Cohen GM, De Laurenzi V, De Maria R, Deshmukh M, Dynlacht BD, El-Deiry WS, Flavell RA, Fulda S, Garrido C, Golstein P, Gougeon ML, Green DR, Gronemeyer H, Hajnoczky G, Hardwick JM, Hengartner MO, Ichijo H, Jaattela M, Kepp O, Kimchi A, Klionsky DJ, Knight RA, Kornbluth S, Kumar S, Levine B, Lipton SA, Lugli E, Madeo F, Malomi W, Marine JC, Martin SJ, Medema JP, Mehlen P, Melino G, Moll UM, Morselli E, Nagata S, Nicholson DW, Nicotera P, Nunez G, Oren M, Penninger J, Pervaiz S, Peter ME, Piacentini M, Prehn JH, Puthalakath H, Rabinovich GA, Rizzuto R, Rodrigues CM, Rubinsztein DC, Rudel T, Scorrano L, Simon HU, Steller H, Tschopp J, Tsujimoto Y, Vandenabeele P, Vitale I, Vousden KH, Youle RJ, Yuan J, Zhivotovsky B, Kroemer G. Guidelines for the use and interpretation of assays for monitoring cell death in higher eukaryotes. Cell Death Differ 2009;16:1093–107
- Shen C, Yan J, Jiang LS, Dai LY. Autophagy in rat annulus fibrosus cells: evidence and possible implications. Arthritis Res Ther 2011;13:R132
- Johnson WE, Stephan S, Roberts S. The influence of serum, glucose and oxygen on intervertebral disc cell growth in vitro: implications for degenerative disc disease. Arthritis Res Ther 2008;10:R46
- Maroudas A, Stockwell RA, Nachemson A, Urban J. Factors involved in the nutrition of the human lumbar intervertebral disc: cellularity and diffusion of glucose in vitro. J Anat 1975;120:113–30
- Ishihara H, Urban JP. Effects of low oxygen concentrations and metabolic inhibitors on proteoglycan and protein synthesis rates in the intervertebral disc. J Orthop Res 1999;17:829–35
- Horner HA, Urban JP. Volvo Award Winner in basic science studies: effect of nutrient supply on the viability of cells from the nucleus pulposus of the intervertebral disc. Spine (Phila Pa 1976) 2001;26:2543–9
- Bibby SR, Urban JP. Effect of nutrient deprivation on the viability of intervertebral disc cells. Eur Spine J 2004;13:695–701
- Risbud MV, Fertala J, Vresilovic EJ, Albert TJ, Shapiro IM. Nucleus pulposus cells upregulate PI3K/Akt and MEK/ERK signaling pathways under hypoxic conditions and resist apoptosis induced by serum withdrawal. Spine (Phila Pa 1976) 2005;30:882–9
- Liang W, Ye D, Dai L, Shen Y, Xu J. Overexpression of hTERT extends replicative capacity of human nucleus pulposus cells, and protects against serum starvation-induced apoptosis and cell cycle arrest. J Cell Biochem 2012;113:2112–21
- Tapp H, Deepe R, Ingram JA, Yarmola EG, Bubb MR, Hanley Jr EN, Gruber HE. Exogenous thymosin beta4 prevents apoptosis in human intervertebral annulus cells in vitro. Biotech Histochem 2009;84:287–94
- Sudo H, Yamada K, Iwasaki K, Higashi H, Ito M, Minami A, Iwasaki N. Global identification of genes related to nutrient deficiency in intervertebral disc cells in an experimental nutrient deprivation model. PLoS One 2013;8:e58806
- Sudo H, Minami A. Regulation of apoptosis in nucleus pulposus cells by optimized exogenous Bcl-2 overexpression. J Orthop Res 2010;28:1608–13
- Csibi A, Fendt SM, Li C, Poulogiannis G, Choo AY, Chapski DJ, Jeong SM, Dempsey JM, Parkhitko A, Morrison T, Henske EP, Haigis MC, Cantley LC, Stephanopoulos G, Yu J, Blenis J. The mTORC1 pathway stimulates glutamine metabolism and cell proliferation by repressing SIRT4. Cell 2013;153:840–54
- Srinivas V, Bohensky J, Shapiro IM. Autophagy: a new phase in the maturation of growth plate chondrocytes is regulated by HIF, mTOR and AMP kinase. Cells Tissues Organs 2009;189:88–92
- Morselli E, Maiuri MC, Markaki M, Megalou E, Pasparaki A, Palikaras K, Criollo A, Galluzzi L, Malik SA, Vitale I, Michaud M, Madeo F, Tavernarakis N, Kroemer G. Caloric restriction and resveratrol promote longevity through the Sirtuin-1-dependent induction of autophagy. Cell Death Dis 2010;1:e10
- Ng F, Tang BL. Sirtuins' modulation of autophagy. J Cell Physiol 2013;228:2262–70
- Ye W, Zhu W, Xu K, Liang A, Peng Y, Huang D, Li C. Increased macroautophagy in the pathological process of intervertebral disc degeneration in rats. Connect Tissue Res 2012;54:22–8
- Ye W, Xu K, Huang D, Liang A, Peng Y, Zhu W, Li C. Age-related increases of macroautophagy and chaperone-mediated autophagy in rat nucleus pulposus. Connect Tissue Res 2011;52:472–8
- Jiang L, Zhang X, Xu H. Protective effect of autophagy on nucleus pulposus cells under starvation. Chin J Pathophysiol 2012;28:1302–7
- Suzuki SW, Onodera J, Ohsumi Y. Starvation induced cell death in autophagy-defective yeast mutants is caused by mitochondria dysfunction. PLoS One 2011;6:e17412
- Gray ML, Pizzanelli AM, Grodzinsky AJ, Lee RC. Mechanical and physiochemical determinants of the chondrocyte biosynthetic response. J Orthop Res 1988;6:777–92
- Grodzinsky AJ. Electromechanical and physicochemical properties of connective tissue. Crit Rev Biomed Eng 1983;9:133–99
- Ichimura K, Tsuji H, Matsui H, Makiyama N. Cell culture of the intervertebral disc of rats: factors influencing culture, proteoglycan, collagen, and deoxyribonucleic acid synthesis. J Spinal Disorders 1991;4:428–36
- Kitano T, Zerwekh JE, Usui Y, Edwards ML, Flicker PL, Mooney V. Biochemical changes associated with the symptomatic human intervertebral disk. Clin Orthop Rel Res 1993;293:372–7
- Diamant B, Karlsson J, Nachemson A. Correlation between lactate levels and pH in discs of patients with lumbar rhizopathies. Experientia 1968;24:1195–6
- Yuan FL, Wang HR, Yuan W, Zhao MD, Cao L, Duan PG, Jiang YQ, Li XL, Dong J. Ovarian cancer G protein-coupled receptor 1 is involved in acid-induced apoptosis of endplate chondrocytes in intervertebral discs. J Bone Miner Res 2014;29:67–77
- Rong C, Chen FH, Jiang S, Hu W, Wu FR, Chen TY, Yuan FL. Inhibition of acid-sensing ion channels by amiloride protects rat articular chondrocytes from acid-induced apoptosis via a mitochondrial-mediated pathway. Cell Biol Int 2012;36:635–41
- Razaq S, Wilkins RJ, Urban JP. The effect of extracellular pH on matrix turnover by cells of the bovine nucleus pulposus. Eur Spine J 2003;12:341–9
- Ohshima H, Urban JP. The effect of lactate and pH on proteoglycan and protein synthesis rates in the intervertebral disc. Spine (Phila Pa 1976) 1992;17:1079–82
- Neidlinger-Wilke C, Mietsch A, Rinkler C, Wilke HJ, Ignatius A, Urban J. Interactions of environmental conditions and mechanical loads have influence on matrix turnover by nucleus pulposus cells. J Orthop Res 2012;30:112–21
- Li X, Xu RS, Jiang DL, He XL, Jin C, Lu WG, Su Q, Yuan FL. Acid-sensing ion channel 1a is involved in acid-induced osteoclastogenesis by regulating activation of the transcription factor NFATc1. FEBS Lett 2013;587:3236–42
- Li X, Wu FR, Xu RS, Hu W, Jiang DL, Ji C, Chen FH, Yuan FL. Acid-sensing ion channel 1a-mediated calcium influx regulates apoptosis of endplate chondrocytes in intervertebral discs. Expert Opin Ther Targets 2014;18:1–14
- Hu W, Chen FH, Yuan FL, Zhang TY, Wu FR, Rong C, Jiang S, Tang J, Zhang CC, Lin MY. Blockade of acid-sensing ion channels protects articular chondrocytes from acid-induced apoptotic injury. Inflamm Res 2012;61:327–35
- Uchiyama Y, Cheng CC, Danielson KG, Mochida J, Albert TJ, Shapiro IM, Risbud MV. Expression of acid-sensing ion channel 3 (ASIC3) in nucleus pulposus cells of the intervertebral disc is regulated by p75NTR and ERK signaling. J Bone Miner Res 2007;22:1996–2006
- Uchiyama Y, Guttapalli A, Gajghate S, Mochida J, Shapiro IM, Risbud MV. SMAD3 functions as a transcriptional repressor of acid-sensing ion channel 3 (ASIC3) in nucleus pulposus cells of the intervertebral disc. J Bone Mineral Res 2008;23:1619–28
- Colombo E, Francisconi S, Faravelli L, Izzo E, Pevarello P. Ion channel blockers for the treatment of neuropathic pain. Future Med Chem 2010;2:803–42
- Jeong S, Lee SH, Kim YO, Yoon MH. Antinociceptive effects of amiloride and benzamil in neuropathic pain model rats. J Korean Med Sci 2013;28:1238–43
- Marino ML, Pellegrini P, Di Lernia G, Djavaheri-Mergny M, Brnjic S, Zhang X, Hagg M, Linder S, Fais S, Codogno P, De Mlito A. Autophagy is a protective mechanism for human melanoma cells under acidic stress. J Biol Chem 2012;287:30664–76
- Wojtkowiak JW, Gillies RJ. Autophagy on acid. Autophagy 2012;8:1688–9
- Wojtkowiak JW, Rothberg JM, Kumar V, Schramm KJ, Haller E, Proemsey JB, Lloyd MC, Sloane BF, Gillies RJ. Chronic autophagy is a cellular adaptation to tumor acidic pH microenvironments. Cancer Res 2012;72:3938–47
- Hanada T, Noda NN, Satomi Y, Ichimura Y, Fujioka Y, Takao T, Inagaki F, Ohsumi Y. The Atg12-Atg5 conjugate has a novel E3-like activity for protein lipidation in autophagy. J Biol Chem 2007;282:37298–302
- Balgi AD, Diering GH, Donohue E, Lam KK, Fonseca BD, Zimmerman C, Numata M, Roberge M. Regulation of mTORC1 signaling by pH. PLoS One 2011;6:e21549
- Wu W, Zhang X, Hu X, Wang X, Sun L, Zheng X, Jiang L, Ni X, Xu C, Tian N, Zhu S, Xu H. Lactate down-regulates matrix systhesis and promotes apoptosis and autophagy in rat nucleus pulposus cells. J Orthop Res 2014;32:253–61
- Sakellaridis N, Androulis A. Influence of diabetes mellitus on cervical intervertebral disc herniation. Clin Neurol Neurosurg 2008;110:810–12
- Sakellaridis N. The influence of diabetes mellitus on lumbar intervertebral disk herniation. Surg Neurol 2006;66:152–4
- Freedman MK, Hilibrand AS, Blood EA, Zhao W, Albert TJ, Vaccaro AR, Oleson CV, Morgan TS, Weinstein JN. The impact of diabetes on the outcomes of surgical and nonsurgical treatment of patients in the spine patient outcomes research trial. Spine (Phila Pa 1976) 2011;36:290–307
- Jiang L, Zhang X, Zheng X, Ru A, Ni X, Wu Y, Tian N, Huang Y, Xue E, Wang X, Xu H. Apoptosis, senescence, and autophagy in rat nucleus pulposus cells: Implications for diabetic intervertebral disc degeneration. J Orthop Res 2013;31:692–702
- Won HY, Park JB, Park EY, Riew KD. Effect of hyperglycemia on apoptosis of notochordal cells and intervertebral disc degeneration in diabetic rats. J Neurosurg Spine 2009;11:741–8
- Illien-Junger S, Grosjean F, Laudier DM, Vlassara H, Striker GE, Iatridis JC. Combined anti-inflammatory and anti-age drug treatments have a protective effect on intervertebral discs in mice with diabetes. PLoS One 2013;8:e64302
- Park EY, Park JB. Dose- and time-dependent effect of high glucose concentration on viability of notochordal cells and expression of matrix degrading and fibrotic enzymes. Int Orthop 2013;37:1179–86
- Zhao CQ, Zhang YH, Jiang SD, Jiang LS, Dai LY. Both endoplasmic reticulum and mitochondria are involved in disc cell apoptosis and intervertebral disc degeneration in rats. Age (Dordr) 2010;32:161–77
- Wang H, Liu H, Zheng ZM, Zhang KB, Wang TP, Sribastav SS, Liu WS, Liu T. Role of death receptor, mitochondrial and endoplasmic reticulum pathways in different stages of degenerative human lumbar disc. Apoptosis 2011;16:990–1003
- Tsai TT, Ho NY, Lin YT, Lai PL, Fu TS, Niu CC, Chen LH, Chen WJ, Pang JH. Advanced glycation end products in degenerative nucleus pulposus with diabetes. J Orthop Res 2014;32:238–44
- Yang L, Zhu L, Dong W, Cao Y, Lin L, Rong Z, Zhang Z, Wu G. Reactive oxygen species-mediated mitochondrial dysfunction plays a critical role in high glucose-induced nucleus pulposus cell injury. Int Orthop 2013;38:205–6
- Park EY, Park JB. High glucose-induced oxidative stress promotes autophagy through mitochondrial damage in rat notochordal cells. Int Orthop 2013;37:2507–14
- Yang L, Zhu L, Cao Y, Wang K. Whether reactive oxygen species plays a critical role in intrinsic (mitochondrial) pathway-mediated apoptosis in notochordal cells under high glucose concentrations? Int Orthop 2013;37:1649–50
- Park EY, Park JB. High glucose-induced oxidative stress promotes autophagy through mitochondrial damage in rat notochordal cells. Int Orthop 2013;37:2507–14
- Wang Y, Li YB, Yin JJ, Wang Y, Zhu LB, Xie GY, Pan SH. Autophagy regulates inflammation following oxidative injury in diabetes. Autophagy 2013;9:272–7
- Ryter SW, Choi AM. Regulation of autophagy in oxygen-dependent cellular stress. Curr Pharmaceut Design 2013;19:2747–56
- Chen ZF, Li YB, Han JY, Wang J, Yin JJ, Li JB, Tian H. The double-edged effect of autophagy in pancreatic beta cells and diabetes. Autophagy 2011;7:12–16
- Sasaki H, Takayama K, Matsushita T, Ishida K, Kubo S, Matsumoto T, Fujita N, Oka S, Kurosaka M, Kuroda R. Autophagy modulates osteoarthritis-related gene expressions in human chondrocytes. Arthritis Rheum 2011;64:1920–8
- Urban JP. The role of the physicochemical environment in determining disc cell behaviour. Biochem Soc Trans 2002;30:858–64
- Bartels EM, Fairbank JC, Winlove CP, Urban JP. Oxygen and lactate concentrations measured in vivo in the intervertebral discs of patients with scoliosis and back pain. Spine (Phila Pa 1976) 1998;23:1–7; discussion 8
- Feng G, Li L, Liu H, Song Y, Huang F, Tu C, Shen B, Gong Q, Li T, Liu L, Zeng J, Kong Q, Yi M, Gupte M, Ma PX, Pei F. Hypoxia differentially regulates human nucleus pulposus and annulus fibrosus cell extracellular matrix production in 3D scaffolds. Osteoarthritis Cartilage 2013;21:582–8
- Li H, Liang CZ, Chen QX. Regulatory role of hypoxia inducible factor in the biological behavior of nucleus pulposus cells. Yonsei Med J 2013;54:807–12
- Risbud MV, Schipani E, Shapiro IM. Hypoxic regulation of nucleus pulposus cell survival: from niche to notch. Am J Pathol 2010;176:1577–83
- Rajpurohit R, Risbud MV, Ducheyne P, Vresilovic EJ, Shapiro IM. Phenotypic characteristics of the nucleus pulposus: expression of hypoxia inducing factor-1, glucose transporter-1 and MMP-2. Cell Tissue Res 2002;308:401–7
- Agrawal A, Gajghate S, Smith H, Anderson DG, Albert TJ, Shapiro IM, Risbud MV. Cited2 modulates hypoxia-inducible factor-dependent expression of vascular endothelial growth factor in nucleus pulposus cells of the rat intervertebral disc. Arthritis Rheum 2008;58:3798–808
- Zeng Y, Danielson KG, Albert TJ, Shapiro IM, Risbud MV. HIF-1 alpha is a regulator of galectin-3 expression in the intervertebral disc. J Bone Miner Res 2007;22:1851–61
- Fujita N, Imai J, Suzuki T, Yamada M, Ninomiya K, Miyamoto K, Iwasaki R, Morioka H, Matsumoto M, Chiba K, Watanabe S, Suda T, Toyama Y, Miyamoto T. Vascular endothelial growth factor-A is a survival factor for nucleus pulposus cells in the intervertebral disc. Biochem Biophys Res Commun 2008;372:367–72
- Agrawal A, Guttapalli A, Narayan S, Albert TJ, Shapiro IM, Risbud MV. Normoxic stabilization of HIF-1alpha drives glycolytic metabolism and regulates aggrecan gene expression in nucleus pulposus cells of the rat intervertebral disk. Am J Physiol Cell Physiol 2007;293:C621–31
- Bohensky J, Leshinsky S, Srinivas V, Shapiro IM. Chondrocyte autophagy is stimulated by HIF-1 dependent AMPK activation and mTOR suppression. Pediatr Nephrol 2010;25:633–42
- Bohensky J, Terkhorn SP, Freeman TA, Adams CS, Garcia JA, Shapiro IM, Srinivas V. Regulation of autophagy in human and murine cartilage: hypoxia-inducible factor 2 suppresses chondrocyte autophagy. Arthritis Rheum 2009;60:1406–15
- Adams MA, Roughley PJ. What is intervertebral disc degeneration, and what causes it? Spine (Phila Pa 1976) 2006;31:2151–61
- Kuo YJ, Wu LC, Sun JS, Chen MH, Sun MG, Tsuang YH. Mechanical stress-induced apoptosis of nucleus pulposus cells: an in vitro and in vivo rat model. J Orthop Sci 2014;19:313–22
- Ding F, Shao ZW, Yang SH, Wu Q, Gao F, Xiong LM. Role of mitochondrial pathway in compression-induced apoptosis of nucleus pulposus cells. Apoptosis 2012;17:579–90
- Chan SC, Walser J, Kappeli P, Shamsollahi MJ, Ferguson SJ, Gantenbein-Ritter B. Region specific response of intervertebral disc cells to complex dynamic loading: an organ culture study using a dynamic torsion-compression bioreactor. PLoS One 2013;8:e72489
- Zhu Q, Jackson AR, Gu WY. Cell viability in intervertebral disc under various nutritional and dynamic loading conditions: 3d finite element analysis. J Biomech 2012;45:2769–77
- Zhang YH, Zhao CQ, Jiang LS, Dai LY. Cyclic stretch-induced apoptosis in rat annulus fibrosus cells is mediated in part by endoplasmic reticulum stress through nitric oxide production. Eur Spine J 2011;20:1233–43
- Xie M, Yang S, Win HL, Xiong L, Huang J, Zhou J. Rabbit annulus fibrosus cell apoptosis induced by mechanical overload via a mitochondrial apoptotic pathway. J Huazhong Univ Sci Technol Med Sci 2010;30:379–84
- Hirata H, Yurube T, Kakutani K, Maeno K, Takada T, Yamamoto J, Kurakawa T, Akisue T, Kuroda R, Kurosaka M, Nishida K. A rat tail temporary static compression model reproduces different stages of intervertebral disc degeneration with decreased notochordal cell phenotype. J Orthop Res 2014;32:455–63
- Wang C, Gonzales S, Levene H, Gu W, Huang CY. Energy metabolism of intervertebral disc under mechanical loading. J Orthop Res 2013;31:1733–8
- Huang M, Wang HQ, Zhang Q, Yan XD, Hao M, Luo ZJ. Alterations of ADAMTSs and TIMP-3 in human nucleus pulposus cells subjected to compressive load: implications in the pathogenesis of human intervertebral disc degeneration. J Orthop Res 2012;30:267–73
- Ma KG, Shao ZW, Yang SH, Wang J, Wang BC, Xiong LM, Wu Q, Chen SF. Autophagy is activated in compression-induced cell degeneration and is mediated by reactive oxygen species in nucleus pulposus cells exposed to compression. Osteoarthritis Cartilage 2013;21:2030–8
- Huang CY, Yuan TY, Jackson AR, Hazbun L, Fraker C, Gu WY. Effects of low glucose concentrations on oxygen consumption rates of intervertebral disc cells. Spine (Phila Pa 1976) 2007;32:2063–9
- Bibby SR, Jones DA, Ripley RM, Urban JP. Metabolism of the intervertebral disc: effects of low levels of oxygen, glucose, and pH on rates of energy metabolism of bovine nucleus pulposus cells. Spine (Phila Pa 1976) 2005;30:487–96
- Malandrino A, Noailly J, Lacroix D. The effect of sustained compression on oxygen metabolic transport in the intervertebral disc decreases with degenerative changes. PLoS Comput Biol 2011;7:e1002112
- Roberts S, Evans EH, Kletsas D, Jaffray DC, Eisenstein SM. Senescence in human intervertebral discs. Eur Spine J 2006;15:S312–16
- Yang Q, Zhao YH, Xia Q, Xu BS, Ma XL, Liu Y, Hu YC, Li HF, Miao J, Wang T, Ma JX, Sun XL. Novel cartilage-derived biomimetic scaffold for human nucleus pulposus regeneration: a promising therapeutic strategy for symptomatic degenerative disc diseases. Orthop Surg 2013;5:60–3